Abstract
Monitors of neurological function have been available since the 1950s. Until recently, however, many have remained in the hands of enthusiasts at academic centres. Many laboratory methods are not readily adaptable for intraoperative use or cannot be practically used routinely because of cost or the need for expert interpretation. The invasive nature of other monitors (e.g. cerebral microdialysis) precludes their use in the setting of systemic anticoagulation. Emerging evidence suggests that modern neuromonitoring technologies – particularly when used together – can be used both to predict and to modify clinical outcome.
Monitors of neurological function have been available since the 1950s. Until recently, however, many have remained in the hands of enthusiasts at academic centres. Many laboratory methods are not readily adaptable for intraoperative use or cannot be practically used routinely because of cost or the need for expert interpretation. The invasive nature of other monitors (e.g. cerebral microdialysis) precludes their use in the setting of systemic anticoagulation. Emerging evidence suggests that modern neuromonitoring technologies – particularly when used together – can be used both to predict and to modify clinical outcome.
Currently available technologies can be broadly classified as either monitors of the physiological milieu (cerebral perfusion and oxygen delivery) or monitors of neuronal function (Table 31.1). It should be borne in mind that neurological monitors complement, rather than replace, routine clinical monitoring of the MAP, CVP, SaO2, PaCO2, temperature, pupil size and the concentrations of Hb and glucose.
Table 31.1 Neurological monitoring during cardiac surgery
Physiological milieu (O2 delivery) | Neuronal function |
---|---|
|
|
rSO2, regional cerebral oxygen saturation; PTIO2, partial pressure of oxygen in brain tissue. Italics indicate research modalities not available for routine clinical use.
Neuronal Function
Neuronal function can be assessed using EEG (raw and processed) and evoked potential (sensory and motor) monitoring.
Electroencephalography
Measurement of cerebral cortical electrical activity is one of the oldest methods of neurophysiological monitoring. The EEG signal represents summated post-synaptic potentials (20–200 μV) of pyramidal neurones, typically measured simultaneously between several pairs of scalp electrodes (Figure 31.1).
Figure 31.1 The internationally standardized 10-20 system of EEG electrode placement. Four bony landmarks – the nasion, inion and preauricular points – act as a reference and the cranium is then apportioned into 10% or 20% segments between these landmarks, as originally described by Jasper. F, frontal; Fp, frontal polar; C, central; O, occipital; P, parietal; T, temporal; A, ear lobe; Pg, nasopharyngeal. Right-sided placements are indicated by even numbers, left-sided placements by odd numbers and midline placements by Z. Auricular (A1 and A2) positions complete the standard 21-electrode positions. The figure also identifies the anticipated locations of the Rolandic and Sylvian fissures, and commonly used additional Pg and cerebellar (Cb) electrodes. The location and nomenclature of these electrodes is standardized by the American Clinical Neurophysiology Society, formerly the American Electroencephalographic Society.
The EEG is described in terms of location, amplitude and frequency. Frequency is conventionally grouped into four bands: δ, θ, α and β (Table 31.2). A normal awake adult has a posteriorly located, symmetrical EEG frequency of around 9 Hz (i.e. α rhythm). Opioids and most anaesthetic agents produce a dose-dependent EEG slowing (↓ α and ↑ δ and θ) culminating in periods of very low EEG amplitude and burst suppression (periods of high-voltage electrical activity alternating with periods of silence). N2O induces high-frequency frontal activity and decreased amplitude. Ketamine increases the EEG amplitude at low doses and slows the EEG at higher doses.
