Effects of Mechanical Ventilation on Control of Breathing: Introduction
The main reasons for instituting mechanical ventilation are to decrease the work of breathing, support gas exchange, and buy time for other interventions to reverse the cause of respiratory failure.1 Mechanical ventilation can be applied in patients who are making or not making respiratory efforts, whereby assisted or controlled modes of support are used, respectively.1 In patients without respiratory efforts, the respiratory system represents a passive structure, and thus the ventilator is the only system that controls breathing. During assisted modes of ventilator support, the patient’s system of control of breathing is under the influence of the ventilator pump.2–4 In the latter instance, ventilatory output is the final expression of the interaction between the ventilator and the patient’s system of control of breathing. Thus, physicians who deal with ventilated patients should know the effects of mechanical ventilation on control of breathing, as well as their interaction. Ignorance of these issues may prevent the ventilator from achieving its goals and also lead to significant patient harm.
Physiology
The respiratory control system consists of a motor arm, which executes the act of breathing, a control center located in the medulla, and a number of mechanisms that convey information to the control center.5,6 Based on information, the control center activates spinal motor neurons that subserve the respiratory muscles (inspiratory and expiratory); the intensity and rate of activity vary substantially between breaths and between individuals. The activity of spinal motor neurons is conveyed, via peripheral nerves, to respiratory muscles, which contract and generate pressure (Pmus). According to equation of motion, Pmus at time t during a breath is dissipated in overcoming the resistance (Rrs) and elastance (Ers) of the respiratory system (inertia is assumed to be negligible) as follows:
where ΔV(t) is instantaneous volume relative to passive functional residual capacity and (t) is instantaneous flow. Equation (1) determines the volume–time profile and, depending on the frequency of respiratory muscle activation, ventilation. Volume–time profile affects Pmus via neuromechanical feedback; inputs generated from other sources (cortical inputs) may modify the function of control center. Ventilation, gas-exchange properties of the lung, and cardiac function determine arterial blood gases, termed arterial oxygen tension (PaO2) and arterial carbon dioxide tension (PaCO2), which, in turn, affect the activity of control center via peripheral and central chemoreceptors (chemical feedback). This system can be influenced at any level by diseases or therapeutic interventions.
During mechanical ventilation, the pressure provided by the ventilator (Paw) is incorporated into the system.3 Thus, the total pressure applied to respiratory system at time t [PTOT(t)] is the sum of Pmus(t) and Paw(t). As a result, the equation of motion is modified as follows:
The relationships of Equation (2) determine the volume–time profile during mechanical ventilation, which via neuromechanical, chemical, and behavioral feedback systems affects the Pmus waveform (Fig. 35-1). The ventilator pressure, by changing flow and volume, may influence these feedback systems and thus alter either the patient’s control of breathing itself or its expression. In addition, Pmus, depending on several factors, alters the Paw waveform (Fig. 35-1). Thus, during assisted mechanical ventilation (i.e., Pmus ≠ 0), ventilatory output is not under the exclusive influence of patient’s control of breathing; instead, it represents the final expression of an interaction between ventilator-delivered pressure and patient respiratory effort.
Figure 35-1
Schematic of variables that determine the volume–time profile during mechanical ventilation. Neuromechanical, chemical, and behavioral feedback systems are the main determinants of Pmus. The functional operation of the ventilator mode (triggering, control, and cycling-off variables) and patient-related factors (namely, respiratory system mechanics and the Pmus waveform) determine the response of the ventilator to Pmus. ΔV(t), instantaneous volume relative to passive functional residual capacity of respiratory system; Ers, elastance of the respiratory system; Paw(t), airway (ventilator) pressure; Pmus(t), instantaneous respiratory muscle pressure; Rrs, resistance of the respiratory system; RS, respiratory system; (t), instantaneous flow.
Effects of Mechanical Ventilation on Feedback Systems
Chemical feedback refers to the response of Pmus to PaO2, PaCO2, and pH.5–7 In spontaneously breathing and mechanically ventilated patients, this system is an important determinant of respiratory motor output both during wakefulness and sleep.7–11
Mechanical ventilation can influence chemical feedback simply by altering the three variables PaO2, PaCO2, and pH. Hypoxemia, hypercapnia, or acidemia may be corrected by mechanical ventilation and thus modify activity of the medullary respiratory controller via peripheral and central chemoreceptors.5,12 The effects of mechanical ventilation on gas-exchange properties of the lung are beyond the scope of this chapter and are discussed in Chapter 37. In this chapter, the fundamental elements of the response of respiratory motor output to chemical stimuli, their relationship to unstable breathing, and the operation of chemical feedback during mechanical ventilation are reviewed.
