- •
Like all organisms, humans have evolved in concert with microbes that serve numerous physiologic and immune functions during normal development and homeostasis.
- •
Critical illness in both children and adults has been associated with profound changes in the microbiome at numerous body sites.
- •
The short- and long-term clinical consequences of these changes in the microbiome have not been clearly elucidated.
- •
Despite huge leaps in our understanding of host microbiome interactions during health and disease, the human microbiome’s diagnostic, prognostic, and therapeutic potential is yet to be realized.
The term microbiome was first used to describe the collective genome of a microbial ecosystem in 2001. , By 2007, the National Institutes of Health (NIH) had launched the first phase of the Human Microbiome Project (HMP) with the objective of describing the bacterial communities of healthy individuals. , Although these initial studies showed substantial interindividual variation at lower phylogenetic levels, such as genus and species, the phyla Bacteroidetes and Firmicutes clearly emerged as dominant in the healthy gut, and Actinobacteria and Firmicutes were shown to be the dominant skin phyla. The clear dominance of a limited set of phyla (and the absence of most others) colonizing human body niches highlights the long nonrandom coevolution between humans and bacteria.
Interestingly, the wide taxonomic variation seen in the gut microbiome of healthy individuals disappears at the gene level. Even during the unstable period of infancy, gut microbes maintain a relatively constant abundance of genes that encode for specific metabolic pathways. This genetic “core” can be attained by different combinations of taxa, which explains why different taxonomic configurations are all compatible with health and the consistent association between taxonomic diversity and a healthy microbiome. The important insight provided by genomic studies was that the functional diversity that allows gut microbes to adapt to environmental, physiologic, and nutritional changes is found at the genomic level, not the taxonomic level. The collective genome of the community, known as a metagenome , is what maintains ecologic stability and appropriate nutrient cycling. ,
At present, the study of microbiome science is moving from correlation to causation. The current approach to microbiome research integrates taxonomic, genomic, proteomic, physiologic, and metabolic data to allow contextualization of microbial communities, mechanistic descriptions, and biomarker identification. In 2014, the second phase of the HMP, known as the integrative HMP (iHMP), was launched by the NIH with the goal of describing the microbiome in pregnancy, inflammatory bowel disease (IBD), and type 2 diabetes—three important conditions linked previously to aberrant patterns of microbial colonization. The initial results of the iHMP have already refined and expanded our understanding of host-microbe relationships in health and disease, moving microbiome science further from correlation and closer to causation.
General concepts in the field of microbiome science
Commensal, pathogenic, and keystone species
The term commensal describes an ecologic relation between two organisms in which the commensal benefits and the host is neither benefited nor harmed. Our understanding of the human microbiome suggests that bacteria have a mutualistic relationship with us rather than a commensal one. That is, both the bacteria and their human host benefit from the relationship. On the other hand, pathogenic species are those that, when present in a host, can cause disease. However, many studies show that these distinctions are fluid in the context of the human microbiome, where nutrient availability, presence of pathogenicity islands, and inflammation can turn mutualists into pathogens. , Another important concept is that of keystone species , which are “highly connected taxa that individually or in a guild exert a considerable influence on microbiome structure and functioning irrespective of their abundance across space and time.”
Site specificity
The human body provides microorganisms with hugely divergent ecosystems to colonize. These ecosystems range from the anaerobic and nutrient-rich gut lumen to the aerobic but nutrient-depleted stratified squamous epithelia of the skin. The different habitats select for different microbes leading to site specificity , which is the well-described observation that microbial community membership varies predictably depending on the sampled body location. , , Although not yet proven, it has been postulated that loss of site specificity may portend worse outcomes in both critically ill children and adults. ,
Dysbiosis
The term dysbiosis has been loosely applied in the literature to any deviation from a healthy microbiome, especially when this deviation is associated with disease. , , These shifts have been associated with changes in diet, disease states, antibiotic use, surgical trauma, and many other insults. In the context of critical illness, a useful definition of dysbiosis is “a state of microbial organ dysfunction, a condition in which the gut-associated microbial community becomes a liability because the host no longer maintains proper control over the ecosystem.” , This dysfunction is most consistently associated with a shift from obligate to facultative anaerobes in the gut.
