Abstract
The ideal operating conditions to enable cardiac surgery are a bloodless and motionless field. This requires both cardiac arrest and the drainage of blood from the heart. In order to provide these conditions a separate means of maintaining nutrient supply to the rest of the body is needed. In addition, the heart must be prevented from becoming sufficiently ischaemic as to infarct previously viable muscle.
Introduction
The ideal operating conditions to enable cardiac surgery are a bloodless and motionless field. This requires both cardiac arrest and the drainage of blood from the heart. In order to provide these conditions a separate means of maintaining nutrient supply to the rest of the body is needed. In addition, the heart must be prevented from becoming sufficiently ischaemic as to infarct previously viable muscle. The two core tenets, therefore, of CPB are:
1. Protect the heart (myocardial protection).
2. Protect the patient (preservation of the patient brain, kidneys and other vital organs while the heart is stopped).
Myocardial Protection
Determinants of Myocardial Supply and Demand
Under normal circumstances myocardial oxygen supply is determined by blood flow and blood oxygen content. Blood flow is determined by Ohm’s law, such that flow is directly proportional to the driving pressure gradient and inversely proportional to the resistance to flow.
In the LV, intramyocardial pressure during systole limits coronary blood flow, such that the majority of flow occurs during diastole. The effect is that the oxygen delivery is significantly reduced during periods of tachycardia as a result of a reduced diastolic time. Increased resistance may also be encountered as a result of ventricular hypertrophy, reduced coronary artery calibre (which may be affected by extrinsic and intrinsic regulation) and increased blood viscosity (Poiseuille’s law). Blood oxygen content is determined by Hb concentration, oxygen saturation and (to a very small extent) dissolved oxygen.
Myocardial oxygen demand is determined by the HR, contractility and wall tension – a ‘stretched’ heart will consume more oxygen. In addition to these factors there is a basal metabolic demand, even when the heart is arrested.
Optimizing Supply and Demand
In the initial phase of CPB, blood is still supplied by the coronary arteries and the mechanical pump replacing the heart reduces myocardial work. However, in order to provide a bloodless and motionless field, the blood supply must be interrupted, and the heart stopped. Minimal energy consumption occurs in diastolic arrest. The heart subsequently only requires basal metabolic demands to be met. The prevention of ventricular dilation using a LV vent may also reduce oxygen demand. Basal cellular metabolic demand may be further reduced using hypothermia. Both cardiac arrest and the supply of metabolic substrate are facilitated using a ‘cardioplegia’ solution. Cold cardioplegia can be used with external cardiac cooling to further reduce myocardial oxygen demand (Figure 5.1).
Figure 5.1 Temperature versus oxygen consumption with varying myocardial activity
While it is also possible to achieve a relatively motionless field by inducing VF, in combination with intermittent cross-clamping and moderate hypothermia, the electrical activity results in a higher oxygen requirement and may result in significant myocardial ischaemia. Cross-clamp/fibrillation is uncommon in contemporary practice.
Myocardial Ischaemia and Injury
Myocardial Hibernation and Stunning
Interruption of myocardial blood supply will eventually cause cardiomyocyte death and MI. However, when the interruption is shorter, periods of ischaemia may result in reduced mechanical function that recovers over minutes to hours. This depression in myocardial function has been termed ‘stunning’. In general, the period of stunning lasts significantly longer than the original ischaemic insult. When the limitation of coronary blood flow is chronic, but insufficient to cause infarction, myocardial hibernation may occur. Function may be restored by inotropic support or restoration of blood flow. The former helps distinction between non-viable and viable tissue that may recover after revascularization.
Ischaemia Reperfusion Injury
Perfusion of a previously ischaemic region of myocardium can lead to significant injury over and above the injury caused by the original insult. This occurs after surgery as well as percutaneous intervention. Clinical presentations include arrhythmias, myocardial stunning, microvascular obstruction and lethal myocardial reperfusion. Myocardial injury after cardiac surgery is likely to be multifactorial, relating to myocardial handling, perioperative inflammation and arterial manipulation, as well as classical ischaemia reperfusion injury (IRI) itself. Mechanisms of IRI relate to cellular calcium overload, oxidative stress, rapid pH change and opening of a transmembrane pore with irreversible cardiomyocyte hypercontracture and cell death.
Ischaemic Conditioning
Ischaemic conditioning refers to the process of rendering myocardium less vulnerable to ischaemic injury – essentially another form of cardioprotection. It was first observed more than 30 years ago when it was noted that intermittent occlusion of the LAD artery for four 5-minute periods before a prolonged period of occlusion was associated with reduced infarct size in dogs. This phenomenon became known as ‘ischaemic preconditioning’ (IPC) and appeared to be an endogenous method of preserving myocardium in response to ischaemia. Since then, it has been shown there are two ‘windows’ of myocardial protection after IPC. The first occurs 2–3 hours after IPC and is known as ‘classical IPC’, the second occurs after 48–72 hours and is known as ‘delayed IPC’ (Figure 5.2). The cellular mechanisms underlying IPC have not been fully elucidated.
