Intermittent Mandatory Ventilation: Introduction
Intermittent mandatory ventilation (IMV) allows the patient to breathe spontaneously between machine-cycled or mandatory breaths. This concept originated in 1955 with an unnamed ventilator designed by Engstrom.1,2 In the early 1970s, Kirby et al3,4 introduced IMV as a means of ventilator support of infants with respiratory distress syndrome. In 1973, Downs et al5 were the first to propose IMV as a method to facilitate discontinuation from mechanical ventilation in adults. Those investigators6,7 also pioneered IMV use as a primary means of ventilator support during acute respiratory failure. Subsequently, breath-delivery design has been modified. Mandatory breaths initially delivered regardless of respiratory timing are synchronized with the patient’s inspiratory effort.8,9 This mode of ventilation has been termed intermittent demand ventilation, 8 intermittent assisted ventilation,9 and synchronous intermittent mandatory ventilation (SIMV). SIMV is an established partial mechanical ventilation mode in critically ill patients, both adult10 and neonate, worldwide.11 Currently, however, SIMV application in adults has declined except in North America12 and Australia–New Zealand,13 whereas in neonates, SIMV application remains prevalent.14 This chapter uses the terms IMV and SIMV interchangeably unless specifically indicated for clarification.
Basic Principles
IMV is a means of ventilator support in which a preset number of positive-pressure (mandatory) breaths are delivered while the patient breathes spontaneously between the mandatory breaths. The mandatory breaths can be in the form of a preset volume (flow-limited, volume-cycled), pressure (pressure-limited, time-cycled),15 or a combination of pressure and volume (dual control).16 In principle, IMV is similar to controlled mechanical ventilation (CMV), in which the patient receives a predetermined number of mandatory machine-triggered breaths independent of spontaneous breathing effort. Likewise, SIMV is similar to assist-control ventilation (ACV), in which mandatory breaths are triggered by the patient. In contrast to CMV and ACV, however, in both IMV and SIMV the patient is allowed to breathe spontaneously between the mandatory breaths. In addition, with IMV and SIMV, the clinician can vary the ventilator support level according to the set IMV rate. At a high IMV rate, in which the patient’s spontaneous effort is suppressed, IMV provides full ventilator support. At a zero IMV rate, it provides no support, and all breaths are spontaneous. Between these extremes, IMV provides partial ventilator support.
Three types of IMV systems are described: continuous-flow IMV and pressure-triggered and flow-triggered SIMV systems.
The original IMV design uses a continuous-flow system.3 Two parallel circuits—one for the patient’s spontaneous breaths and the other for the mechanical breaths—are connected through a sidearm and a one-way valve, and share a common oxygen and air source. The continuous-flow IMV setup can be either an open or a closed system.17 The open system employs a reservoir tube that has a capacity of at least 1.5 times the patient’s tidal volume (VT) and is open to the atmosphere (Fig. 7-1). For this reason, continuous positive airway pressure (CPAP) cannot be applied during the spontaneous breathing cycles. To reduce inspiratory resistance, the side port of the spontaneous breathing circuit is placed between the patient Y and the humidifier. The continuous flow of fresh gas is humidified using a venturi nebulizer. The reservoir tubing’s considerable length and the inability to maintain a CPAP level make this open system cumbersome.
Figure 7-1
Continuous-flow intermittent mandatory ventilation setup with a reservoir tube. The sidearm of the spontaneous breathing circuit is connected through a one-way valve to the inspiratory limb of the ventilator circuit. The sidearm is placed between the humidifier and the patient Y. See text for further explanation. PEEP, positive end-expiratory pressure.
The closed system employs a reservoir bag (Fig. 7-2) that minimizes airway pressure fluctuations because the inspired gas flow rate may be limited by the maximum flow generated by the hospital’s compressed air and oxygen source. In addition, constant positive airway pressure can be maintained during both the mandatory (i.e., positive end-expiratory airway pressure [PEEP]) and spontaneous breaths (i.e., CPAP). During spontaneous breathing, the patient breathes from the reservoir bag via the one-way valve. When the ventilator cycles, the one-way valve closes, and a positive-pressure breath is delivered to the patient. During the mechanical breath, excess gas in the reservoir bag is vented through a relief valve. Exhalation occurs through the ventilator’s exhalation circuit, which is supplied with a PEEP valve.
Figure 7-2
Continuous-flow intermittent mandatory ventilation setup with a reservoir bag. The sidearm for the spontaneous breathing circuit is connected through a one-way valve to the inspiratory limb of the ventilator circuit. The sidearm is placed proximal to the humidifier. See text for further explanation. PEEP, positive end-expiratory pressure.
In a continuous-flow IMV system, the inspired gas flow rate within the spontaneous breathing circuit must exceed the patient’s peak inspiratory flow rate to minimize airway pressure fluctuations and hence the patient’s inspiratory work. When set appropriately, spontaneous breathing in continuous-flow IMV should resemble breathing from the atmosphere.
