Effect of Mechanical Ventilation on Gas Exchange: Introduction
The major function of the lung is to exchange physiologic (respiratory) gases, namely oxygen (O2) and carbon dioxide (CO2). Once the lungs fail as a gas exchanger, arterial hypoxemia, hypercapnia, or both appear and respiratory failure ensues. Arterial PO2 (PaO2) and (
) are the measurable end-point variables used routinely by clinicians to manage patients with acute respiratory failure. When the latter is severe, mechanical ventilation is then considered the final strategy for treating patients.
Relevance
Classically, the mechanisms of hypoxemia are alveolar hypoventilation, limitation of alveolar to end-capillary O2 diffusion, intrapulmonary shunt, and ventilation–perfusion () imbalance; the major causes of hypercapnia are alveolar hypoventilation and
mismatching.1 Ideally, it would be of great interest to solely manage respiratory blood-gas measurements, such as alveolar-arterial PO2 difference P(A-a)O2, venous admixture ratio (
), and physiologic dead space (dead-space-to-tidal-volume ratio [VD/VT]), as a general marker of the overall function of the lung. Thus, impaired or improved results of these variables, whose principal merits are their simplicity and relative ease of measurement, could reflect impaired or improved pulmonary gas exchange. Unfortunately, all these variables reflect not only the state of the lung, but also the conditions under which the lung is operating. These conditions, which uniquely determine the PO2 and
in any single gas-exchange unit of the lung, are the
ratio, the composition of inspired gas, and the composition of mixed venous blood heavily modulated by the behavior of the cardiac output.2,3 It is important to appreciate the key role played by these three factors governing the respiratory gases in any single gas-exchange unit.3
Physiology: Multiple Inert Gas Elimination Technique
This chapter reviews the effect of ventilator support on pulmonary gas exchange using the multiple inert gas elimination technique (MIGET), an approach that represents a major conceptual breakthrough in our understanding of pulmonary medicine pathophysiology in disease states.4–7 MIGET has three major advantages. First, it estimates the pattern of pulmonary blood flow and alveolar ventilation and calculates the mismatch of relationships. Second, it partitions the P(A-a)O2 into determinants of intrapulmonary shunt,
inequality, and diffusion limitation to O2. Third, it apportions and unravels arterial oxygenation into intrapulmonary, namely the latter three factors, and extrapulmonary components, that is, inspired PO2, overall ventilation, cardiac output, and O2 consumption. Of paramount importance is the ability to perform measurements at any fractional inspired oxygen concentration (FIO2) without perturbing the vascular and bronchomotor tones.8
Figure 37-1 illustrates the distribution obtained with MIGET in a healthy, young individual at rest breathing ambient air. Each data point represents a particular amount of blood flow or alveolar ventilation. Both overall pulmonary perfusion and total ventilation correspond to the sum of the respective data points (the lines have been drawn for clarity only). These quantities (distributions) are plotted against a broad range (50) of
ratios, from zero (intrapulmonary shunt) to infinity (dead space), on a log scale. The unimodal profile of each distribution has three main characteristics: symmetry, location around a
ratio of 1, and narrowness (very little dispersion). Note that there is no inert-gas shunt (contrasted with the concept of venous admixture ratio), because the tracer nature of inert gases utilized for MIGET is insensitive to the presence of postpulmonary shunt (i.e., the bronchial and thebesian circulations). Inert-gas physiologic dead space is also slightly lower than Bohr dead space because it does not include the alveolar units, which have an alveolar
that is lower than
.
Nature of Gas Exchange in Disease States
From a clinical standpoint, the three principal mechanisms of altered arterial respiratory gases during spontaneous breathing in any pulmonary disease state are mismatching, increased intrapulmonary shunt, and alveolar hypoventilation. The role of diffusion limitation to O2 is modest and plays a role in patients with pulmonary fibrosis9 and in healthy individuals under very extreme conditions at high altitude.10 During mechanical ventilation, however, alveolar hypoventilation is controlled in such a way that
does not represent a problem.
inequalities play a pivotal role in disorders characterized by chronic lung disease (“dry lung”), namely during exacerbations of chronic obstructive pulmonary disease (COPD) and bronchial asthma, which have in common expiratory airflow limitation and large pulmonary volumes. Increased intrapulmonary shunt is a key determinant of hypoxemia in conditions characterized by acute lung injury (“wet lung”), such as acute lung injury (ALI), and its most severe form, acute respiratory distress syndrome (ARDS), and life-threatening pneumonia, all of which have small lung volumes.11
The main mechanisms of pulmonary gas exchange are extensively reviewed (see the section Acute Respiratory Failure “Wet Lung”) during mechanical ventilation in ALI or ARDS, pneumonia, COPD, and asthma—the most common conditions in the critical care setting. In each condition, the gas-exchange abnormalities at maintenance FIO2 and while breathing 100% O2 are addressed. Likewise, the effects of external positive end-expiratory pressure (PEEP) and intrinsic PEEP (PEEPi) on gas exchange, and the effects of several ventilator settings are considered.
