Pearls
- •
Cellular respiration is largely coordinated in the mitochondria and normally aligns energy demand with energy production. In critical illness, disruption of cellular bioenergetic homeostasis may be a final common pathway for organ dysfunction and death.
- •
Cellular respiration consists of three related series of biochemical reactions: (1) glycolysis of carbohydrates, β-oxidation of fatty acids, and catabolism of amino acids to produce acetyl-coenzyme A (acetyl-CoA); (2) metabolism of acetyl-CoA in the Krebs cycle to produce electron-rich nicotinamide dinucleotide (NADH) and flavin adenine dinucleotide (FADH 2 ); and (3) shuttling of electrons from NADH and FADH 2 along the electron transport system to oxygen in order to synthesize adenosine triphosphate (ATP) in the mitochondria.
- •
Shock is an imbalance between oxygen (and substrate) delivery and oxygen (and substrate) utilization such that cellular metabolic demands are not met. If oxygen delivery is not rapidly restored or appropriate oxygen utilization is not restored, ATP turnover will decrease, and an altered state of bioenergetic homeostasis can trigger cell injury and organ dysfunction. Persistent mitochondrial dysfunction may contribute to a clinical state of cytopathic hypoxia in which cellular respiration remains abnormal even despite restoration of oxygen delivery.
- •
Bedside measures of cellular respiration remain challenging. The two most commonly used measures, lactate and venous oxygen saturation, provide important information about global oxygen utilization and have proved to be useful to guide acute resuscitation, but they have important limitations. More direct measures of cellular respiration may be available in the near future.
- •
A therapeutic approach that better aligns oxygen (and substrate) utilization with oxygen (and substrate) delivery may help to restore bioenergetic homeostasis and improve cellular—and thus organ—function. Several existing and novel therapies may help to improve mitochondrial function in particular.
“In every one of us there is a living process of combustion going on very similar to that of a candle, and I must try to make that plain to you. For it is not merely true in a poetical sense.” —Michael Faraday, A Course of Six Lectures on the Chemical History of a Candle (1861)
In 1920, Haldane was credited with the observation that hypoxemia not only stops the (respiration) machine but wrecks the (respiration) machinery as well. Indeed, the priorities of pediatric advanced life support and cardiopulmonary resuscitation are to restore oxygen and substrate delivery to tissues and cells. Critical care extends these basic principles of restoration of oxygen and substrate delivery to also support oxygen utilization and cellular respiration with the goal to reestablish and maintain bioenergetic homeostasis.
Alterations in cellular respiration can contribute to a bioenergetic imbalance that results in cell and, ultimately, organ dysfunction. Oxygen availability and utilization are vital for cells to efficiently convert the chemical energy of nutrient molecules into useful energy in the form of adenosine triphosphate (ATP). This process involves a highly regulated network of enzymatic reactions, largely coordinated in the mitochondria, which normally closely align energy demand with energy production. In critical illness, this delicate homeostatic balance may be disrupted and has been identified as a possible final common pathway for organ failure and death. , For example, with improved biological understanding, the concept of septic shock has evolved from a state solely defined by cardiovascular dysfunction to now include cellular metabolic abnormalities. Consequently, a working knowledge of cellular respiration is a key tenet of critical care medicine.
This chapter reviews (1) major pathways of cellular respiration with a focus on the mitochondria; (2) the role of impaired cellular respiration in critical illness, particularly in shock and multiple-organ dysfunction syndrome (MODS); (3) clinical assessment of oxygen utilization and mitochondrial function; and (4) potential therapeutic strategies to improve mitochondrial respiration and restore bioenergetic homeostasis.
Pathways of cellular respiration
Plants harness the sun’s energy to split water into hydrogen and oxygen ( Fig. 79.1 ). The hydrogen is then attached to carbon to create glucose and starch, while the oxygen is released into the atmosphere. Animals, including humans, eat plants to acquire carbohydrates (as well as other animals to obtain protein and fat), ultimately removing the hydrogen and combining it with oxygen to regenerate water. Through this cyclical process, humans convert energy in the form of photons from the sun to usable cellular energy in the form of ATP through a process called oxidative phosphorylation . As will be discussed, an interconnected and alternative pathway of anaerobic respiration also exists within the cytoplasm. However, in the absence of oxygen, it is not sufficient to sustain human life.

Apart from erythrocytes, all human cells possess mitochondria. These organelles account for nearly all total body oxygen consumption and are responsible for more than 90% of ATP production. Although mitochondria participate in many cellular processes other than ATP production, this chapter focuses on their role as the bioenergetic powerhouse of the cell. An alternative end production of oxygen consumption is the formation of reactive oxygen species (ROS), which is also discussed. Mitochondrial involvement in cell signaling, heat production, calcium regulation, hormone synthesis, cell death pathways, and genomic/epigenomic expression are reviewed elsewhere. ,
A typical human adult male consumes approximately 380 L of oxygen per day, with well-conditioned athletes achieving rates up to 10 times higher. Oxygen serves as the final electron receptor at the terminal complex of the mitochondrial electron transport system (ETS). Through a series of oxidation-reduction (redox) reactions, electrons are transferred through the ETS to oxygen, pumping protons from the mitochondrial matrix to the intermembrane space, producing a proton motive force that is coupled to ATP production by ATP synthase (ETS complex V). Mitchell first described this chemiosmotic principle —the coupling of biological electron transfer to ATP synthesis—in the 1960s. It is now recognized that 3 × 10 21 protons per second are transferred across all of the inner mitochondrial membranes in an adult male producing ATP at a rate of 9 × 10 20 molecules per second. This is equivalent to a turnover rate of 65 kg of ATP per day, with even higher rates during periods of activity.