Although many ecologic perturbations are transient, the dysbiotic state persists when an insult is chronic, such as in IBD, or severe enough in acute conditions to cause a loss of keystone species . This persistent dysbiosis is further exacerbated by physiologic insults accompanying acute critical illness that increase expression of virulence factors even among commensal organisms. , The persistence of these dysfunctional communities has been associated with immunologic dysfunction, increased inflammation, and increased epithelial permeability, suggesting a role for the microbiome as both a cause and/or a consequence of critical illness ( Fig. 102.1 ). As discussed later, it is not yet known whether intensive care unit (ICU)–related dysbiosis resolves over time in patients who experience clinical improvement and whether microbiome-targeted therapies to reverse dysbiosis can hasten patient recovery or improve outcomes.

This chapter focuses on the bacterial component of the human microbiome. However, it is important to note that the human microbiome also consists of viral, fungal, and protozoan communities that are currently harder to study for technical reasons. , For example, study of the human virome, which includes viruses that infect human cells as well as other microbes within the body (e.g., bacteriophages), is limited because (1) viruses do not contain a conserved genomic region, such as the 16S gene in bacteria; and (2) many viruses are not included in viral databases. Newer techniques (see later discussion on metagenomic sequencing), although more expensive than amplicon sequencing, have overcome several barriers to studying nonbacterial communities in the human microbiome and revealed increasingly complex interactions within the microbiome and with the host in healthy and diseased states.
Studying the microbiome
Historically, clinical microbiology has relied heavily on the ability to cultivate pathogens from patient samples sent to clinical microbiology laboratories. This approach works well for a sample such as cerebrospinal fluid, which should be sterile but in some circumstances may contain a heavy burden of a single causative pathogen. However, this approach is less effective in characterizing mixed communities of organisms—especially in samples such as stool or sputum, with high bacterial density even in the absence of infection. One reason for this problem is that many or most organisms within the human microbiome cannot be cultivated for technical reasons. This may change with time with new knowledge about the metabolic and nutritional requirements of human-associated microbes. It is important for clinicians and scientists alike to realize that culture-dependent and culture-independent studies of the microbiota each have advantages and disadvantages.
The ability to complement or bypass laboratory culture in the study of the human microbiome has improved dramatically as a result of three recent scientific advances: high-throughput nucleic acid sequencing, the use of gene sequences encoding the 16S ribosomal subunit to enable taxonomic assignments, and the development of robust bioinformatic methods to analyze and interpret these results. Similar to -omics studies of the human genome, culture-independent studies of the microbiome can be categorized into several categories depending on the input variables of the experiment ( Table 102.1 ), for example, DNA or RNA.
Data Type | (Meta)genomics | Meta(proteomics), Meta(transcriptomics) | Metabolomics | Nutrition |
---|---|---|---|---|
Input data | 16S rRNA data Metagenomic reads Host genetics |
|
|
|
Affected constraints | Microbiome composition and relative abundances Host phenotype | Active metabolic pathways in individual microbes and host reconstruction |
| Input metabolites |
Bacterial profiling of microbiome samples is commonly based on analysis of a 1.5-Kbp gene that encodes the subunit of bacterial ribosomes. The nine hypervariable regions (V1–V9) of this gene are highly conserved among bacterial phyla but variable at lower taxonomic levels, making it an effective phylogenetic marker. , 16S rRNA gene sequencing results typically contain genes from numerous organisms, which can be identified using reference databases such as the Ribosomal Database Project (myRDP) or Silva. This process is called closed reference operational taxonomic unit picking. , In the case of the human gut microbiome, for example, extensive reference databases exist that are reliable and provide good specificity and sensitivity. In samples without extensive reference databases, such as respiratory samples, more challenging bioinformatic methods may be required to identify the bacterial composition present within the samples. ,
Once taxonomic assignments have been made, diversity measures can be calculated. The most commonly used diversity measures are alpha diversity and beta diversity . Alpha diversity measures the number of taxa within an environment, while beta diversity quantifies differences between environments. In other words, alpha diversity describes a single population, while beta diversity describes two or more populations. In summary, the steps of a typical taxonomic analysis are extraction of DNA from a sample (stool, saliva, etc.), targeted or untargeted (see later discussion) sequencing of microbial DNA, taxonomy assignment, and community analyses of diversity.