This section reviews the two most frequent disorders seen in the intensive care unit, ARDS and severe life-threatening pneumonia, together with a short review of gas exchange in patients with head trauma and following cardiac surgery. It can be difficult to differentiate ALI or ARDS from the other disorders—after all, ALI or ARDS is a constellation of many entities, among which pneumonia and the other conditions of acute respiratory failure are common causes. From a gas-exchange viewpoint, however, ALI or ARDS and pneumonia show different functional findings. Furthermore, the response to high FIO2 differs substantially.
Severe acute respiratory failure in previously healthy subjects may result from a primary infectious lung process or a more widespread, noninfectious process, namely either ALI or ARDS. The latter entity is characterized by severe hypoxemia refractory to high FIO2, and differences are related to the severity of gas-exchange abnormalities.12
The main cause of hypoxemia in patients with ALI or ARDS is a characterized by an increased intrapulmonary shunt, averaging 20% or more of cardiac output. In approximately half the patients, however, there are considerable additional areas with low ratios: A moderate percentage of total pulmonary blood flow is distributed to areas of the lung with reduced ventilation.13
Figure 37-2 illustrates the profiles of two representative distributions of patients with ARDS during mechanical ventilation with PEEP (at FIO2, 0.5).14 Although both patterns show marked amounts of shunt and dead space, the main body of the dispersion of blood flow is different. In one patient, the dispersion is narrowly unimodal (right panel). In the other, the pattern of perfusion is broadly unimodal, that is, blood flow is distributed to areas with low
ratios (usually less than 10% of cardiac output) (left panel). The former profile reflects an “all-or-none” phenomenon: pulmonary perfusion is essentially diverted to two lung areas, those with ventilation that is normal and proportional to blood flow, and those that are completely unventilated.15 The presence of areas with low
ratios suggests the coexistence of areas with partially filled alveolar spaces or alveolar units in which ventilation is reduced compared to blood flow (because of increased airways resistance) or both. A few patients showed an increase of alveolar ventilation distributed to high
ratios, including a bimodal pattern of the dispersion of ventilation; in contrast, dead space was increased in most patients. These two findings may reflect areas with reduced pulmonary blood flow secondary to the effects of external PEEP, although an additional influence of pulmonary vascular derangement cannot be ruled out.16
Figure 37-2
Two patterns of distributions in patients with ARDS (with PEEP). Note that intrapulmonary shunt and dead space were considerably increased in both individuals. Notice also the presence of some amount of blood flow distributed to units with low
(left panel). (Reprinted with permission of the American Thoracic Society. Copyright © 2012 American Thoracic Society. Reyes A, Roca J, Rodriguez-Roisin R, et al. Effect of almitrine on ventilation-perfusion distribution in adult respiratory distress syndrome. Am Rev Respir Dis. 1988;137:1062–1067. Official Journal of the American Thoracic Society. Modified with permission of the American Thoracic Society.)
There was no limitation to the diffusion of O2, as reflected by the close agreement between predicted PaO2 (according to MIGET, this reflects the underlying amount of intrapulmonary shunt and mismatch only) and measured PaO2 (Fig. 37-3).15,17 When measured PaO2 is not significantly different from the predicted PaO2, this suggests that other potential causes of hypoxemia, namely diffusion limitation for O2, increased postpulmonary shunt, and/or augmented intrapulmonary O2 consumption,18 are not occurring.