The phosphorylation of adenosine diphosphate (ADP) to form ATP through aerobic and, to a lesser extent, anaerobic processes provides the currency for cells to perform a wide range of energy-consuming activities, such as the active pumping of solutes against a concentration gradient across a membrane barrier. The first law of thermodynamics states that the total amount of energy in a system remains constant during a chemical reaction. The second law of thermodynamics states that, even though total energy does not change, the net amount of usable or free energy (termed Gibbs free energy and designated by the letter G) is always decreased—that is, a negative ΔG. Chemical reactions are characterized either as those that produce energy ( exergonic or ΔG <0) or those that consume energy ( endergonic or ΔG >0). In living organisms, the highly complex, ordered state of homeostasis would naturally degrade to a less complex disordered state (i.e., toward a negative overall ΔG) without continual cellular maintenance. By coupling energy-consuming endergonic processes to the hydrolysis of ATP to yield ADP and inorganic phosphate—an energetically favorable exergonic reaction—cells are able to drive forward critical chemical reactions that would otherwise not be possible.
Complete oxidation of nutrient fuels is accompanied by a large release of free energy that is used to produce ATP. In general, cellular respiration consists of three related series of biochemical reactions:
- •
Chemical reactions resulting in the formation of two-carbon acetyl-coenzyme A (acetyl-CoA) through glycolysis of carbohydrates, β-oxidation of fatty acids, and catabolism of amino acids
- •
Metabolism of acetyl-CoA to carbon dioxide in the Krebs cycle with generation of the electron-rich reducing equivalents nicotinamide dinucleotide (NADH) and flavin adenine dinucleotide (FADH 2 )
- •
Shuttling of electrons from the reducing equivalents in NADH and FADH 2 along the mitochondrial ETS, leading to ATP synthesis and ultimately reducing oxygen to water
Although the initial catabolic steps vary among the different fuels (e.g., carbohydrates, fats, and amino acids), all nutrient molecules eventually converge to a common mitochondrial pathway ( Fig. 79.2 ).

Glycolysis (anaerobic respiration)
Glucose is the principal metabolic substrate for glycolysis and the primary fuel for many organ systems, including the central nervous system. Glucose is transported into cells via glucose transporter (GLUT) receptors and down osmotic gradients. Once in the cell, glucose enters the glycolytic pathway and is rapidly phosphorylated by the enzyme hexokinase to glucose-6-phosphate. Consequently, cellular glucose concentrations are low, allowing for a substantial favorable osmotic gradient for glucose to enter cells.
Ten enzymatic reactions within the cell cytoplasm define the metabolic pathway of anaerobic respiration, termed glycolysis . The free energy extracted from glucose through glycolysis is used to synthesize two net molecules of ATP, two molecules of NADH, and two molecules of pyruvate. Under aerobic conditions (i.e., when oxygen is available), pyruvate is shuttled into the mitochondrial matrix where it is metabolized by pyruvate dehydrogenase (PDH) to acetyl-CoA and carbon dioxide (CO 2 ), and NAD + is converted to NADH. Thus, PDH is a key enzyme that links cytoplasmic glycolysis to mitochondrial respiration. Inhibition of PDH activity occurs with elevated NADH/NAD + , ATP/ADP, and acetyl-CoA/CoA ratios indicating an energy-replete state, helping to maintain bioenergetic homeostasis. Alternatively, under anaerobic conditions, pyruvate is reduced by NADH to lactate by lactate dehydrogenase (LDH) in order to regenerate the NAD + needed to continue glycolysis. During periods of hypoxemia, anaerobic respiration through glycolysis can be increased for a limited time to generate ATP in the absence of oxygen. However, glycolysis alone results in a net generation of only two ATP molecules compared with the much more efficient process (10–15 times more efficient) of oxidative phosphorylation described later.
Fatty acid β-oxidation
Fatty acids are primarily oxidized through β-oxidation in the mitochondrial matrix. Entry of long-chain free fatty acids into the mitochondria is dependent on the carnitine transport system, while medium-chain fatty acids and ketone bodies enter the mitochondria without carnitine. Catabolism of fatty acids by β-oxidation sequentially removes two-carbon units from the carboxyl terminal to generate one molecule of acetyl-CoA, one FADH 2 , and one NADH for each two-carbon fatty acid fragment cycle. Like carbohydrate metabolism through glycolysis, lipid metabolism is a tightly regulated process. Lipid stored in adipose tissue cycles continuously between triglycerides and free fatty acids. When glucose and insulin concentrations are high (e.g., after a meal), fatty acid uptake into adipocytes is increased, resulting in the synthesis and storage of triglycerides. In contrast, when glucose levels are low (e.g., during fasting), upregulation of catecholamines and glucagon stimulate lipases that release free fatty acids into the circulation to be used directly by peripheral tissues as fuel for the Krebs cycle or are transported to the liver to be converted into ketone bodies. The ketone bodies acetoacetate and β-hydroxybutyrate are important substrates for oxidative phosphorylation in most tissues, including the heart, brain, kidney, and skeletal muscle. Because generation of acetyl-CoA by fatty acid β-oxidation (either directly or through catabolism of ketone bodies) occurs independent of PDH, lipids provide an alternative fuel for oxidative phosphorylation when glucose availability is limited or PDH activity is impaired.