Table 31.2 EEG waveforms
Waveform | Frequency (Hz) | Amplitude (μV) | Comments | |
---|---|---|---|---|
Delta (δ) | 1.5–3.5 | >50 | Normal during sleep and deep anaesthesia, indication of neuronal dysfunction | |
Theta (θ) | 3.6–7.5 | 20–50 | Normal in children and elderly, normal adults during sleep, produced by hypothermia | |
Alpha (α) | 7.6–12.5 | 20–50 | Awake, relaxed, eyes open, mainly over occiput | |
Beta (β) | 12.6–25 | <20 | Awake, alert, eyes open, mainly in parietal cortex, produced by barbiturates, benzodiazepines, phenytoin, alcohol | |
Gamma (γ) | 25.1–50 | <20 |
The EEG has long been regarded as the ‘gold standard’ for the detection of cerebral ischaemia. At constant temperature and depth of anaesthesia (DOA), progressive ischaemia produces a reduction in total power and slowing – decreased α and β power and increased δ and θ power. These changes only become apparent when the cerebral blood flow (CBF) halves (i.e. <50 ml 100 g–1 min–1). An EEG amplitude attenuation of <50% or increased δ power is regarded as being indicative of mild ischaemia, whereas >50% attenuation or a doubling in δ power is regarded as being indicative of severe ischaemia. An isoelectric or ‘silent’ EEG is seen when CBF < 7–15 ml 100 g–1 min–1. EEG changes are not specific for pathology.
Under conditions of constant DOA and autoregulated cerebral perfusion, the effects of hypothermia on the EEG can be used to guide temperature management in cases requiring deep hypothermic circulatory arrest (DHCA). The wide range of temperatures at which patients reach burst suppression (15.7–33.0 °C) and EEG silence (12.5–27.2 °C) suggests that the use of arbitrary DHCA temperatures (e.g. 20 °C) may be inappropriate for many patients (Figure 31.2). Temperature-induced EEG changes are not predicted by age, isoflurane concentration, PaCO2, or other physiological factors.