Figure 37-3
Plots of predicted (estimated by MIGET) PaO2 versus measured PaO2 in patients with ARDS (left panel; open symbols = without PEEP; closed symbols = with PEEP) and with severe pneumonia (right panel; open symbols = with mechanical ventilation; closed symbols = without mechanical support). Note the good agreement between both variables in each clinical condition (dashed line = regression line). (Used, with permission, from Dantzker et al.15 and Gea et al.17)
The concept of an increase in intrapulmonary shunt while breathing 100% oxygen in patients with acute respiratory failure is old.19,20 Earlier data using MIGET, however, did not support this view.16 It was considered that areas with critical inspiratory ratios may remain partly open and facilitate O2 transfer; potential mechanisms include the efficiency of collateral ventilation, interdependence of surrounding lung parenchyma, or the interaction of mechanical forces exerted during artificial ventilation.8 No studies, however, properly addressed this question.
We have shown that PaO2 increases modestly (≤300 mm Hg) and intrapulmonary shunt increases considerably (approximately 35%) during hyperoxic breathing over 1 hour, suggesting the development of reabsorption atelectasis (Fig. 37-4).8,21 The increase in intrapulmonary shunt was noticed within less than half an hour, confirming the theoretical analysis on the minimum time required for collapse of alveolar units with various fixed inspired ratios according to the different levels of FIO2.8 The limited increase in PaO2 while breathing 100% O2 indicates that increased intrapulmonary shunt is the key determinant of hypoxemia in ALI and ARDS. Furthermore, the increments in shunt persisted for 1 hour after resuming maintenance FIO2, indicating the persistence of atelectasis. Of note, this worsening of shunt was also accompanied by a small but significant increase in
, possibly explained by the Haldane effect (Fig. 37-5). The Haldane effect is the increase in
at a given arterial CO2 content that occurs in response to an increase in arterial O2 saturation. The dispersions of blood flow and alveolar ventilation remained unchanged.
Figure 37-4
Effect of breathing 100% oxygen (right panel) in a representative patient with ARDS (with PEEP). Compared with low FIO2 (left panel), PaO2 increased modestly (approximately 300 mm Hg), but intrapulmonary shunting increased moderately; in contrast, both the dispersion of blood flow (Log SD Q) and the amount of blood flow distributed to areas with low ratios remained unchanged. This suggests the development of reabsorption atelectasis without release of hypoxic vasoconstriction. Dead space tended to increase.
Figure 37-5
Sequence of PaO2/FIO2 (in millimeters of mercury), shunt (in percentage of cardiac output), and Log SD Q (dimensionless). In patients with ALI (open circles) arterial oxygenation and Log SD Q remained almost unchanged, whereas shunt increased significantly, suggesting reabsorption atelectasis. In contrast, in patients with COPD (solid squares), Log SD Q and arterial oxygenation substantially increased whereas shunt remained low and unvaried, indicating release of hypoxic vasoconstriction. For further explanation, see text. (Used, with permission, from Santos et al.21)
The increase in shunt not accompanied with release of hypoxic pulmonary vasoconstriction in patients with ALI or ARDS is compatible with the concept that, at any level of FIO2, units with low cannot redistribute blood flow from areas of intrapulmonary shunt or very low
ratios, because their vascular resistance remains unchanged.8
The gas-exchange response to external PEEP illustrates one of the best examples of the complex interplay between intrapulmonary and extrapulmonary determinants of respiratory gases.5,16 Several studies have assessed this action in patients with ALI or ARDS.
In a seminal study,15 which measured distributions at increasing levels of PEEP, two distinct patterns were shown (Fig. 37-6). In some patients, the
pattern remained unchanged despite progressive increases of PEEP. In others, there was a broadening of the dispersion of ventilation because areas with high
ratios increased and ventilation was redistributed to infinity
ratios. In each patient, however, PEEP led ultimately to a marked decrement in cardiac output distributed to unventilated (shunt) areas or poorly ventilated
units and to a considerable increase in dead space. The final result was a substantial optimization of PaO2.
Figure 37-6
The application of 16 cm H2O of PEEP in a patient with ARDS induced a reduction of shunt and an increase of dead space, whereas distributions remain essentially unaltered (top, from left to right). In another patient, 12 cm H2O of PEEP caused similar effects on shunt and dead space, but
distributions are broadened (bottom, from left to right). For further explanation, see text. (Reprinted with permission of the American Thoracic Society. Copyright © 2012 American Thoracic Society. Dantzker DR, Brook CJ, Dehart P, et al. Ventilation-perfusion distributions in the adult respiratory distress syndrome. Am Rev Respir Dis. 1979;120:1039–1052. Official Journal of the American Thoracic Society. Modified with permission of the American Thoracic Society.)