Protein catabolism
Although typically protected as a fuel source, amino acids can be mobilized for energy production in times of energy need (such as starvation or critical illness). As part of the metabolic stress response mediated by a decrease in insulin and upregulation of cortisol, catecholamines, tumor necrosis factor-α, and interleukin (IL)-1 and IL-6, protein degradation can occur with release of amino acids from skeletal muscle (see also Chapter 80 ). Amino acid catabolites can either enter the Krebs cycle by way of acetyl-CoA or can be used to fuel gluconeogenesis.
Krebs cycle
The Krebs cycle summarizes a circular series of nine reactions that occur in the mitochondrial matrix in which acetyl-CoA derived from glycolysis, β-oxidation, or protein catabolism is metabolized to two molecules of CO 2 , one molecule of guanosine triphosphate (GTP), three molecules of NADH, and one molecule of FADH 2 . GTP is equivalent to ATP in terms of energy charge and is ultimately converted to ATP by the enzyme nucleoside diphosphokinase. Although oxygen itself is not part of the Krebs cycle, its presence at the end of the mitochondrial electron transport system ensures recycling of NAD + and FAD required in the Krebs cycle.
The first step in the Krebs cycle catalyzes the formation of the six-carbon citrate molecule (hence, the alternative name of the Krebs cycle is the citric acid cycle ) from the two-carbon acetyl-CoA and four-carbon oxaloacetate. This reaction is catalyzed by the enzyme citrate synthase and is the rate-limiting step of the Krebs cycle. With each revolution of the cycle, the fuel substrate that entered the cycle as acetyl-CoA is completely oxidized to CO 2 and water, and oxaloacetate is regenerated to again react with a new acetyl-CoA molecule.
Mitochondrial oxidative phosphorylation
Some 2 to 4 billion years ago, aerobic bacteria invaded and subsequently colonized a form of primordial eukaryotic cells that lacked the ability to use oxygen. Over the ensuing millions of years, a critical symbiotic relationship developed between the cell and aerobic bacteria that has remained steadfast through evolution. , The cell nucleus has evolved to regulate structure and overall cell function, while the oxygen-consuming bacteria evolved into mitochondria specialized in the energy production necessary for the development of increasingly complex species, including humans. Despite extensive intercommunication between mitochondria and their nuclear-cytosol host, mitochondria have retained many features characteristic of their bacterial origins: (1) mitochondria arise only from other mitochondria; (2) mitochondria maintain their own genome that, in contrast to eukaryotic nuclear DNA, is circular in structure, lacking in associated histones, and is replicated up to dozens of times; (3) mitochondria synthesize their own proteins that, like bacterial proteins, include N -formyl methionine at the protein amino terminus; and (4) many antibiotics that inhibit protein synthesis in bacteria are also toxic to mitochondria. The independent mitochondrial genome contains genes that encode 37 proteins involved in the ETS, enzymes comprising the Krebs cycle, fatty acid β-oxidation, and pyruvate oxidation and the machinery needed for mitochondrial gene translation. Notably, nuclear DNA encodes approximately 1500 additional mitochondrial proteins, including 79 of the 92 subunits of the ETS complexes.
Mitochondrial oxidative phosphorylation is fueled by pairs of elections originating from NADH and FADH 2 ( Fig. 79.3 ). These electrons are shuttled along the mitochondrial ETS, ultimately reducing oxygen to water. To capture as much of the energy released in a usable form when strong reducing agents such as NADH and FADH 2 react with a powerful oxidizing agent such as oxygen, mitochondria “step down” the reducing potential of these molecules through a controlled series of intermediate compounds in the ETS with progressively lower reducing potentials. Five complexes of proteins and cytochromes embedded within the inner mitochondrial membrane comprise the ETS, including NADH dehydrogenase–ubiquinone oxidoreductase (complex I), succinate dehydrogenase–ubiquinone oxidoreductase (complex II), ubiquinone–cytochrome c oxidoreductase (complex III), cytochrome c oxidase (complex IV), and ATP synthase (complex V). The cofactors ubiquinone (coenzyme Q10) and cytochrome c facilitate the transfer of electrons from complexes I and II to complex III and from complex III to complex IV, respectively. Electrons essentially cascade along these protein/cytochrome complexes toward complex IV, where they are finally passed to molecular oxygen, generating water. Protons generated during these reactions are pumped across the inner mitochondrial membrane by complexes I, III, and IV, generating a proton motive force. The resulting electrochemical gradient across the inner mitochondrial membrane drives ATP synthesis by the chemiosmotic principle . Energy for ATP synthesis arises from an influx of these protons back into the matrix through the rotary motor of ATP synthase. For each pair of electrons, three molecules of ATP are produced. This process is termed oxidative phosphorylation because the reduction of molecular oxygen to water is linked to the addition of inorganic phosphate to ADP, producing the high-energy terminal pyrophosphate in ATP. Aerobic respiration through oxidative phosphorylation is up to 15 times more efficient than anaerobic metabolism, producing 30 to 34 ATP molecules. ATP molecules are transferred out of the mitochondria through a specific adenine nucleotide translocase (ANT) antiporter on the inner mitochondrial membrane and through a voltage-dependent anion channel (VDAC) on the outer mitochondrial membrane for use as energy currency for all cellular functions. The overall metabolism of glucose through cellular respiration can be summarized as follows (the negative ΔG indicates that the overall reaction can occur spontaneously):
Glucose+6O2→6CO2+6H2O+heat
ΔG=-2880 kJ per molecule of glucose

Although multiple regulatory steps exist along the pathways of cellular respiration, the following three are preeminent:
- 1.