Whole-genome sequencing, or metagenomics, is another commonly used technique to study the microbiome but is more costly and labor intensive. This technique consists of sequencing and characterizing all of the microbial DNA present within a sample. Whereas 16S rRNA gene sequences are effective in profiling community membership, metagenomic sequencing studies are unbiased analyses of all genetic information present within microbial communities. In theory, metagenomic studies are not restricted to bacteria and offer the potential not only to identify which organisms are present but also to catalog all genes present, to predict their metabolic or virulence potential, and, in some cases, assemble entire microbial genomes. ,
Complementary -omics studies build on studies of DNA content to characterize the RNA, protein, and/or metabolites present within a given sample.
Development of the microbiome in children
Prior to delivery, the fetus is considered to be sterile or near-sterile. Cesarean and vaginal delivery each expose the newborn to a significant burden of bacteria; predominantly skin and oral flora in the case of cesarean delivery and vaginal and intestinal flora such as Enterobacteriaceae and Bacteroidaceae in the case of vaginal delivery. Controversy exists as to whether the mode of delivery confers lasting changes in bacterial colonization. However, no functional differences of the microbiome have been observed between infants born by vaginal or cesarean delivery. Recent evidence describing amniotic, placental, and meconium microbiomes suggests that maternal-fetal microbiota transfer may also occur.
Site specificity of the microbiome has been observed at as early as 6 weeks of life. At this age, stool, oral, and skin microbiota cluster distinctly and are enriched with characteristic microbiota—for example, Streptococcus in oral samples, Staphylococcus and Corynebacterium in skin samples, and Bacteroides in stool samples. Diet significantly influences the development of the infant microbiome. Multiple studies have demonstrated differences in gut microbiota between breastfed and formula-fed infants. Breastfed infants receive significant bacterial exposure from breastmilk, which contains high quantities of Lactobacillus and Bifidobacterium spp. These bacteria are able to synthesize compounds with antimicrobial effects against pathogenic bacteria and which assist digestion of human milk. Formula-fed infants have a higher prevalence of Clostridium and Roseburia spp. in their gut and overall have reduced alpha- and beta-diversity relative to breastfed children.
Following the introduction of cereals and other solid foods, a rapid expansion of diversity occurs in the infant gut with an increase in relative abundance of obligate anaerobic bacteria, including Bacteroidetes and Firmicutes . By approximately 2 to 3 years of age, the microbiome of children resembles that of adults and remains relatively stable over time. A disrupted microbiome early in life (during a so-called critical window ) has been linked to abnormal development of the immune system, risk of obesity, and atopic diseases, including asthma ( Fig. 102.2 ).

Development of intensive care unit dysbiosis in children
Following the launch of the HMP, most initial studies investigating links between dysbiosis and disease focused on outpatients with chronic diseases. As also seen in adults, children with chronic medical conditions can harbor different microbiota in their mouth, colon, and skin relative to healthy controls. For example, patients dependent on enteral tube feeds have been found to be depleted of commensal microbiota and enriched with pathogenic bacteria. A well-studied topic is the dysbiosis observed in children undergoing hematopoietic stem cell transplant (HSCT). Studies are beginning to describe the impact of these changes. In addition to the risk of infection, HSCT patients with dysbiosis may be more likely to develop graft-versus-host disease.
In recent years, studies of the microbiome and disease have been extended to critical illness, where alterations in the microbiome are particularly susceptible to rapid temporal variation. Disruptions to the microbiome in critical illness are likely unavoidable due to a combination of factors related to the host, illness, ICU environment, and ICU therapies. Preclinical models of critical care—which include starvation, preoperative antibiotics, and abdominal surgery—reliably cause severe dysbiosis. A landmark article recently demonstrated that many classes of medications—including proton pump inhibitors, antipsychotics, and calcium channel blockers—have unanticipated antimicrobial effects. A list of ICU factors affecting the microbiome is presented in Table 102.2 .