Continuous-flow IMV has several disadvantages.18–23 In addition to gas wastage, inaccurate VT measurement and extra circuitry requirement, patient–ventilator asynchrony potentially can occur because mandatory breaths are not delivered in concert with the patient’s inspiratory effort (Fig. 7-3A). Whereas asynchrony has no significant effect in adults,24 in neonates, particularly preterm infants,25 asynchrony associated with continuous-flow IMV resulted in large fluctuations of VT26 and lower partial pressure of arterial oxygen (PaO2)27,28 than with SIMV. The effect of IMV on PaO2 was confirmed in a large randomized multicenter trial.29 The degree and duration of hypoxia appear to result from active exhalation,30 while muscle relaxant improves oxygenation.31,32 Furthermore, the application of SIMV is superior to IMV in terms of reduced duration of mechanical ventilation, requirement for reintubation, incidence of intraventricular hemorrhage, and bronchopulmonary dysplasia.33 In term infants and children (ages 1 month to 4 years), however, IMV or SIMV plus pressure support has similar effects on duration of mechanical ventilation, weaning, and intensive care unit length of stay.34
Figure 7-3
A. Continuous-flow intermittent mandatory ventilation (IMV). Airway pressure (Paw) and flow tracings (). The vertical dashed line indicates the onset of inspiratory flow. Minimal fluctuations of Paw are a result of circuit resistance. Large fluctuations of Paw occur when inspiratory flow rates are insufficient and a reservoir bag is not used. exp, Expiration; insp, inspiration; PEEP, positive end-expiratory airway pressure. B. Pressure-triggered synchronous intermittent mandatory ventilation (SIMV). A period of zero flow before the onset of flow (indicated by the vertical dashed line) can be detected on the flow tracing. Zero flow coincides with patient triggering to open the proportional valve. During the unassisted breathing after flow onset, Paw continues to drop during inspiration because of inadequate flow delivery. C. Flow-triggered synchronous intermittent mandatory ventilation (SIMV). Triggering phase is significantly shorter than with pressure-triggered SIMV. During the unassisted breathing following patient triggering, Paw is maintained at or slightly above the PEEP level, suggesting adequate flow delivery during inspiration.
A technologically advanced continuous-flow IMV or CPAP is the flow-regulated IMV or CPAP.35 Flow within the circuit is polled, for example, every 20 minutes and used as a feedback signal to increase the basal flow to match the patient’s ventilatory demand during inspiration and subtract it during exhalation. Flow-regulated IMV or CPAP eliminates airway fluctuations and decreases imposed work during both inspiration and exhalation. Using a mechanical lung model with flow-regulated CPAP of 5 cm H2O, the total imposed work was 3.4 mJ/breath versus 43.5 mJ/breath with a continuous-flow CPAP device and an added 20-L reservoir bag.35
DiBlasi et al36 described a modified continuous-flow IMV, a combination of continuous-flow IMV and low-to-moderate frequency oscillatory ventilation. It is also called Bubble IMV (B-IMV), intended as an inexpensive means of ventilator support in infants. The device is equipped with a microprocessor-controlled rate, inspiratory timing, and pinch valve. The pinch valve regulates the path of gas exiting the system into a water-seal chamber (Fig. 7-4). The inhalation path controls the peak inspiratory pressure level of the mandatory breaths, and the exhalation path allows spontaneous breaths (i.e., CPAP), and control PEEP level. The exhalation path outlet is connected to an adjustable bubbler with three configurations: a bubbler angle of 0 degrees, 90 degrees, and 135 degrees. The bubbler angle determines the oscillation amplitudes in airway pressure (Paw). Only the bubbler angle of 135 degrees is associated with the largest pressure oscillations at a frequency of 2 to 5 Hz, and, hence, the largest change in volume (Fig. 7-5A).37 In paralyzed juvenile rabbits with saline lavage-induced lung injury, B-IMV provided comparable peak inspiratory and mean Paw, PEEP, and PaO2 as with CMV, irrespective of the bubbler angle (Fig. 7-5B).36 B-IMV135, however, was associated with consistently low mean partial pressure of arterial carbon dioxide (PaCO2; 35 mm Hg). Mean PaCO2 with B-IMV90, B-IMV0, and CMV was 45, 45, and 55 mm Hg, respectively. The low PaCO2 with B-IMV135 is caused by additional volume produced by the oscillatory pressures (DiBlasi, personal communication) (Fig. 7-5A). At this time, B-IMV is an experimental ventilator, its potential application in preterm infants with respiratory distress syndrome and its efficacy when compared with SIMV remains to be determined.