Subsequently, the response to external PEEP was investigated while cardiac output was kept constant at control values with dopamine.22 During PEEP, PaO2, mixed venous PO2, and oxygen delivery increased significantly, whereas the venous admixture ratio decreased markedly. Similarly, shunt decreased substantially, and pulmonary blood flow was redistributed from nonventilated units with zero
ratios to areas with normal
ratios. Dead space increased slightly, but the dispersion of the alveolar ventilation did not vary. Because PEEP causes redistribution of extravascular lung water from alveoli to peribronchial and perivascular spaces,23 these data suggest that the improvement in pulmonary gas exchange with external levels of PEEP results from the reopening of collapsed airways and alveoli. The key message from this study is that the avoidance of the reduction of cardiac output during PEEP application enhances a more optimal gas exchange.
In another study,24 the gas-exchange response to increments in PEEP was studied. In most patients, PaO2 improved substantially because of a decrease in intrapulmonary shunt or in the zone of low ratio, or a derecruitment of blood flow from areas with shunt to those with low or normal
ratio; blood flow reductions to low
areas were less predictable. When PaO2 did not vary in response to PEEP, there were no variations in the
relationships. An increase either in poorly perfused
units or in dead space was shown in only a few individuals. The beneficial effect of PEEP on PaO2 could not be predicted by the etiology of ARDS or the severity of abnormal gas exchange. As expected, cardiac output and mixed venous PO2 decreased progressively as PEEP was increased. The mean change in cardiac output was essentially similar between PEEP trials irrespective of the improvement in PaO2.
Experimentally, low levels of PEEP (5 to 10 cm H2O) decrease physiologic dead space by reducing both shunt and mismatch.25 At higher levels of PEEP, however, physiologic dead space increases because both ventilation to high
regions and anatomic dead space increase while the efficiency of CO2 elimination by the lungs is diminished.
All in all, these studies are of interest for two reasons. First, these findings indicate that the beneficial effects of PEEP in ALI and ARDS essentially result from a redistribution of blood flow from severely injured (shunt) areas to poorly or normally ventilated alveolar units. This seems to be related to the reopening of collapsed alveoli and airways rather than to a decrease of cardiac output by itself. Alternatively, the depression of cardiac output may reduce shunt or blood flow distributed to units with poorly ventilated ratios.2 It has been shown both clinically and experimentally, using pharmacologic and mechanical techniques, that changes in cardiac output lead to directionally similar changes in shunt. Likewise, in many instances, the shunt fraction also changes in a similar direction to changes in the mixed venous and negatively with pulmonary vascular resistance.26
Second, in two of the studies in which the values of mixed venous PO2 were reported, this variable either increased22 or remained stable24 when cardiac output was reduced. Arterial PO2 increased during PEEP because the beneficial effects on gas exchange, essentially secondary to the reduction in shunt (intrapulmonary factor), were not offset by the simultaneous deleterious effect on PaO2 secondary to decreased cardiac output (extrapulmonary factor), which had allowed mixed venous PO2 to decrease, other factors being equal. If the reduction in cardiac output had been more severe, PaO2 would have remained unaltered or even decreased despite the reduction in shunt.16 Alternatively, the observation that mixed venous PO2 values did not always vary in the same direction as cardiac output raises the question of the pathologic supply dependence between O2 delivery and the O2 consumption.27 Such patients may respond to the depression of cardiac output during PEEP by reducing O2 consumption.
The development of high peaks and the moderate-to-severe increments of dead space during PEEP are consistent with experimental data indicating that the reduction of cardiac output and the depression of perfusion in the uppermost regions of the lung determine changes in the pattern of ventilation with PEEP.28
Over the last 10 years, increasing emphasis has been placed on avoiding ventilator-associated lung injury and the use of a protective ventilator strategy, accepting an increase in if necessary (permissive hypercapnia), in patients with ALI or ARDS. Ventilator-associated lung injury29 is defined as lung damage with characteristic features of ARDS that can occur in patients receiving mechanical ventilation. The two most important factors responsible for ventilator-associated lung injury are thought to be: (a) the association of alveolar overdistension and high transpulmonary pressure; and (b) repeated alveolar collapse and reopening owing to ventilation at inappropriate tidal ranges of transpulmonary pressure. The prevention of alveolar overdistension by reducing tidal volume has been investigated in combination with relatively low levels of PEEP and high levels of PEEP. We describe them separately.