Oxygen availability to serve as the ultimate electron acceptor
- 2.
Availability of nutrient metabolism to generate reducing equivalents in the form of NADH and FADH 2
- 3.
The overall cellular energy state defined by the ratios of NADH/NAD + , ATP/ADP, and acetyl-CoA/CoA
Oxygen toxicity
In health, approximately 1% to 2% of oxygen consumption is directed toward the production of ROS and an even greater amount is “uncoupled” from energy production and lost as heat. Heat production varies substantially from tissue to tissue, being low in the heart and much higher in skeletal muscle and brown fat. Despite the terminology, uncoupling is itself a controlled process regulated by a series of uncoupling proteins (UCPs) that insert within the inner mitochondrial membrane and provide an alternative pathway for protons to move back into the matrix, bypassing ATP synthase. For example, UCP-1 present in brown fat provides a vital source of heat generation for neonates and hibernating mammals.
Successive one-electron additions to molecular oxygen result in the production of superoxide anion, hydrogen peroxide (H 2 O 2 ), hydroxyl radical, and water, respectively. The partially reduced oxygen compounds are referred to as reactive oxygen species and are responsible for the so-called antagonistic pleiotropy characteristic of oxygen. Under normal conditions, small amounts of constitutively produced ROS, primarily in the form of H 2 O 2 , are necessary for regulation of a variety of intracellular signaling pathways. However, increased production of ROS or decreased availability of endogenous antioxidant compounds (e.g., manganese superoxide dismutase, catalase, glutathione peroxidase, uric acid, vitamin C, and vitamin E) risks deleterious oxidative modifications in cellular membrane or intracellular molecules. Although the majority of cellular ROS is sequestered within the mitochondria—thereby protecting the redox state of other cellular molecules—an increase in ROS production can quickly outstrip antioxidant defense mechanisms and cause oxidant injury to cellular proteins, lipids, and DNA.
Nitrogen is also involved in the generation of another group of toxic metabolic moieties known as reactive nitrogen species (RNS). Endogenous nitric oxide (NO) can react with the superoxide anion to form the powerful oxidant peroxynitrite, which can cause peroxidation of lipids within cell and organelle membranes, damage to various elements of the mitochondrial electron transport chain and ATP synthase, inhibition of glyceraldehyde 3-phosphate dehydrogenase, injury of the sodium-potassium adenosine triphosphatase pump, disruption of sodium channels, production of deoxyribonucleic acid (DNA) strand breaks, and activation of the polyadenosine ribosyl phosphate system. Peroxynitrite can also irreversibly inhibit the Krebs cycle enzyme aconitase and increase mitochondrial proton leak.
Increased ROS- and RNS-mediated stress can promote the formation of the mitochondrial permeability transition pore (MPTP), leading to release of proapoptotic proteins, including cytochrome c, into the cytoplasm. , , This can trigger activation of the apoptotic pathway, orchestrating cell death. Integrity of the mitochondrial ETS defines a fine line between maintaining mitochondrial homeostasis and efficient respiration versus excessive ROS/RNS production, mitochondrial dysfunction, cellular dysfunction, and cell death.
Impaired cellular respiration in critical illness
Mitochondrial ATP production relies on a complex series of chemical reactions that, overall, are controlled by metabolic demand. During periods of rest, metabolic needs are generally low and less energy is required. Conversely, during periods of activity and stress, metabolic requirements increase with a concurrent rise in cellular oxygen utilization and ATP production. An increase in ATP production ensures that sufficient energy is available to respond to the activity or stress and prevents the cell from depleting ATP levels below a critical threshold that can trigger apoptotic and necrotic cell death pathways. With an acute decrease in oxygen delivery (DO 2 ) to tissues, such as with cardiac arrest, shock, or hemorrhage, mitochondrial energy output will necessarily fall. A concurrent increase in anaerobic respiration through glycolysis will attempt to partially offset the decline in ATP production, but if DO 2 is not rapidly restored, bioenergetic homeostasis will be perturbed, leading to eventual cell injury and organ dysfunction.
In critical illness, shock represents the imbalance between oxygen (and substrate) delivery and oxygen (and substrate) utilization such that cellular metabolic demands are not met. Early in the course of shock and other inflammatory conditions, the total body metabolic rate rises with the surge in catecholamine and catabolic hormones. In healthy volunteers, injection of endotoxin leads to a systemic inflammatory response characterized by fever and a rapid rise (within hours) in total-body oxygen consumption (VO 2 ). Over time, total-body VO 2 and energy expenditure decrease with increasing illness severity in both animal models and critically ill patients. Following trauma, failure to attain a low-normal level of VO 2 has been associated with increased risk of multiorgan dysfunction. Survivors of critical illness subsequently demonstrate a return of VO 2 toward baseline levels.