Figure 7-4
Bubble-intermittent mandatory ventilation (B-IMV). A modified continuous-flow IMV is equipped with a rotameter to control bias flows of air and oxygen. In line of patient’s circuit are a high-pressure relief valve set to a limit of 40 cm H2O, oxygen analyzer, humidifier, airway pressure monitor, a demand flow valve that allows room air to enter the circuit if the bias flow does not meet the patient’s flow demand, and a pinch valve with a rate and inspiratory time controller. The pinch valve controls the path of gas exiting the system, either through the inhalation path with the tube placed deep in the water-seal chamber to control peak inspiratory pressure level of the mandatory breaths, or through the exhalation path to allow spontaneous breaths (continuous positive airway pressure [CPAP]) and control positive end-expiratory airway pressure (PEEP) level. The exhalation path outlet is connected to a bubbler with the angles adjustable to 0 degrees, 90 degrees, and 135 degrees (B-IMV0, B-IMV90 and B-IMV135, respectively). (Adapted, with permission, from DiBlasi RM, Zignego JC, Smith CV, et al. Effective gas exchange in paralyzed juvenile rabbits using simple, inexpensive respiratory support devices. Pediatr Res. 2010;68:526–530.)
Figure 7-5
A. Effects of bubbler angle configuration on airway pressure (Paw) oscillations. Bubble-continuous positive airway pressure connected to a test lung with a bias flow set at 8 L/min. Bubbler angle of 135 degrees produces the largest airway pressure oscillations at a frequency of 2 to 5 Hz, and largest change in volume. B. Superimposed airway pressure waveform during CMV (solid black) and bubble-intermittent mandatory ventilation with bubble angle of 135 degrees (B-IMV135) (double gray line) in an animal with lung injury. Horizontal dashed line represents mean airway pressure. (Adapted, with permission, from DiBlasi RM, Zignego JC, Tang DM, et al. Noninvasive respiratory support of juvenile rabbits by high-amplitude bubble continuous positive airway pressure. Pediatr Res. 2010;67:624–629.)
SIMV is currently the standard for clinical use.10,11 SIMV incorporates a demand valve that is triggered by the patient with each spontaneous breath and delivers a mandatory breath in concert with the patient’s inspiratory effort. If the patient ceases to trigger the ventilator, mandatory breaths will be triggered by the machine and delivered according to the preset rate. The demand valve can be triggered by either a fall in pressure (pressure-triggered) or a change in flow (flow-triggered). In pressure-triggered SIMV, a preset pressure sensitivity must be achieved before the ventilator delivers fresh gas into the inspiratory circuit.38,39 A noticeable delay in opening the demand valve occurs between onset of inspiratory effort and flow delivery (see Fig. 7-3B). Flow-triggered SIMV uses a preset flow sensitivity as the trigger mechanism.38,39
The pressure-triggering and flow-triggering characteristics of the spontaneous breaths (CPAP) are an important component of the imposed work of a SIMV system (see Chapter 3).40,41 This situation arises because there is little adaptation to the mandatory breaths’ ventilatory assistance unless the system is set at a substantial assistance level.42 Fortunately, most modern microprocessor-based ventilators employ a remarkably responsive proportional solenoid valve such that the work imposed during the trigger phase (the interval from onset of patient effort to valve opening or flow delivery) is a small percentage of the total inspiratory work of breathing (<10% with pressure-triggered SIMV).43 Nevertheless, flow triggering has become the preferred triggering method not only for SIMV but also for various other ventilation modes because of its faster response time and therefore shorter triggering phase compared with pressure triggering (see Fig. 7-3C).44 In the posttrigger phase (the interval from onset of flow delivery to the end of inspiration), flow delivery with pressure triggering may not be adequate during the spontaneous breaths.38 The feedback signal for flow delivery is the gradient between a manufacturer-determined target pressure and circuit pressure. As the pressure gradient increases, flow delivery increases. This pressure gradient can be enhanced by adding pressure support or sensing the circuit pressure at the distal end of the endotracheal tube.45,46 From a practical standpoint, adding pressure support is preferable. Alternatively, flow delivery during the spontaneous breaths may be augmented by using changes in flow instead of pressure as a feedback signal for adding and subtracting flow from the base flow during inspiration and exhalation, respectively.35
The mandatory breaths can be in the form of volume-limited or pressure-limited. In preterm infants, pressure-targeted ventilation is prevalent,14 with similar efficacy as with volume-targeted ventilation in maintaining oxygenation.47 Augmenting flow delivery during the mandatory breaths can be accomplished by setting the pressure-attack rate sufficiently high when pressure-limited mandatory breaths are employed.48,49 To maintain a constant VT with pressure-limited mandatory breaths, both pressure-limited and volume-limited breaths can be combined in the form of dual control within breaths50 or breath-to-breath ventilation (see Chapter 15).51 Several microprocessor-based ventilators are equipped with dual-control breath-to-breath ventilation that can be applied in the SIMV mode.52 In essence, this form of mandatory breath is a pressure-limited, time-cycled breath that uses VT as feedback control for continuously adjusting the pressure limit to attain the set VT. The volume signal used as feedback to the ventilator controller is the volume exiting the ventilator and not the exhaled VT . This step prevents runaway of airway pressure that could occur if a leak in the circuit caused inaccurate measurement of exhaled volume. As an additional safety feature, if the volume exiting the ventilator exceeds 150% of the set VT, then the ventilator exhalation valve opens, ending the mechanical inspiration. With SIMV, most microprocessor-based ventilators are able to apply pressure support and dual-control breathing to the spontaneous and mandatory breaths, respectively, to improve flow delivery and maintain a set VT during pressure-limited mandatory breaths. In preterm infants, because changes in respiratory system mechanics occur frequently, the set VT of the mandatory dual-control breaths not only provides a guaranteed volume when respiratory system compliance decreases but also prevents overinflation when compliance improves.53,54 In fact, a recent report demonstrates a decrease in mortality and chronic lung disease with volume-targeted compared with pressure-targeted ventilation.55
Physiologic Effects
The physiologic effects of IMV not listed in this section are discussed in comparison with other ventilation modes.