Factor | References |
---|---|
Intensive care unit environment | 22, 63–66 |
Sedation | 67 |
Acid suppression (proton pump inhibitor and histamine H2 receptor antagonist) | 62, 68, 69 |
Catecholamines | 70 |
Antibiotic therapy | 71–75 |
Intubation | 76–79 |
Enteral nutrition | 59, 80 |
Parenteral nutrition | 81, 82 |
Head position | 83, 84 |
Selective decontamination of the digestive tract | 85 |
Vascular catheterization | 86, 87 |
Older culture-dependent microbiome studies in the ICU, , which focused narrowly on specific pathogens such as S. aureus and P. aeruginosa , have reproducibly shown that the risk of colonization with pathogens increases over time in the ICU and that such colonization is associated with risk of a hospital-acquired infection. More recently, the application of culture-independent techniques to longitudinal studies of oral, tracheal, skin, and rectal samples from critically ill children have identified changes in multiple pathogenic organisms as well as healthy commensal bacteria over time in the pediatric intensive care unit (PICU). In one study, which enrolled a population with 95% of patients requiring mechanical ventilation, samples were collected within 48 hours of admission and then every 3 to 4 days and compared to a healthy volunteer database and children admitted for minor trauma. The findings of this study showed that children admitted to the PICU rapidly develop (1) loss of diversity, (2) loss of body-site specificity, and (3) presence of pathogens at high levels. Perhaps equally important, critical illness was characterized by a depletion of commensal microorganisms, such as Ruminococcus from the gastrointestinal tract and Rothia and Haemophilus from the mouth. These results are similar to those seen in studies of the microbiome in critically ill adults.
The natural history of ICU dysbiosis is not yet known. According to some reports, ICU dysbiosis appears to improve but not completely resolve during hospitalization. For example, in a 2-year old chronically ill child admitted with culture-positive sepsis caused by Enterococcus , enterococcal populations at three body sites disappeared with time and initiation of appropriate antibiotics. By day 15, the skin and oral communities had adopted a more healthy configuration. However, there is little if any long-term data assessing the microbiota of ICU patients months or years after hospital discharge. It seems likely that the resilience of an individual’s microbiome—that is, whether the microbiome ultimately returns to its baseline configuration—impacts the risk of adverse outcomes, including hospital readmission. The resilience of the gut microbiome has been characterized in the context of antibiotic treatment for healthy volunteers but has not been characterized after ICU discharge.
Known and potential clinical consequences of intensive care unit dysbiosis
Studies in the past several years have succeeded in characterizing temporal and spatial changes in the microbiome during critical illness. However, the correlations between dysbiosis and clinical outcomes are unclear. To date, the available data does strongly suggest that aberrant colonization profiles predispose patients to bloodstream infections, ventilator-associated pneumonia (VAP), and urinary infections. The gut of patients in the neonatal ICU (NICU) are frequently colonized by bacterial communities present on room surfaces touched by caregivers. These frequently contain pathogenic bacteria, including E. faecalis and K. pneumonia . , Disruptions in the normal colonization of infants, rather than growth of a single pathogen, has been associated with necrotizing enterocolitis and late-onset sepsis in neonates.
In acute respiratory distress syndrome (ARDS), recent research using culture-independent techniques has begun to characterize changes in the lung microbiome. A study of adults with sepsis and ARDS observed that the lung microbiome was enriched with bacteria from the gastrointestinal tract, including Bacteroides spp., and correlated with the degree of inflammation as determined by measurement of cytokines in bronchoalveolar lavage fluid. Research is ongoing to determine whether translocation of enteric bacteria to the lung precedes the development of ARDS. Intubated trauma patients were found to have significant loss of diversity over the initial 48 hours of mechanical ventilation and that community characteristics at 48 hours was associated with the subsequent risk of ARDS. A positive feedback mechanism that has been proposed for development of pneumonia and ARDS is shown in Fig. 102.3 . As depicted, local inflammation and nutrient supply inhibit further bacterial growth under normal conditions. However, in cases in which local inflammation causes sufficient endothelial and epithelial injury to allow leakage of protein-rich fluid into the alveolar space, the nutrient abundance may be restored, leading to development of pneumonia and collapse of the commensal microbial communities, in turn, leading to growth of a dominant pathogen in a “low-diversity high-biomass” state. Future studies should apply culture-independent methods of microbiome analysis to mechanically ventilated children and determine whether the lung microbiome can be targeted directly or indirectly via the gut to prevent the development of ARDS or to reduce symptoms in patients with established ARDS.
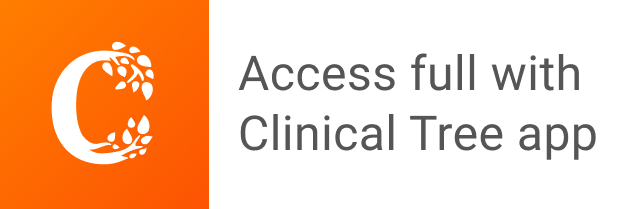