Changes in total-body VO 2 and energy expenditure suggest that mitochondrial respiration is altered in critical illness. The inability of cells to effectively use oxygen to generate ATP is termed cytopathic hypoxia . , To date, most studies have focused on sepsis, though increasing evidence supports the development of cytopathic hypoxia after trauma, brain injury, and cardiac arrest. Ultrastructural mitochondrial abnormalities in a variety of organ systems have been recognized in models of sepsis for over 30 years. For example, Crouser demonstrated marked swelling and disruption of mitochondrial architecture in the liver following cecal ligation and puncture (CLP) in a mouse model ( Fig. 79.4 ). Morphologic abnormalities have also been noted in mitochondria taken from the heart, kidney, liver, endothelial cells, intestinal epithelial cells, and skeletal muscle in animal models of sepsis. In human sepsis, heart and liver biopsies obtained immediately postmortem from adult nonsurvivors similarly show swollen and damaged mitochondria.

Functional alterations in mitochondrial respiration have been more variably reported as increased, decreased, or unchanged in short-term sepsis models. However, longer-term models that may better align with human critical illness more consistently demonstrate depressed mitochondrial function. In human sepsis, most—but not all—studies demonstrate decreased mitochondrial VO 2 in both immune and nonimmune cells. , , For example, in a study of pediatric patients with septic shock and multiorgan dysfunction, direct measurements of mitochondrial respiration in peripheral blood mononuclear immune cells (PBMCs) showed that spare respiratory capacity—which reflects the ability of the ETS to keep pace with a rise in energy demand—was decreased and mitochondrial uncoupling was increased compared with noninfected controls. Similar findings have been reported in PBMCs in adults with sepsis, though one study demonstrated a progressive increase in mitochondrial respiration from day 1 to day 2 through day 6 to day 7 of sepsis compared with healthy controls.
When considering bioenergetic impairment in sepsis, investigators have most commonly focused on NADH dehydrogenase–ubiquinone oxidoreductase (complex I) and cytochrome oxidase (complex IV). As the largest complex of the ETS, complex I is subject to impairment from changes in a variety of protein subunits. Multiple studies have demonstrated decreased activity of ETS complex I in sepsis models and humans. Complex IV contains two heme subgroups (cytochrome a and a3) that assist in the final transfer of electrons to reduce oxygen to water. A reduced cytochrome a,a3 redox state in the absence of tissue hypoxia indicates a defect in mitochondrial oxygen use and suggests impaired oxidative phosphorylation. A number of investigators have demonstrated reduced cytochrome a,a3 redox status during endotoxemia and Gram-negative bacteremia in the heart, brain, skeletal muscle, and intestine in animals. , In addition, decoupled cytochrome a,a3 was more likely in adults who did versus did not develop multiorgan system dysfunction following trauma despite normalization of DO 2 . Interestingly, Verma et al. demonstrated that, when complex IV activity is stimulated by administration of caffeine, myocardial function and survival improved after cecal ligation and puncture in a rodent model.
Despite structural, biochemical, and functional evidence for mitochondrial dysfunction in sepsis, trauma, and other critical illness, the literature is less clear regarding these effects on tissue ATP availability. Several studies have reported that ATP levels are preserved in septic myocardium, but others have shown decreased high-energy phosphates with endotoxemia. , In a study of 28 human adults with severe sepsis, 12 (43%) of whom died of sepsis-related MODS, nonsurvivors had lower levels of ATP in skeletal muscle compared with survivors. However, even preservation of ATP does not imply an absence of mitochondrial dysfunction in sepsis. When DO 2 and cellular hypoxia are limited (as in shock), cells may adapt to maintain viability by downregulating VO 2 , energy requirements, and ATP demand. , , Thus, a fall in ATP utilization may help to preserve ATP even if energy production is diminished. In the heart, this response is referred to as myocardial hibernation and classically occurs following an acute coronary syndrome. If cellular metabolic activity continued unchanged despite mitochondrial dysfunction, then ATP levels would inevitably diminish and cell death pathways would be activated. Because cell death does not appear to be a primary feature of sepsis-induced organ dysfunction, it follows that cells may instead adapt to cope with the falling energy supply. , Thus, finding preserved ATP during sepsis reveals little about the integrity of oxidative phosphorylation.
The precise etiology by which mitochondrial function is altered in critical illness remains unclear. A number of mutually compatible mechanisms may contribute to a clinical state of cytopathic hypoxia under pathologic conditions, including:
- 1.
Decreased tissue oxygen (and substrate) delivery impairing oxidative phosphorylation
- 2.
Inhibition of PDH with decreased flux of acetyl-CoA through the Krebs cycle
- 3.
NO-mediated inhibition of ETS complexes
- 4.
Peroxynitrite-mediated inhibition of the Krebs cycle and ETS complexes I, II, and V
- 5.
Depletion of cellular NADH due to activation of the enzyme poly(ADP-ribose) polymerase (PARP)-1 in response to ROS-mediated nuclear DNA damage
- 6.
Insufficient mitochondrial turnover due to impaired mitochondrial biogenesis, fission/fusion, or mitophagy
- 7.
Hormonal alterations, including relative hypothyroidism
- 8.