In early IMV development, it was thought that inspiratory muscle activity is downregulated during the mandatory breaths and that IMV allows a combination of unassisted breathing with respiratory muscle rest to promote weaning.5,56 By adjusting mandatory breath frequency, inspiratory effort could be modified until the patient resumed complete control of spontaneous breathing. Several studies, however, disproved this hypothesis.42,57–60 Imsand et al57 studied the neuromuscular output of patients recovering from acute exacerbation of chronic obstructive pulmonary disease who were receiving pressure-triggered SIMV. The mandatory-breath VT was set at 10 to 12 mL/kg. Neuromuscular output was estimated from the amplitude of the integrated diaphragmatic electrical activity (Edi), measured using bipolar esophageal electrodes. Sternocleidomastoid muscle electrical activity was recorded using surface electrodes. Neuromuscular output, occlusion esophageal pressure (Pes0.1, another index of neuromuscular output), and neural inspiratory time (TI) were measured at three ventilator support levels: >60%, 50% to 20%, and 0% of total support. Total support was defined as the support at which Edi was suppressed completely. Only at the highest machine assistance rate did Edi decrease significantly, whereas sternocleidomastoid muscle electrical activity did not (Fig. 7-6). Moreover, across all levels of ventilator support, both Edi (Fig. 7-7) and Pes0.1 of the unassisted and machine breaths were similar. Pes0.1, however, tended to increase with decreasing machine assistance. Likewise, neural TI, defined as the interval from onset to peak Edi, was equivalent for unassisted and machine breaths.
Figure 7-6
Peak inspiratory amplitude of integrated electrical activity of the diaphragm (Edi) and sternocleidomastoid muscles (EMGscm) at three levels of machine assistance during SIMV, expressed as a percentage of mean value of 0% of or minimal (4 breaths/min) machine assistance. Values are mean ± standard error. *P < 0.01 compared with 0% of machine assistance. † P <0.05 compared with spontaneous cycles at 50% to 20% of assistance. Assist, Machine-assisted breaths; spont, intervening spontaneous breaths. (Adapted, with permission, from Imsand C, Feihl F, Perret C, et al. Regulation of inspiratory neuromuscular output during synchronized intermittent mechanical ventilation. Anesthesiology. 1994;80:13–22.)
Figure 7-7
Electrical activity of the diaphragm (Edi) and sternocleidomastoid muscles (EMGscm) in a patient receiving SIMV. Intensity and duration of electrical activity in successive assisted (A) and intervening spontaneous (S) breaths are similar. The regular spikes in the Edi tracing are the QRS complex of the electrocardiogram signal. Paw, Airway pressure; Pes, esophageal pressure; red lines and green lines, inspiratory phase of the assisted and spontaneous breaths, respectively; yellow lines, expiratory phase. (Adapted, with permission, from Imsand C, Feihl F, Perret C, et al. Regulation of inspiratory neuromuscular output during synchronized intermittent mechanical ventilation. Anesthesiology. 1994;80:13–22.)
In another study, Uchiyama et al58 applied continuous-flow IMV to anesthetized rabbits and measured Edi with implanted electrodes into the diaphragm. The IMV rate was set at 0, 5, 10, 15, and 20 breaths/min, at which Edi was suppressed completely. Edi was expressed as percent of Edi at zero IMV rate per minute. Compared with spontaneous breathing, Edi decreased significantly only at an IMV rate of 15 breaths/min (75% of total support). In contrast with the study of Imsand et al,57 at an IMV rate of 10 and 15 breaths/min, Edi of the mandatory breaths was absent. The difference in neuromuscular output response during mandatory breaths in the study of Uchiyama et al58 may be related to the effect of anesthesia and the large VT applied (15 mL/kg), causing vagally mediated inspiratory inhibition.59 It appears that in anesthetized animals at ventilator support of 50% or greater, breath-by-breath adaptation to ventilator assistance occurred, but it was not observed in conscious humans.