Figure 5.2 Types and timing of ischaemic conditioning in relation to the original ischaemic and reperfusion episode. Dark shading represents clinical situations in which conditioning has been tested, while light shading represents potential conditioning applications.
One of the challenges in IPC is that myocardial events occur without prior warning, such that by the time they have occurred it is too late to institute IPC. In addition, in the case of cardiac surgery, clamping and unclamping of the aorta in order to provide IPC may have significant adverse thromboembolic effects. The process of intermittent reperfusion of an ischaemic territory before complete reperfusion has been termed ischaemic postconditioning and is most feasible in the cardiac catheter laboratory. However, despite promising early trials, later trials showed mixed effects and the results of the largest randomized trials have failed to demonstrate benefit. Given these findings and the increased risk of thromboembolism with external arterial occlusion it seems unlikely that postconditioning will be useful in adult cardiac surgery.
The application of ischaemic conditioning stimulus to the heart itself limits the application and feasibility of IPC. However, there is evidence that application of an ischaemic stimulus remote to the heart can induce cardioprotection. This so-called remote ischaemic preconditioning (RIPC) is most commonly induced by using an inflatable tourniquet to produce 5–20 minutes of limb ischaemia. Like IPC, the mechanisms underlying RIPC are only partially understood, although it appears that both neural and humoral components are required. The relative simplicity of the technique has led to a number of large trials in cardiac surgery; however, to date no large trial has demonstrated efficacy.
Pharmacological Preconditioning
Arguably, the most attractive form of preconditioning would use a pharmacological agent to activate myocardial protection. Volatile agents were found to reduce IRI in animal models in the 1970s and 1980s. The proposed mechanisms of cardioprotection share IPC pathways. Multiple proof-of-concept clinical studies have shown reductions in surrogate markers of myocardial ischaemia (e.g. troponin) using volatile anaesthesia versus propofol and/or midazolam. Retrospective studies have suggested a reduction in mortality with volatile anaesthesia and in a recent meta-analysis mortality was halved. However, all the studies were small, and, given the low conversion rate for positive meta-analyses when repeated in large randomized trials, this result should be taken with a degree of scepticism.
Cardioplegia
Cardioplegia refers to a range of solutions designed to induce temporary diastolic cardiac arrest. In addition to the chemical induction of cardiac arrest, cardioplegia may also be used to induce myocardial hypothermia and provide additional protection using buffers, metabolic substrates and other pharmacological agents. Most cardioplegia solutions use a concentrated K+ solution (15–20 mmol l–1) with approximately physiological concentrations of other electrolytes to maintain isotonicity. The hyperkalaemia results in a less negative membrane potential, maintaining cell depolarization. This is known as extracellular cardioplegia. Alternatively, a solution with very low Na+ content (~15 mmol l–1) may be used to hyperpolarize the membrane and prevent depolarization. This is known as intracellular cardioplegia. There is little evidence to support one cardioplegia type over the other.
Additives may be used to provide cellular nutrition or reduced energy consumption and cellular damage. The most common additive used is blood. There is reasonable evidence that the addition of blood to cardioplegia provides superior myocardial protection versus crystalloid solution alone. Blood is usually added to crystalloid in a 4:1 ratio (4 parts blood: 1 part crystalloid) with final electrolyte concentrations similar to those described above. Other additives used include antiarrhythmic agents, calcium channel blockers, β-blockers, amino acids and bicarbonate. There is little high-quality evidence to support or refute their use. Cardioplegia may be delivered cold (<20 °C) or warm (21–37 °C). Of studies that have examined the question of warm versus cold cardioplegia many are small and of poor quality. Meta-analysis of these studies found no difference in major adverse cardiac events, although some indices of myocardial function and injury were improved in the warm cardioplegia group. Where cold cardioplegia is used, a ‘hot-shot’ of warm cardioplegia is sometimes given before removal of the AXC and myocardial reperfusion. Small studies have suggested that this may reduce myocardial injury on reperfusion.
Cardioplegia may be delivered via the coronary arteries (antegrade) or via the venous system using the coronary sinus (retrograde). Most commonly, the antegrade cardioplegia is delivered using a cannula placed between the AXC and the heart (Figure 5.3A). A pressure of 80–100 mmHg is used, and providing the AV is competent, all flow will be delivered into the coronary arteries. In the case of an incompetent or open aorta, cardioplegia can be delivered by direct coronary ostial cannulation. Alternatively, a balloon-tipped cannula can be placed in the coronary sinus to deliver blood via the venous system. This may be done ‘blind’ or guided using TOE. Delivery pressures are lower to avoid damage to the sinus. The theoretical advantage of retrograde cardioplegia is protection of myocardium rendered more vulnerable by coronary artery disease and ventricular hypertrophy. However, a significant proportion of the retrograde blood is lost to the Thebesian system (requiring larger cardioplegia volumes to be given), and RV protection may be inferior. In practice, a combined retrograde and anterograde approach may provide the best protection (Figure 5.3B).