Pharmacologic inhibition of mitochondrial function as a side effect of many drugs commonly used in the intensive care unit (ICU) setting, including some antibiotics, catecholamines, and sedatives (propofol in particular)
Injection of lipopolysaccharide (LPS) endotoxin into healthy human volunteers also results in widespread suppression of genes regulating mitochondrial energy production and protein synthesis. Differential expression of mitochondrial genes in blood cells has been reported for several diseases in which bioenergetic failure is a postulated mechanism. , In a study of adult sepsis, decreased ETS gene expression correlated with impaired activity of ETS complexes I and IV in skeletal muscle. In pediatric sepsis, suppression of nuclear-encoded mitochondrial genes was greatest in the subgroup of patients with the highest rates of MODS and death.
Accumulating evidence also supports a propagative role for mitochondrial damage to feed-forward the systemic inflammatory response and contribute to distant organ injury in trauma and sepsis. Oxidative injury due to an increase in mitochondrial ROS and RNS can fragment mitochondrial DNA (mtDNA). Oxidatively damaged mtDNA fragments can be exported from the mitochondrial matrix through the MPTP to the cytosol or the extracellular space. In the cytosol, mtDNA promotes the formation of the Nod-like receptor-P3 (NLRP3) inflammasome, a supramolecular platform that increases the release of the proinflammatory cytokines IL-1β and IL-18. In the circulation, mitochondria are recognized by the innate immune systems as a danger-associated molecular pattern (DAMP) via the pattern recognition receptor, toll-like receptor 9, due to their evolutionary similarities with bacterial pathogens. Several studies in human sepsis and trauma have demonstrated that an increase in circulating levels of mtDNA is associated with adverse outcomes. , Consequently, mtDNA has been proposed as a potential novel biomarker linked to mitochondrial dysfunction in critical illness.
Despite demonstration of altered mitochondrial structure and function, there is actually little evidence that impaired mitochondrial respiration causes organ dysfunction in critical illness. In fact, the decrease in oxygen utilization and overall metabolic rate seen in sepsis parallels similar physiologic changes observed in hibernating mammals that reduce their metabolism to promote survival amid decreased substrate availability. Indeed, despite physiologic and biochemical mitochondrial dysfunction in many tissues recovered from animal models and human patients with sepsis, there tends to be minimal evidence of cell death in dysfunctional organ systems. Moreover, survivors of critical illness rapidly recover organ function. Therefore, although a profound and prolonged metabolic downregulation can trigger cell death, the observation that mitochondrial hibernation is a conserved physiologic response across models and species suggests that some degree of transient energetic swoon early in sepsis (and other critical illnesses) could be an adaptive response to ischemia, hypoxemia, and shock. , , The use of induced hypothermia to decrease metabolic demand and protect vital organs during cardiopulmonary bypass supports the notion that a decrease in metabolic demand may help to restore bioenergetic homeostasis when oxygen (and substrate) delivery—and thus ATP production—is limited. Although this may manifest clinically as organ dysfunction, protected bioenergetic homeostasis may help to prevent cell death and offer the prospect of organ recovery once oxygen (and substrate) supply is restored.
Clinical assessment of oxygen utilization
Biochemical pathways and cellular energetics may seem far removed from the daily care of patients in the pediatric ICU, where discussions about the respiratory and circulatory systems seem to dominate. However, at its core, the goal of critical care medicine is to maintain and support the basic physiologic functions of the patient until the patient’s own homeostasis returns. This means that the underlying goal of cardiopulmonary and other organ support is to optimize cellular function and that function requires energy derived from the metabolism of oxygen. Inherent in this goal is the need to assess the adequacy of DO 2 and utilization at the bedside.
Lactate
Lactate is a commonly used surrogate for tissue hypoxia, as it is produced as a by-product of anaerobic metabolism. In health, blood lactate concentration is maintained in the approximate range of 0.5 to 1.5 mmol/L. In and of itself, lactate is not harmful but rather promotes ongoing glycolysis and provides an alternative metabolic fuel through gluconeogenesis. However, lactate can accumulate in the absence of oxygen or with mitochondrial dysfunction, such that hydrogen ions are not able to be used to convert ADP to ATP through oxidative phosphorylation. This combination of hyperlactatemia and acidosis is called lactic acidosis . Blood lactate monitoring is frequently performed in critically ill patients, usually with the aim of detecting tissue hypoxia leading to anaerobic respiration, traditionally termed type A lactic acidosis . However, other processes not related to tissue hypoxia can also result in increased blood lactate levels, such as with excess adrenergic stimulation that increases aerobic glycolysis and pyruvate production beyond the rate of mitochondrial metabolism, termed type B lactic acidosis and common in severe asthma, therapy with epinephrine, impaired PDH activity, or delayed lactate clearance seen in patients with hepatic dysfunction.
The causal relationship between anaerobic hyperlactatemia and tissue hypoxemia has been confirmed in studies that limit VO 2 by decreasing DO 2 below a threshold with a resulting increase in lactate levels. , Accordingly, in the early phase of septic shock, hyperlactatemia is accompanied by oxygen supply dependency, and initial lactate correlates with increased mortality in children with septic shock. Moreover, lactate normalization with 4 hours of sepsis recognition predicts survival in pediatric sepsis. , In adults, an elevated blood lactate concentration may be sufficient evidence that tissue perfusion is inadequate even if hypotension is not present (so-called cryptic shock ). Moreover, the revised Sepsis-3 criteria now include both hypotension requiring vasopressors and hyperlactatemia greater than 2 mmol/L in the operational criteria for septic shock in adults.