In unsedated preterm infants with acute respiratory failure receiving flow-triggered SIMV, Beck et al60 measured Edi and neural timing of mandatory breaths and the unassisted breaths immediately preceding and following the mandatory breaths using miniaturized electrodes mounted on a feeding tube. The set SIMV rate ranged from 5 to 25 breaths/min. Both Edi and neural TI amplitude were similar for the mandatory and unassisted breaths (Fig. 7-8). In contrast to improved triggering in adults,57 during the mandatory breaths, a substantial delay was observed from onset of Edi to flow delivery, an average of 95 milliseconds (range: 5 to 110 milliseconds). Because most of the infants had bronchiolitis and likely were hyperinflated, the authors attributed the trigger delay or inspiratory asynchrony to neuroventilatory uncoupling (a delay from onset of diaphragmatic activation to flow generation)61 rather than to the ventilator triggering system. Neural expiratory time (TE) of the mandatory breath, defined as the interval from peak to Edi onset of the subsequent breath, was prolonged. The relative increase in neural TE during the mandatory breaths was related to the time the ventilator continued to inflate beyond the end of neural TI (R2 = 0.66; P = 0.01). This extended time was consistent with a vagally mediated reflex when pulse inflation was delivered into early expiration.62,63 Both inspiratory asynchrony and expiratory asynchrony were present during every mandatory breath and constituted, on average, 53% of the total breath duration (Fig. 7-9). Expiratory asynchrony may result in increased peak airway pressure as the subject attempts to terminate inspiration by recruiting the expiratory muscles.64 It also may result in increased work of breathing and discomfort and manifest as a patient “fighting the ventilator,”65 potentially dictating sedation.
Figure 7-8
Diaphragmatic electrical activity (Edi) time profile for group mean data. No significant difference exists between peak Edi amplitude and neural timing on inspiration for the premandatory spontaneous breaths (solid line), mandatory breaths (dashed line), or after mandatory spontaneous breaths (dotted line). Neural expiratory time was prolonged significantly for the mandatory breaths (dashed line). The plot does not represent the shape of the Edi recruitment pattern but simply represents three points: the onset of Edi, peak of Edi, and onset of Edi of subsequent breath. Values are mean ± standard error. (Adapted, with permission, from Beck J, Tucci M, Emeriaud G, et al. Prolonged neural expiratory time induced by mechanical ventilation in infants. Pediatr Res. 2004;55:747–754.)
Figure 7-9
Inspiratory and expiratory asynchrony during mandatory breaths. Schematic of patient neural timing (upper bar) and ventilator timing (middle bar) during mandatory breaths. Upper bar shows the neural inspiratory time (TI) (yellow area) and neural expiratory time (TE) (white area). Middle bar shows periods of ventilator timing, including trigger delay, ventilator TI (green area), and ventilator TE. Bottom bar shows periods of infant–ventilator synchrony (white area) and asynchrony (red area). (Adapted, with permission, from Beck J, Tucci M, Emeriaud G, et al. Prolonged neural expiratory time induced by mechanical ventilation in infants. Pediatr Res. 2004;55:747–754.)
Using airway occlusion pressure (P0·1) and mean inspiratory flow rate (VT/TI) as indices of neuromuscular output, Weiss et al66 examined the effect of IMV on respiratory drive in stable ventilator-dependent patients who received mechanical ventilation for 12 to 24 hours each day. The IMV rate was initially set at 10 breaths/min and reduced gradually to 6, 3, 2, and 1 breath/min, and each level was maintained for 10 minutes. The VT of the mandatory breaths was set at 8 to 12 mL/kg. End-tidal partial pressure of carbon dioxide (PCO2) and arterial oxygen saturation did not change as the IMV rate was reduced. In the presence of stable chemical input, P0·1 and VT/TI measured during spontaneous breathing varied inversely with IMV rate. Increases in VT/TI with IMV rate reduction primarily were the result of progressive VT augmentation because inspiratory time (TI) and the duty cycle (TI/TTOT) remained unchanged at all IMV support levels. This VT response probably was related to changes in CO2 production that stimulated chemoreceptors. In acutely ill patients,42 the effect of IMV on P0·1, measured during spontaneous breathing, is similar to that in stable patients.66 Using another index of neuromuscular output, dP/dt (change in pressure over change in time) of esophageal pressure at the onset of ventilator triggering, Leung et al43 also demonstrated an inverse relationship between dP/dt and machine-assistance level.