(A) Antegrade cardioplegia cannula with side-port to allow function as a vent. From Buckberg GD, Todd RJ. Patent US 5013296 A. May 1991.
(B) Retrograde cardioplegia cannula with rigid, curved introducer to allow placement in coronary sinus, balloon-tip for secure placement and side-port for continuous pressure monitoring. From Bicakci M, Higgins SW. Patent US 6500145 B1. December 2002.
The Cardiopulmonary Bypass Circuit
The CPB circuit provides protection for the patient by maintaining blood flow to the rest of the body while the heart is stopped. The key components of CPB are: a venous drainage line, a reservoir for drained blood, a pump, a heat exchanger and oxygenator and an arterial line to return blood to the patient. However, modern CPB machines include many more components. A schematic diagram is shown in Figure 5.4.
Figure 5.4 Schematic diagram of CPB machine components and connections
Components
Tubing
Tubing is required to connect the components of the system to one another. Flow characteristics are determined by the length and width of the tubing (Poiseille’s law). Larger-diameter pipes allow higher flow rates but carry the disadvantage of a higher prime volume and greater haemodilution. A variety of materials are available, including polyvinyl chloride (PVC), silicone and rubber. PVC has the advantages of relative strength and flexibility (although flexibility is reduced with lower temperatures), and is used in the majority of the circuit. PVC can be bonded with heparin to reduce the occurrence of clot formation in the circuit. Silicone may be used in the roller pumps due to a reduced tendency to cause haemolysis; however, prolonged use may cause spallation (release of silicone into the lumen).
Arterial Cannula
Blood is returned to the patient via an arterial cannula, usually placed in the ascending aorta (an easily accessible location at sternotomy with a low incidence of cannulation-related injury). Other common sites of return include the femoral, subclavian and innominate arteries. The arterial cannula should be designed to provide optimal blood flow, minimize arterial wall trauma and be easily secured. The flow properties of the numerous different types of cannulae available can be compared using their performance index, which is defined as the pressure gradient versus the outer diameter at any given flow. A variety of cannula designs are available (Figure 5.5).
Figure 5.5 Arterial cannulae
Venous Cannula
The venous cannulae drain blood from the heart into the venous reservoir. While they can be placed peripherally they are most commonly placed centrally. A two-stage cannula may be inserted via the RA into the IVC. The tip drains blood from the IVC while proximal holes drain blood from the SVC (Figure 5.6). Alternatively, single-stage cannulae can be inserted into each of the IVC and SVC, allowing complete drainage of venous return and better access for open-heart valvular procedures. Flow of blood into the CPB circuit is achieved by gravity-driven (siphon) drainage or by using vacuum-assisted drainage. Siphoning venous blood relies on air-free tubing to avoid accumulated air stopping drainage (“air lock”) and on the bypass reservoir being placed below the level of the patient’s thorax. Venous drainage is largely determined by the patient’s CVP, the height difference between the thorax and the reservoir and the diameter of the venous cannula.
Figure 5.6 (A) Y-piece for connecting two single-stage cannulae; (B) single-stage cannula; (C) two-stage cannula
Venous Reservoir
The venous reservoir receives blood from the venous lines. It allows a margin of safety by maintaining a level of fluid in the reservoir when venous drainage is temporarily impeded. Drainage from the lowermost part prevents any air in the venous line from entering the arterial side of the circuit. The majority of venous reservoirs are rigid and ‘open’. This allows easier filtering and addition of cardiotomy sucker blood (Figure 5.7). ‘Closed’ reservoirs are collapsible and tend to require more attentive management. They are commonly used in ‘mini-bypass’. A vacuum assist may be added to the drainage line to improve drainage, although this may increase the likelihood of air entrainment.
Figure 5.7 Rigid, ‘open’ venous reservoir
Pumps
Two types are in widespread use: roller pumps and centrifugal pumps. Roller pumps have been used since the early days of CPB and remain the most commonly used device. They typically consist of a rotating head with two rollers that compress the tubing mounted in a circular track (‘raceway’). Rotation generates a positive pressure after the roller and a negative pressure behind, resulting in forward flow of blood (Figure 5.8). Rapidly altering the rate of pump head rotation can produce pulsatile blood flow. While this is more ‘physiological’ than constant (non-pulsatile) flow, evidence of improved outcome is sparse. Roller pumps are robust, relatively cheap and largely insensitive to preload and afterload. Disadvantages include more haemolysis than centrifugal pumps, and the potential for pumping large volumes of air if entrained into the circuit. Sudden venous occlusion may result in ‘cavitation’ within the circuit (the formation of gas bubbles due to a reduction in pressure).
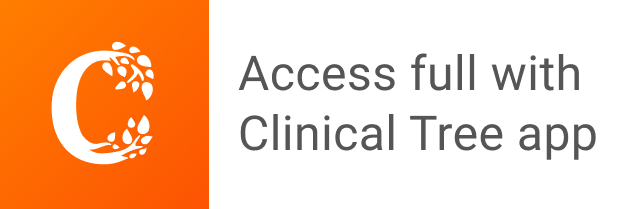