Despite wide availability and an association with both global tissue hypoxia and poor outcome, the exact utility of measuring initial and serially trending lactate remains unclear. For example, in a study of 123 adult patients with vasopressor-dependent septic shock, mortality remained high (20%) in the 45% of patients found to be “nonlactate expressers” (defined as a lactate level <2.4 mmol/L). Additionally, no data are currently available to support monitoring lactate levels beyond the immediate resuscitative period, especially once DO 2 has been restored. The numerous etiologies of increased lactate and the lack of sensitivity and specificity for regional tissue perfusion and respiration remain important limitations in using lactate to monitor cellular bioenergetics. A common clinical scenario occurs during volume resuscitation of patients (iatrogenic hyperchloremia) or epinephrine infusion, in which a chloride load and type B lactic acidosis can be interpreted as ongoing shock, thus prompting additional fluid and more vasoactive-inotropic support. This scenario may initiate a vicious cycle with the potential for overresuscitation. Therefore, the treatment of the patient with lactic acidosis should be aimed at the underlying disease, with resuscitation guided by the overall clinical improvement of the patient and not just the lactate concentration itself. Accordingly, the absence of an elevated lactate should not slow resuscitative efforts in children with other indices of altered perfusion.
Venous oxygen saturation
Bedside evaluation of DO 2 and VO 2 would be of great value to the clinician. Unfortunately, most of the methods of calculating VO 2 rely on cardiac output (via the Fick principle), which results in a situation in which a single measured variable is included in two parts of a regression analysis, so-called mathematical coupling . This dependency leads to amplification of any error in that measurement and may result in an apparent relationship between variables that does not truly exist. Other methods to measure VO 2 in intubated critically ill patients require use of a metabolic cart, but routine use is currently lacking. As in many areas of critical care medicine, when something cannot be measured directly, a surrogate measure must be used.
In practice, the central venous oxygen saturation (Scv o 2 ) or mixed venous oxygen saturation (Smv o 2 ) can be used as a continuous or intermittent measure to assess the global balance of D o 2 and consumption in a patient with septic shock. As central venous catheters have largely replaced the use of pulmonary arterial catheters, particularly in pediatrics, Scv o 2 is now more commonly measured than Smv o 2 . Scv o 2 is ideally measured from a catheter with its tip at the superior vena cava–right atrial junction. Measurements taken from a femoral catheter with its tip in the inferior vena cava are thought to be less reliable due to greater variability in splanchnic oxygen utilization. An Scv o 2 less than 70% indicates that tissue oxygen extraction is increased from normal, suggesting inadequate D o 2 due to either low cardiac output, decreased arterial oxygen content, or both. However, Scv o 2 may also be low if oxygen utilization is increased, as in states of high metabolic demand (e.g., fever, seizure, or hyperthyroidism). Alternatively, elevated Scv o 2 above 80% suggests that oxygen utilization may be impaired due to mitochondrial dysfunction, reflecting a global state of cytopathic hypoxia.
In a trial of early goal-directed therapy in adult septic shock by Rivers et al., an Scv o 2 greater than 70% was targeted as a primary hemodynamic end point. Although three more recent trials failed to demonstrate benefit from routine Scv o 2 monitoring, an Scv o 2 greater than 70% remains the recommended target in current pediatric shock guidelines. A trial in children with fluid refractory shock found that targeting an Scv o 2 greater than 70% for 72 hours compared with standard therapy reduced mortality from 39.2% to 11.8% ( P = .002). Furthermore, in a pediatric prospective cohort trial evaluating the effect of intermittent Scv o 2 monitoring on 120 children with fluid refractory septic shock, children who had intermittent Scv o 2 monitoring performed at 1, 3, and 6 hours had significantly lower in-hospital mortality (33% vs. 55%, P = .02) and a reduction in the number dysfunctional organs (2 vs. 3, P < .001).
An important limitation of Scv o 2 is the need for central venous access, making this laboratory value difficult to obtain in many children who may not otherwise require such an invasive procedure. In addition, continuous Scv o 2 measurements require a specialized catheter that may not be available in all centers. Several studies have also failed to show an advantage of using Scv o 2 over other markers, including lactate, in predicting in-hospital mortality. , Three large randomized trials also recently failed to show that routine Scv o 2 monitoring improved outcomes during resuscitation of adults with septic shock. Consequently, although both low (<70%) and high (>80%) Scv o 2 may provide general insight into the global state of D o 2 -V o 2 mismatch, this measure, much like lactate, cannot detect tissue-specific changes in bioenergetic homeostasis.
Microdialysis
Microdialysis allows for the measurement of energy-related metabolites within the interstitial space of a regional tissue bed. This is most commonly performed using a thin, flexible catheter with a semipermeable membrane inserted into skeletal muscle. A solution of a known solute concentration is slowly pumped into the catheter, and soluble small molecules—such as glucose, lactate, pyruvate, and glycerol—equilibrate across the membrane. The fluid is then collected and analyzed as a regional measurement of cellular metabolic activity. Because blood and D o 2 are diverted away from skeletal muscle to critical organs (brain, heart, and kidneys) in shock, changes in muscle cellular respiration may be a sensitive indicator of inadequate systemic perfusion. Indeed, animal and human studies in sepsis and hemorrhage have demonstrated an association between microdialysis lactate/pyruvate ratios and outcome. , Further studies are needed to validate the utility of microdialysis as a clinically useful measure of cellular respiration.