As with neuromuscular output, IMV’s effects on breathing patterns are determined by assistance level. Both VT and unassisted breath frequency increase progressively with a decreasing IMV rate.42,43,57,67 Because VT of the unassisted breaths is relatively small compared with the mandatory breaths, dead-space-to-tidal-volume ratio inevitably increases. Consequently, spontaneous breathing frequency increases to maintain constant alveolar ventilation. Indeed, PaCO2 remained constant at all machine-assistance levels.67
The sensation of dyspnea during SIMV depends on the assistance level when applied without pressure-support ventilation (PSV) but was independent of assistance level when 10 cm H2O of pressure support was added to the unassisted breaths.43 During low assistance levels, dyspnea probably is secondary to increased inspiratory effort, whereas the high rate of nontriggering efforts may be responsible for the dyspnea at higher assistance levels. Knebel et al68 also demonstrated that during the weaning trial on SIMV, the sensation of dyspnea and patient anxiety were independent of support level. In that study,68 a PEEP of 5 cm H2O was maintained, which might have offset the increased inspiratory muscle work with a decreasing IMV rate.69,70
In summary, with IMV, the neuromuscular output of both the mandatory and unassisted breaths is downregulated only at high machine-assistance levels (approximately 75% to 80% of total machine assistance).57,58 Breath-by-breath adaptation to machine assistance does not occur. The fact that Edi was the same during assisted and preceding unassisted breathing suggests that neuromuscular output during the mandatory breaths must be determined at least in part by factors operating during the previous breath. This could be accounted for by the central neural feedback mechanism in the manner of a “memory” phenomenon as described by Eldridge71 (see below). Neuromuscular output increases inversely with the set IMV rate. Whereas VT and frequency increase when IMV rate decreases, neural inspiratory timing of the mandatory and unassisted breaths is of the same duration across all levels of machine assistance. Neural expiratory timing of the mandatory breaths is prolonged secondary to mechanical TI encroaching into neural TE, causing expiratory asynchrony. Respiratory timing asynchrony, however, can be substantially mitigated by using inspiratory and expiratory neural triggering with a neurally adjusted ventilatory assist.61,72,73
The effect of IMV on inspiratory muscle work and inspiratory effort is, as expected, determined by the level of machine assistance, and the response is similar in adults42,43,57 and neonates.67 A higher number of mandatory breaths reduces total workload and inspiratory effort in patients during acute respiratory failure42 or recovery.43,74,75 Marini et al42 measured work of breathing per liter of ventilation (joules per liter) and pressure-time product per breath during ACV and at SIMV support levels of 80%, 60%, 40%, 20%, and 0% of the ACV rate. When the SIMV support level was less than 80% of the ACV rate, the patient’s total workload increased markedly compared with that during ACV. Moreover, at all SIMV levels, the patient expended a similar effort during both the mandatory and spontaneous breaths, as gauged by pressure-time product per breath, which reflects the energy consumed by contracting respiratory muscles (patient effort). The patient does not vary effort on a breath-by-breath basis in response to machine unloading, as confirmed by others on the basis of Edi recording.57,60 It appears that during SIMV the heightened respiratory activity during the spontaneous breath is carried over to the subsequent mandatory breath in the manner of an “after discharge” or “memory” phenomenon.71 In fact, when 10 cm H2O of pressure support was applied during the intervening spontaneous breaths, inspiratory muscle effort decreased not only during the intervening breaths but also during the mandatory breaths (Fig. 7-10).43 This afterdischarge phenomenon is central in origin and unrelated to chemoreceptor or mechanoreceptor feedback or the state of sleep or wakefulness.71,76
Figure 7-10
Pressure-time product (PTP) per breath during synchronous intermittent mandatory ventilation (IMV) in the presence and absence of pressure support (PS). The addition of 10 cm H2O PS to the intervening spontaneous breaths decreased the overall PTP per breath. PTP per breath, however, did not differ between the mandatory and intervening spontaneous IMV breaths at each level of machine assistance, irrespective of whether PS was present or absent. (Adapted, with permission, from Leung, et al. Am J Respir Crit Care Med. 1997;155:1940–1948. Reprinted with permission of the American Thoracic Society. Copyright © American Thoracic Society.)
The patient workload during IMV is determined not only by machine-assistance level, but also by the IMV system employed.74,75 Patient workload or inspiratory effort during spontaneous breathing cycles with flow-triggered SIMV was significantly less than with a pressure-triggered SIMV system,74,75 particularly at a relatively low SIMV rate.75 The effect, however, of the triggering system on patient mandatory breath workload is conflicting. Sassoon et al75 demonstrated that the triggering system had no effect on patient mandatory-breath workload. In contrast, Giuliani et al74 found that patient mandatory-breath workload was significantly less with flow-triggered than with pressure-triggered SIMV irrespective of the mandatory-breath type (whether SIMV was flow-limited or pressure-limited). Moreover, patient workload with combined flow-triggered and pressure-limited mandatory breaths was lower than the other combinations of triggering and mandatory-breath types (flow-triggered flow limit and pressure-triggered flow and pressure limit).74 The decrease was so substantial that the pressure-time product per breath of the mandatory breath was significantly less than that of the intervening spontaneous breaths. It is not clear as to why the data of Giuliani et al74 differed from the data of others42,43,57,60 who demonstrated the lack of breath-by-breath adaptation to machine unloading.