Carbon dioxide (CO2) is a powerful stimulus of breathing.5,12 This stimulus, expressed by PaCO2, largely depends on the product of tidal volume (VT) and breathing frequency (f) (i.e., minute ventilation) according to Equation (3):
where is CO2 production, and VD/VT is the dead-space-to-tidal-volume ratio. Because minute ventilation is an adjustable variable in ventilated patients, understanding the relationship between respiratory motor output and CO2 stimuli is of fundamental importance.
Several studies have examined the respiratory motor output to CO2 in ventilated, conscious, healthy subjects.7,13–16 Major findings include
Manipulation of PaCO2 over a wide range has no appreciable effect on respiratory rate. Despite hypocapnia, subjects continue to trigger the ventilator with a rate similar to that of eucapnia. Respiratory rate increases slightly when PaCO2 approaches values well above eucapnia (Fig. 35-2).
The intensity of respiratory effort (respiratory drive) increases progressively as a function of PCO2. This response is evident even in hypocapnic range. The response slope increases progressively with increasing CO2 stimuli, reaching its maximum in the vicinity of eucapnic values (see Fig. 35-2).
There is no fundamental difference in the response to CO2 between various ventilator modes.
Above eupnea, the slope of the response does not differ significantly with that observed during spontaneous breathing, suggesting that mechanical ventilation per se does not considerably modify the sensitivity of respiratory system to CO2.
Figure 35-2
Schematic of the response of respiratory frequency (open squares) and pressure-time product of the inspiratory muscles per breath (an index of the intensity of patient effort, closed squares), both expressed as a percentage of values during spontaneous eupnea (baseline), to CO2 challenge in conscious healthy subjects ventilated with a high level of ventilator assistance. PETCO2 is end-tidal PCO2, and the dotted vertical line is PETCO2 during spontaneous breathing (eupnea). Contrast the vigorous response of intensity of inspiratory effort to CO2, even in the hypocapnic range, with the response of respiratory frequency, which remains at eucapnic level over a broad range of CO2 stimuli. The response is based on data from references 7 and 13 to 16.
During sleep (or sedation), the response of respiratory motor output to CO2 differs substantially from that during wakefulness, secondary to loss of the suprapontine neural input to the medullary respiratory controller.10,17 In ventilated sleeping subjects, a decrease in PaCO2 by a few millimeters of mercury causes apnea.10 Respiratory rhythm is not restored until PaCO2 has increased significantly above eupneic levels. The difference between eupneic PaCO2 and PaCO2 at apneic threshold, referred to as CO2 reserve,18 depends on several factors (see Response of Respiratory Motor Output to Chemical Stimuli—Chemical Stimuli and Unstable Breathing). This reserve determines the propensity of an individual to develop breathing instability during sleep; propensity increases as CO2 reserve decreases. Similar to wakefulness, the response of respiratory motor output to CO2 is mediated mainly by the intensity of respiratory effort, whereas respiratory rate decreases abruptly to zero (apnea) when the CO2 apneic threshold is reached.19
The effects of mechanical ventilation on the response of respiratory motor output to stimuli other than CO2 have not been studied adequately. In a steady state during wakefulness, the effects of oxygen (O2) and pH on breathing pattern are similar qualitatively to that observed with CO2: Changes in O2 and pH mainly alter the intensity of patient effort, whereas respiratory rate is affected considerably less.5,12 There is no reason to expect a different response pattern during mechanical ventilation. Indeed, this is the case regarding the hypoxic response in normal conscious subjects ventilated in assist-control mode during eucapnia.20 Indirect data also revealed that during eucapnia, the sensitivity of respiratory motor output to hypoxia was not modified by mechanical ventilation.20 During mild hypocapnia, however, the response was attenuated, whereas at moderate hypocapnia (end-tidal PCO2 approximately 31 mm Hg) the response was negligible. The latter observations may be relevant clinically because ventilated patients do not always keep PaCO2 at eucapnic levels and can become hypocapnic.16
The response pattern of respiratory motor output to CO2 during sleep is relevant to the occurrence of periodic breathing in mechanically ventilated patients. Studies indicate that this breathing pattern might increase the morbidity and mortality of critically ill patients because it can cause sleep fragmentation and patient–ventilator dyssynchrony.21–23 Sleep deprivation may cause serious cardiorespiratory,24,25 neurologic,26,27 immunologic, and metabolic consequences.28–31
The following is a brief review of the factors that can lead to unstable breathing. In a closed system governed mainly by chemical control (such as occurs during sleep or sedation), a transient change in ventilation at a given metabolic rate (Δinitial) will result in a transient change in alveolar gas tensions. This change is sensed by peripheral and central chemoreceptors, which, after a variable delay, exert a corrective ventilatory response (Δ