Four recent controlled studies13,30–32 have compared the outcome with use of low (6 to 7 mL/kg) versus high (10 to 12 mL/kg) tidal volumes with standard levels of PEEP (8 to 11 cm H2O). Only one study13 showed a significant (20%) decrease in mortality, likely because of a superior statistical power and a larger difference between the low-tidal-volume and high-tidal-volume branches. Use of low tidal volume, however, can worsen pulmonary gas exchange, necessitating higher FIO2 requirements, secondary to reduction in alveolar ventilation and increased shunt caused by both alveolar collapse and increased cardiac output, which may further worsen intra-pulmonary shunt in patients with ARDS.15
The induction of permissive hypercapnia by reducing tidal volume and maintaining constant standard levels of PEEP was shown to increase cardiac output, decrease PaO2, and increase shunt, but had no effect on the dispersion of blood flow (Log SD Q).33 When baseline tidal volume was reinstated and cardiac output was maintained with use of dobutamine, both shunt and PaO2 remained unchanged. Thus, low tidal volume with standard PEEP increased intrapulmonary shunt and the deterioration in gas exchange was caused by the combined effects of increased cardiac output and decreased alveolar ventilation. In another study,34 the effects of permissive hypercapnia were compared in two groups of patients with ARDS, with and without hyperdynamic sepsis. Permissive hypercapnia was induced by decreasing tidal volume and increasing PEEP to prevent alveolar recruitment. In both groups of patients, permissive hypercapnia increased shunt but did not worsen PaO2 because of an increase in mixed venous PO2 secondary to an increased cardiac output. In septic patients, PaO2 remained unchanged, and in nonseptic patients, it increased. Dead space and the descriptors of imbalance remained unchanged in both subsets of patients.