In summary, during acute respiratory failure or recovery, patient workload during SIMV remains substantial unless the SIMV support is equivalent to or greater than 80% of the support during ACV.42,43 IMV often allows adequate gas exchange at lower support levels77 (see the section Intermittent Mandatory Ventilation and Assist-Control Ventilation) but at the expense of increased patient workload.42,43,57
Rationale, Advantages, and Limitations
IMV was developed as an alternative to CMV and ACV. The rationale for developing IMV was based on the premise that by maintaining spontaneous breathing amid the mechanical breaths, (a) machine breaths can be titrated to adjust alveolar ventilation, thereby decreasing the incidence of respiratory alkalemia,78 (b) distribution of inspired gas and ventilation-perfusion to the lung base improves, and physiologic dead space decreases,79 (c) mean airway pressure and pulmonary vascular resistance decrease, allowing PEEP titration and therefore more effective treatment of hypoxemia,80 and (d) pleural pressure decreases, resulting in better venous return and cardiac output.79 Unfortunately, subsequent clinical trials did not support some of the rationales (see the section Intermittent Mandatory Ventilation and Controlled Mechanical Ventilation).
IMV’s flexibility in providing a range of support levels makes it advantageous for use as a primary means of ventilator support81 and as a method for discontinuing mechanical ventilation.5 An inherent limitation of IMV is the fixed rate of machine breaths. When set at a very high rate, IMV functions as CMV that has been shown to rapidly lead to diaphragmatic muscle atrophy in both experimental animals82,83 and humans.84,85 During weaning from mechanical ventilation, the pace of decreasing the IMV rate potentially can prolong weaning when it is inappropriately slow.
Indications and Contraindications
IMV has been used as a primary means of ventilator support in postoperative patients86 and during acute respiratory failure of various etiologies.6,7,87–91 For treatment of acute respiratory failure in adults, North Americans apply SIMV with pressure support more frequently than ACV (49% vs. 27%, respectively).12 A similar trend is observed in Australia and New Zealand, 41% versus 17% with other mandatory pressure-limited modes of ventilation.13 In preterm neonates, the frequency of SIMV application in acute respiratory failure is higher than in adults, 60% versus 31% with ACV.14 However, it needs to be emphasized that in preterm infants in addition to the ventilatory strategy used, early treatment with surfactant is essential.92 The level of machine assistance must be tailored to the patient’s ventilatory requirement. The optimal mandatory-breath settings are similar in principle to those for ACV.93–95 A low IMV setting is contraindicated when the patient’s ventilatory demand is high; likewise, the setting should not be left high after the patient’s ventilatory requirement has decreased. A gradual decrease in the IMV rate is unwarranted when discontinuation from mechanical ventilation is imminent.
Comparison with Other Modes
Unlike IMV, CMV imposes a fixed breathing pattern. Hence, to some extent, a comparison favors IMV, which has been claimed to be superior to CMV for the following reasons: (a) it prevents the patient from “fighting the ventilator,” reducing the need for sedation and paralysis; (b) it prevents respiratory alkalosis; (c) it improves intrapulmonary gas distribution; (d) it lowers mean airway pressure, benefiting cardiac output and preventing barotrauma; (e) it decreases oxygen consumption (); (f) it prevents muscle atrophy and discoordination; and (g) it improves renal function.
IMV prevents the patient from “fighting the ventilator,” reducing the need for sedation and paralysis. Because the patient has no autonomy to alter breathing pattern with CMV, the patient will increase inspiratory effort, which is manifested as “fighting the ventilator” when ventilatory demand rises.78 No studies, however, have compared the respective sedative doses used during CMV and IMV.
IMV prevents respiratory alkalosis. In a prospective study comparing IMV and CMV in patients with acute respiratory failure, CMV resulted in a mean pH of 7.49 and a PaCO2 of 32.3 mm Hg, whereas IMV resulted in a mean pH of 7.44 and a PaCO2 of 39.9 mm Hg.7 The CMV rate, however, was set to suppress spontaneous respiratory effort, whereas, during IMV, the rate was adjusted downward continuously as long as pH remained greater than 7.30. Hence the result in favor of IMV is to be expected. When a normal pH is achieved at a low IMV rate, subsequent data suggested that it was at the expense of increased work of breathing.42,74 Moreover, some patients on mechanical ventilation who are allowed to set their own PaCO2 do not always choose a normal level (e.g., patients with brain injury). These patients will have persistent respiratory alkalosis regardless of ventilation mode.77,96
IMV improves intrapulmonary gas distribution. This hypothesis is based on the premise that in the supine position, spontaneous breathing causes inspired gas to be distributed preferentially to dependent lung regions because the dependent diaphragm, which is displaced cephalad, operates at an improved mechanical advantage.97 Conversely, during mechanical ventilation, ventilation is distributed preferentially to nondependent lung regions because the diaphragm is not used, and the nondependent lung and chest wall are more compliant.98 Therefore, IMV’s combination of spontaneous and mechanical breaths theoretically should result in a better matching of ventilation and perfusion.99 A comparison study evaluating IMV and CMV ventilation–perfusion distribution with the inert-gas elimination technique100 was performed in stable patients recovering from major abdominal aortic surgery.101 During CMV, VT was set at 14 to 16 mL/kg with a set rate of 8 to 10 breaths/min. During SIMV, the rate was set at 50% of the CMV rate (4 to 5 breaths/min); a pressure support of 5 to 8 cm H2O was added to the intervening spontaneous breaths to overcome the ventilator circuit and endotracheal tube resistance.102 Each ventilator mode was maintained for 45 minutes. Compared with CMV, physiologic dead space increased with SIMV (22.0% vs. 26.8%, respectively; p < 0.05) and was associated with a significant increase in
, resulting in a similar PaCO2. The SIMV perfusion distributions remained unaltered. This study, using the inert-gas elimination technique, shows that IMV does not improve ventilation–perfusion distributions.