corrective) that is in the opposite direction to the initial perturbation32,33 (Fig. 35-3). The ratio of Δ
corrective to Δ
initial defines the loop gain of the system.32 Loop gain is a dimensionless index that is the mathematical product of three types of gains: plant gain (the relationship between the change in gas tensions in mixed pulmonary capillary blood and Δ
initial), feedback gain (the relationship between gas tensions at the chemoreceptor level and those at the mixed pulmonary capillary level), and controller gain (the relationship between Δ
corrective and the change in gas tensions at the chemoreceptor level) (Fig. 35-3). Loop gain has both a magnitude and a dynamic component.32,33 In this system, instability occurs when the corrective response is 180 degrees out of phase with initial disturbance (dynamic component) and loop gain is greater than 1 (magnitude component). This instability leads to fluctuation in chemical stimuli, namely, PCO2. If PCO2 reaches the apneic threshold, apnea occurs.
Figure 35-3
Schematic of the variables that determine the propensity of an individual to develop periodic breathing in a closed system dominated by chemical feedback. Loop gain is the product of three gains: plant, feedback, and controller. Instability occurs when Δcorrective (the final response) is 180 degrees out of phase with Δ
initial (the transient initial perturbation) and Δ
corrective/Δ
initial is greater than 1. Mechanical ventilation, by affecting almost all variables of the system (↑, increase; ↔, no change; ↓, decrease), may change both the magnitude and the dynamic component of loop gain and thus the propensity of an individual to develop periodic breathing. CO, cardiac output; ΔPCCO2 and ΔPCO2, the difference in partial pressures of CO2 and O2 in mixed pulmonary capillary blood, respectively; ΔPchCO2 and ΔPchO2, the difference in partial pressure of CO2 and O2 at chemoreceptors (peripheral and central), respectively; Ers and Rrs, elastance and resistance of respiratory system, respectively; FRC, functional residual capacity; LG, Gplant, Gfeedback, and Gcontroller, loop, plant, feedback, and controller gains, respectively;
alveolar partial pressure of CO2; Paw, airway (ventilator) pressure; Pmus, pressure developed by respiratory muscles;
, ventilation–perfusion ratio; VD/VT, dead-space fraction.
Positive-pressure breathing exerts multiple effects on loop gain by influencing almost all the factors that determine plant, feedback, and controller gains. The effects are complex and at times opposing and variable (Table 35-1; see also Fig. 35-3). Nevertheless, the effect of mechanical ventilation on controller gain exerts the most powerful influence on the propensity to develop breathing instability.8,19,21,23 The magnitude and direction of the change in controller gain depends on the ventilator mode, the level of assistance, the mechanics of the respiratory system, and the Pmus waveform (see the section Interactive Effects of Patient-Related Factors and Ventilator on Control of Breathing).8,16,19,21 Disease states as well as medications (e.g., sedatives) also may interfere with the effects of mechanical ventilation on loop gain. For example, positive-pressure ventilation may increase or decrease cardiac output, causing corresponding changes in circulatory delay depending on cardiac function and intravascular volume (see Chapter 36).34–37 It has been shown that nocturnal mechanical ventilation in patients with congestive heart failure decreases the frequency of Cheyne-Stokes breathing, presumably by causing an increase in cardiac output secondary to afterload reduction.38–40 Sedatives at moderate doses, commonly used in ventilated patients, decrease considerably the loop gain, partly mitigating the effect of mechanical ventilation on controller gain and thus promote ventilatory stability.41
Gain Factors (Influence) | Ventilator Effect* | Gain Change |
---|---|---|
Lung volume (stabilizing) | ↑ | ↓Gplant |
Cardiac output (destabilizing) | ↓ | ↑Gplant, ↑Gfeedback |
Thoracic blood volume (destabilizing) | ↓ | ↑Gfeedback |
Paw response to Pmus (destabilizing) | ↑ | ↑Gcontroller |
Alveolar ![]() | ↓ | ↓Gplant |
Alveolar ![]() | ↑ | ↓Gplant, ↓Gcontroller |
Respiratory elastance (destabilizing) | ↓ | ↑Gcontroller |
In addition to CO2, O2 and pH can play a key role in producing unstable breathing in ventilated patients during sleep (or sedation). It is well known that hypoxia, acting via peripheral chemoreceptor stimulation, decreases PaCO2. The result reduces the plant gain (stabilizing influence); for a given change in alveolar ventilation, PaCO2 will change less when baseline PaCO2 is low than when it is high.18 Hypoxia, however, increases the controller gain to a much greater extent42 because the slope of ventilatory response to CO2 below eupnea increases,12 a highly destabilizing influence.32,33 Similar principles apply if pH is considered as a chemical stimulus; acidemia decreases the plant gain (lowers PaCO2) and increases, to a much lesser extent, the controller gain.18,42 During mechanical ventilation, the propensity to unstable breathing in the face of changing O2 and pH stimuli depends on a complex interaction between the effects of these stimuli and mechanical ventilation on plant, feedback, and controller gains (Fig. 35-4; see also Table 35-1).