Figure 31.2 Intraoperative nasopharyngeal temperatures of 109 patients undergoing DHCA with EEG monitoring. The distribution of temperatures at which burst suppression (A) and EEG silence (B) were achieved.
Individualized temperature management may allow safe DHCA at higher temperatures, reducing the physiological burden of extreme hypothermia and CPB duration.
Depth of Anaesthesia
Although ubiquitous, routine clinical monitoring (e.g. MAP, HR, respiratory pattern, diaphoresis and pupil size) is a crude and imprecise measure of DOA. The use of more reliable monitors of DOA has been driven, at least in part, by the recognition that:
Pharmacological suppression of EEG activity does not mitigate against cerebral ischaemic injury
Anaesthetic agents may themselves be neurotoxic
There is a correlation between DOA and mortality
There may be a link between DOA and postoperative cognitive dysfunction
Using a 16-channel EEG montage to monitor DOA is fraught with problems:
Interpreting the EEG requires training and is time-consuming
There is no ‘gold standard’ for wakefulness, sedation, anaesthesia and deep anaesthesia
The EEG is altered by ischaemia, temperature and metabolism – as well as drugs
Currently available DOA monitors process the raw EEG obtained from electrodes placed on the forehead, to produce a time-averaged, proprietary number: typically, a dimensionless integer (e.g. 0 to 100). For the reasons stated above, both ketamine and N2O may paradoxically increase this index, suggesting an apparent decrease in DOA.
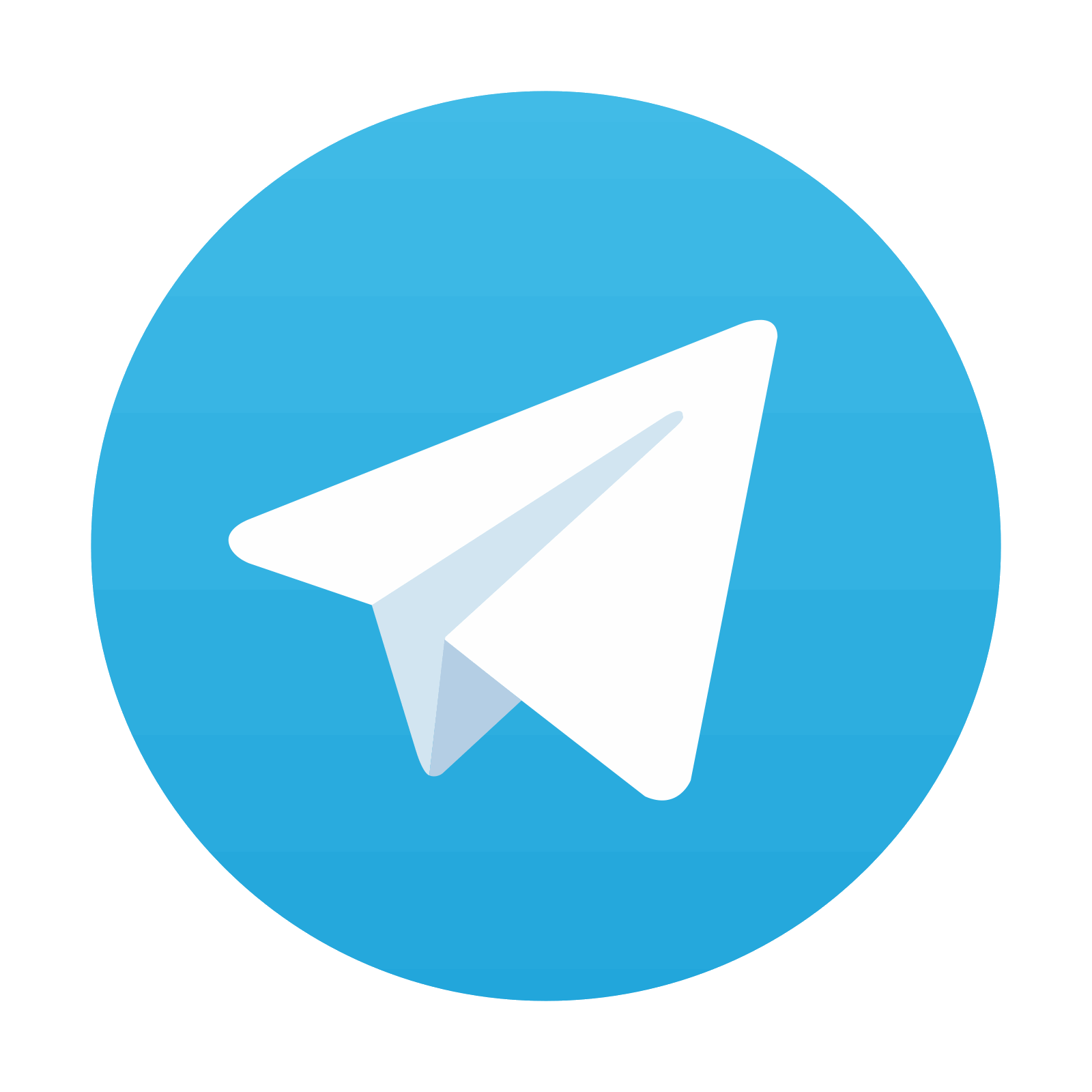
Stay updated, free articles. Join our Telegram channel
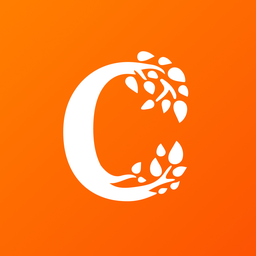
Full access? Get Clinical Tree
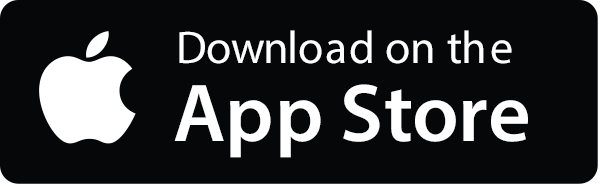
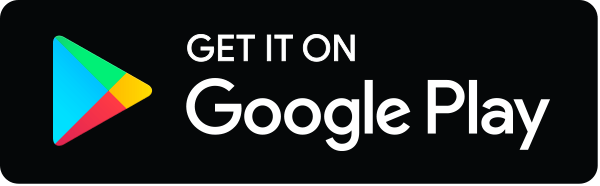
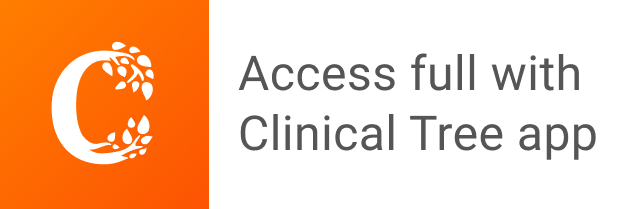