The combination of low tidal volume, to avoid alveolar overdistension, and high PEEP, to prevent sequential collapse and reopening of alveolar units throughout the ventilatory cycle, has been advocated as an alternative strategy to decrease ventilator-associated lung injury. In patients with ARDS,35 this approach achieved a significant reduction in mortality at 4 weeks, but hospital mortality was not decreased. The protective ventilation group had a higher rate of weaning from mechanical ventilation and a lower rate of barotrauma. This type of permissive hypercapnia produced an acute hyperdynamic state,36 with transient systemic vasodilation and increased cardiac output. The acute hemodynamic effects were in part related to acute respiratory acidosis, and progressively attenuated during the first 36 hours of ventilation despite persisting hypercapnia. The gas-exchange response to this ventilator strategy37 revealed superior efficacy in early ARDS (Fig. 37-7), mainly through recruitment of previously collapsed alveoli and redistribution of pulmonary blood flow from unventilated alveolar units to normal units. Arterial PO2 improved and intrapulmonary shunt decreased. Recruitment of previously collapsed alveolar units was the key factor underlying the decreased intrapulmonary shunt. The beneficial effects of this approach on gas exchange fully overcame the deleterious effect of permissive hypercapnia on arterial oxygenation (i.e., reduced alveolar PO2). Moreover, the increase in cardiac output secondary to systemic vasodilatation36 did not result in a proportional increase in intrapulmonary shunt, as one might expect in patients with ARDS,15 likely because pulmonary blood flow was appropriately redistributed to normal alveolar units secondary to the concomitant efficiency of alveolar recruitment (Fig. 37-8). The substantial improvement in
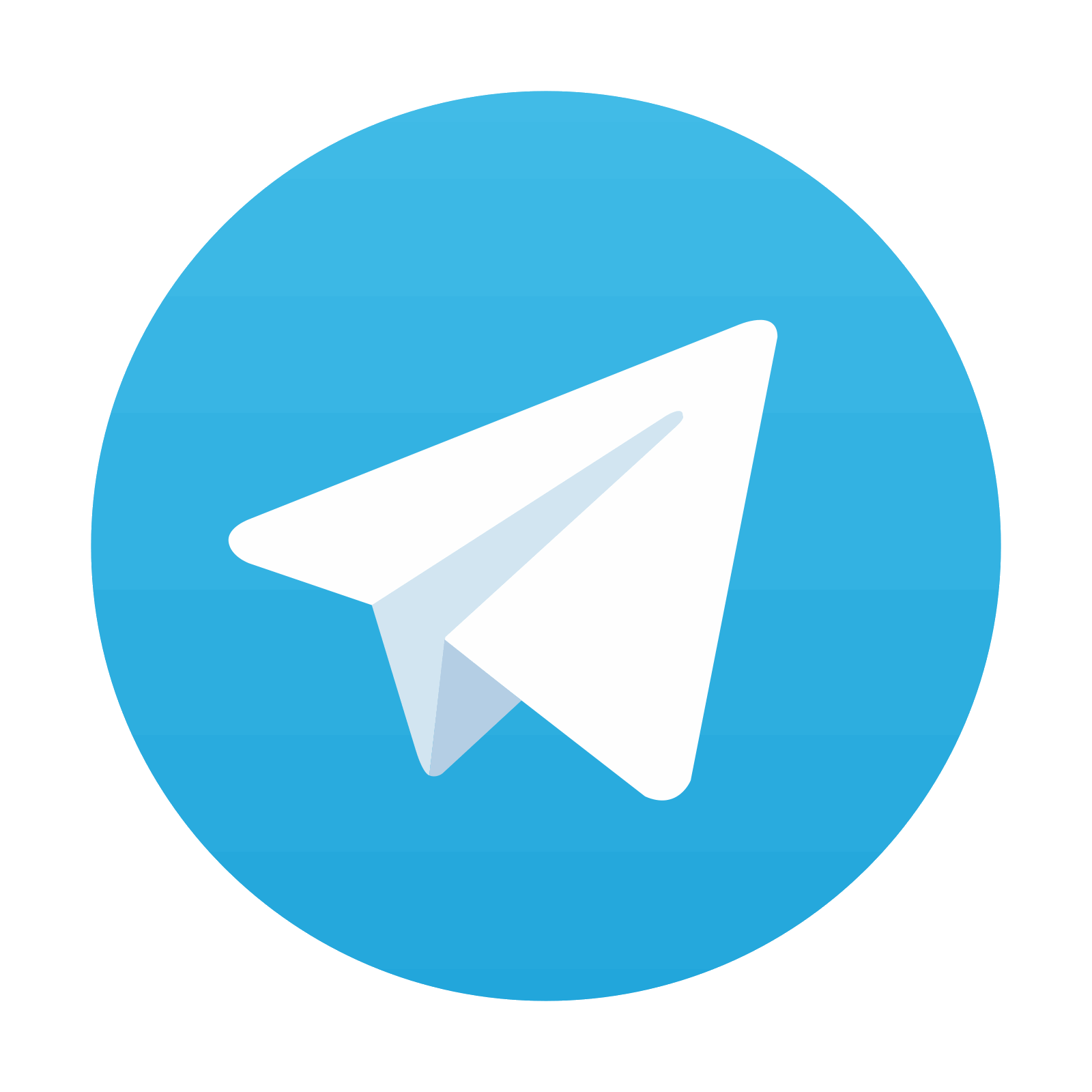
Stay updated, free articles. Join our Telegram channel
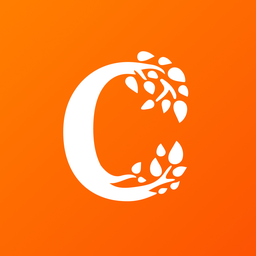
Full access? Get Clinical Tree
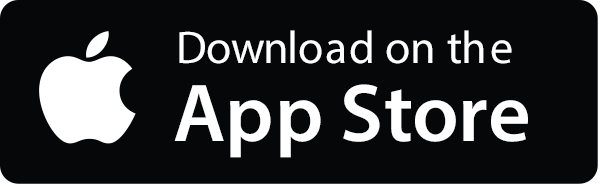
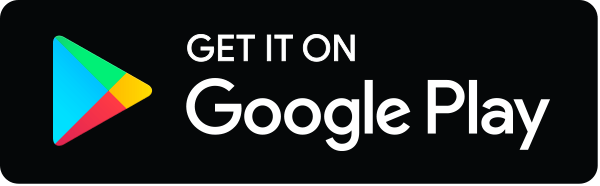
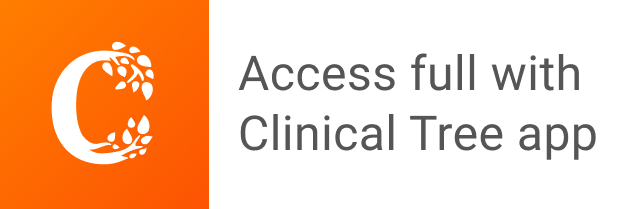