Figure 35-4
Tidal volume (VT), airway pressure (Pm), integrated diaphragmatic electrical activity (Edi, arbitrary units), and partial pressure of end-tidal CO2 (PETCO2) in a tracheostomized dog during non–rapid eye movement sleep without and with pressure-support ventilation at a pressure level that caused periodic breathing. (A) At a background of 5 hours of metabolic acidosis (pH 7.34, HCO3− 16 mEq/L, 30 mm Hg). (B) At a background of 1 hour of metabolic alkalosis (pH 7.51, HCO3− 35 mEq/L,
44 mm Hg). (C) During hypoxia (
47 mm Hg,
31 mm Hg). At a background of metabolic acidosis, CO2 reserve was quite high; consequently, the pressure level that caused periodic breathing (20 cm H2O) was significantly higher than the corresponding values (approximately 10 cm H2O) during metabolic alkalosis or hypoxia. Hyperventilation during spontaneous breathing was similar during metabolic acidosis and hypoxia (similar stabilization influence via a decrease in plant gain secondary to low
), indicating that the destabilizing influence of hypoxia was caused by an increase in controller gain (hypoxic increase in the slope of CO2 below eupnoea). (Used, with permission, from Dempsey et al. J Physiol. 2004;560:1–11, based on data from Nakayama H, Smith CA, Rodman JR, et al. Effect of ventilatory drive on carbon dioxide sensitivity below eupnea during sleep. Am J Respir Crit Care Med. 2002;165:1251–1260.)