IMV lowers mean airway pressure, benefiting cardiac output and preventing barotrauma. Because IMV intersperses spontaneous breaths between mechanical breaths, mean airway pressure averaged over time is lower than with CMV. The lower mean airway pressure results in maintenance of cardiac output. Several studies have shown a higher cardiac output with IMV than with CMV.90,91,103 The interactions between intrathoracic pressure associated with mechanical ventilation and cardiac performance, however, are quite complex.104 Right-ventricular and left-ventricular interaction, direct pressure on the heart, and changes in systemic and pulmonary venous return all play a role. The net effect of this interaction depends on left-ventricular filling pressure and reserve. Mathru et al105 compared the effect of CMV, IMV with 5 cm H2O PEEP (IMV–5PEEP), and IMV with 0 cm H2O PEEP (IMV–0PEEP) in two groups of patients following aortocoronary bypass surgery. VT and ventilator rate were adjusted to achieve a PaCO2 of between 35 and 40 mm Hg. One group had normal left-ventricular function. The second group had decreased left-ventricular reserve: left-ventricular diastolic pressure of greater than 16 mm Hg and ejection fraction of less than 0.6. In the first group, IMV–0PEEP resulted in an increase in cardiac output of 27% compared with CMV. In the second group, however, IMV resulted in a significant decrease in cardiac output (19%) compared with CMV. IMV–5PEEP affected cardiac output similarly to CMV. These data indicate that when compared with CMV, IMV improves cardiac output in patients with normal left-ventricular function or hypovolemia, but it may be harmful in patients with poor left-ventricular reserve.
The frequency of barotrauma with IMV and CMV was compared in a retrospective study of 292 postoperative and nonsurgical patients who received mechanical ventilation for 24 hours or more.106 The ventilator VT was set at 12 to 15 mL/kg. The IMV rate was set at 6 breaths/min or lower, provided that normocapnia was maintained. If hypercapnia developed, it was treated by increasing VT to 15 mL/kg and, if necessary, by increasing the IMV rate to 8 breaths/min. No sedation or muscle relaxants were used. In the CMV group, the rate was set at 12 breaths/min. Hypercapnia was corrected by increasing VT. The patients were sedated and paralyzed. Compared with the CMV group, patients receiving IMV had a significantly higher peak airway pressure (34 vs. 51 cm H2O, respectively) and PEEP/CPAP (15 vs. 27 cm H2O, respectively). Yet the barotrauma was 22% in the CMV group compared with 7% in the IMV group. The authors speculated that the less frequent barotrauma with IMV was related to the smaller number of mechanical breaths with large VT. Mean transpulmonary pressure, which may be responsible for the barotrauma, was not measured in either group.
IMV decreases oxygen consumption. Downs et al7 found that
was lower both during mechanical ventilation and 15 minutes after its discontinuation in patients ventilated with IMV versus CMV. The authors speculated that the higher
on CMV could be ascribed to respiratory alkalosis107,108 and abrupt withdrawal from mechanical ventilation rather than reflecting metabolic work of breathing. In contrast, Wolff et al103 showed that CO2 production (
) tended to be lower with CMV than with IMV. Because the respiratory quotient was similar with both ventilation modes, it is reasonable to assume that
during CMV was lower than that during IMV. In this study, PaCO2 was 39 and 44 mm Hg on CMV and IMV, respectively, suggesting that pH was within the normal range.103 This observation suggests that, in the absence of alkalemia,
is lower on CMV than on IMV.
IMV prevents muscle atrophy and discoordination. Maintaining spontaneous breathing activity during IMV has been proposed to achieve respiratory muscle conditioning and preserve respiratory muscle function.5 The periodic hyperinflation also may reinforce coordinated breathing.5,109 During CMV, muscle atrophy84,85
Stay updated, free articles. Join our Telegram channel
Full access? Get Clinical Tree
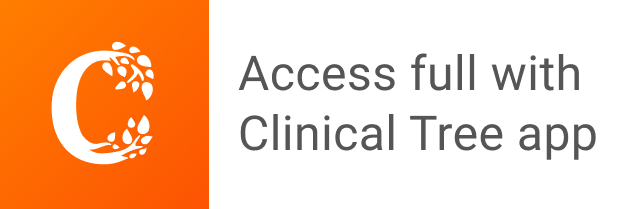