The ventilator mode is a major determinant of driving pressure for flow and thus arterial blood gases. Before discussing the operation of chemical feedback, it is useful to review briefly the functional features of three main modes of assisted ventilation, namely, assist-control ventilation (ACV), pressure-support ventilation (PSV), and proportional-assist ventilation (PAV) (for detailed descriptions, see Chapters 6, 8, and 12). Figure 35-5 shows the response of the ventilator to respiratory effort in a representative subject ventilated with each mode in the presence and absence of CO2 challenge.16 With CO2 challenge, Paw decreases with ACV, it remains constant with PSV, and it increases with PAV. Pressure-time product of inspiratory muscle pressure (PTP-PmusI) is an accurate index of the intensity of inspiratory effort.43 With ACV, the ratio of VT to PTP-PmusI per breath (neuroventilatory coupling) decreases with increasing Pmus; the ratio is largely independent of inspiratory effort with PAV. With PSV, VT/PTP-PmusI
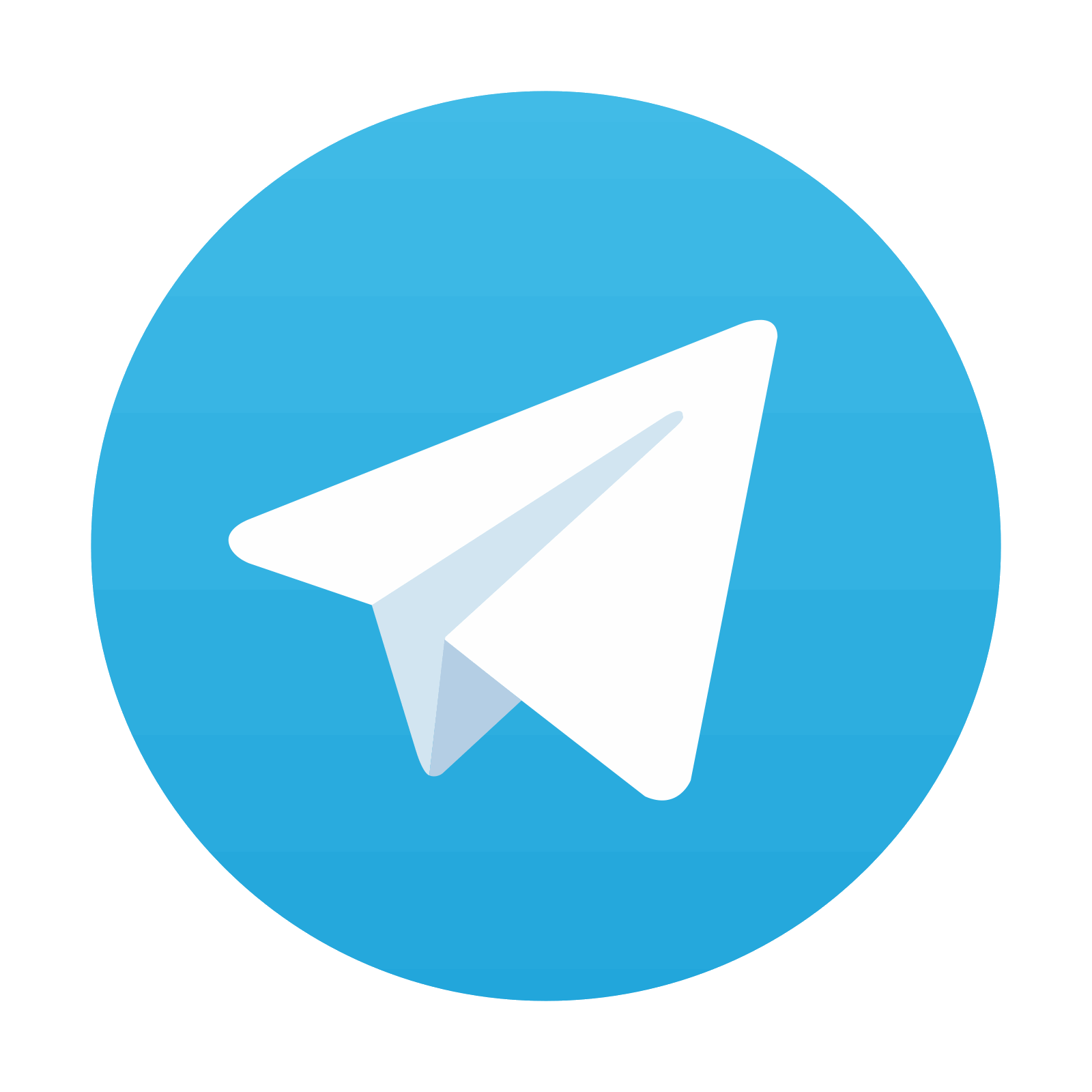
Stay updated, free articles. Join our Telegram channel
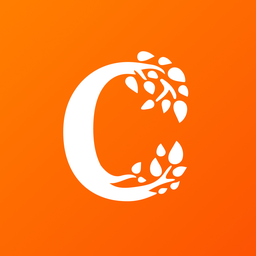
Full access? Get Clinical Tree
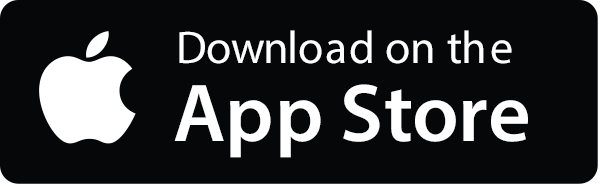
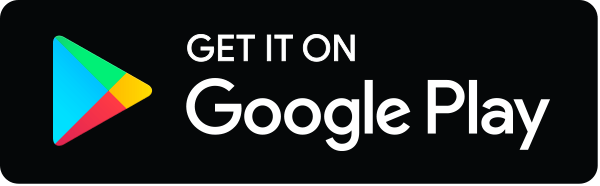
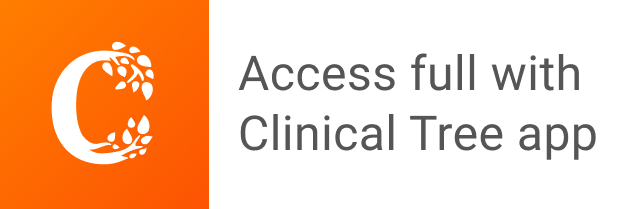