Near-infrared spectroscopy
Near-infrared spectroscopy (NIRS) enables continuous, noninvasive bedside monitoring of regional tissue oxygenation and mitochondrial complex IV redox state. As with pulse oximetry, NIRS is based on the principle that the oxygen-carrying pigments hemoglobin and myoglobin and cytochrome a,a3 have well-defined absorption spectra that are influenced by oxygen binding. NIRS technology thus uses a modification of the Beer-Lambert law, which describes the relationship between absorption of light and the concentration of deoxygenated hemoglobin (Hb), oxygenated hemoglobin, and intracellular chromophores (cytochrome a,a 3 ).
Clinical utilization of NIRS to date has generally focused on monitoring tissue-specific oxy- and deoxyhemoglobin concentrations, most commonly in brain, kidney, and skeletal muscle. Available NIRS monitors are mathematically weighted toward venous blood, thus largely reflecting deoxygenated Hb levels, and display a number (referred to as regional S o 2 or rS o 2 ) that varies with local D o 2 and extraction. A decrease in NIRS rS o 2 has been correlated with a fall in local tissue perfusion in animal models of shock, decreased cardiac output in infants following cardiac surgery, and predicted fluid responsiveness in dehydrated children. However, large variations in “normal” rS o 2 levels, the lack of defined “critical threshold” for rS o 2 , and the inability of rS o 2 to differentiate between changes in D o 2 or V o 2 have limited the universal acceptance of NIRS monitoring.
In addition to hemoglobin, NIRS can assess the cytochrome a,a 3 redox state of complex IV in the mitochondrial ETS. Cytochrome a,a 3 is the terminal component along the ETS that reduces oxygen to water, and it remains in a reduced state during hypoxemia. The absorption spectrum of cytochrome a,a 3 in its reduced state shows a weak peak at 700 nm, whereas the oxygenated form does not. Therefore, monitoring changes in the cytochrome a,a 3 redox state can provide a measure of the adequacy of oxidative metabolism. To date, however, NIRS assessment of mitochondrial redox state, while promising, has been limited to research settings.
Optical spectroscopy
Similar to NIRS, optical spectroscopy uses absorption of light by tissues to assess concentration of various analytes of interest. Advances in optical spectroscopy have made it possible to measure the oxygenation state of myoglobin independently from that of hemoglobin. Myoglobin is an intracellular protein found in skeletal and cardiac muscle cells that is involved in the transport of oxygen from the cytoplasm to the mitochondria. Quantification of myoglobin saturation (percentage of total myoglobin bound with oxygen) provides a direct measure of intracellular oxygen availability. Arakaki et al. at the University of Washington recently demonstrated a progressive decrease in noninvasive muscle oxygenation measurements with increasing severity of shock in trauma patients.
Optical spectroscopy has the potential to separately identify the oxygenation state in anatomically and physiologically distinct tissue regions, including the vascular (hemoglobin), cellular (myoglobin), and mitochondrial (cytochromes) levels. However, clinical application of such data to guide therapy is not yet clear.
Tissue oxygen tension
Tissue oxygen tension (t po 2 ) measures the partial pressure of oxygen within the interstitial space. Traditional probes use Clark electrodes, but newer techniques are now available that can more accurately measure t po 2 without themselves consuming oxygen. A decrease in D o 2 would be expected to lower t po 2 , whereas a decrease in V o 2 would raise t po 2 (so long as D o 2 levels were not concurrently diminished). In sepsis models, several studies have demonstrated that skeletal muscle and mucosal t po 2 are unchanged or increased, suggesting a fall in V o 2 either concurrent with or greater than the decrease in D o 2 . , In human studies, brain t po 2 monitoring has been demonstrated to improve outcomes following traumatic brain injury. A more recently developed technique to measure mitochondrial oxygen tension (mito po 2 ) may provide a new opportunity to assess mitochondrial function in vivo by noninvasively monitoring the oxygen-dependent change in lifetime of protoporphyrin IX upon photoexcitation with a pulse of light. More studies are needed to determine the benefits of t po 2 (and mito po 2 ) monitoring in pediatric critical illness.
Magnetic resonance spectroscopy
Magnetic resonance spectroscopy (MRS) uses radiolabeled molecules to noninvasively measure in vivo tissue metabolite concentrations. MRS has been used clinically for more than two decades in neurologic disorders to measure blood vessel distribution and architecture, blood flow velocity, regional perfusion and blood volume, blood and tissue oxygenation, lactate production and intracellular pH, Krebs cycle activity, and mitochondrial oxidative phosphorylation in the brain. For bioenergetic studies, phosphorus-31 MRS is primarily used to detect changes in phosphocreatine, ATP and inorganic phosphate, and certain compounds related to membrane synthesis and degradation. Because phosphocreatine is the primary ATP buffer in muscle, depletion in this molecule leads to a drop in ATP levels. Proton MRS can also be used to measure four markers related to oxygen metabolism: the peak of N -acetyl-aspartate (NAA), an amino acid present in neurons that reflects the status of neuronal tissue; creatine, found in glia and neurons, which serves as a point of reference because its level is believed to be stable; choline, a constitutive component of cell membranes that reflects glial proliferation or membrane breakdown; and lactate, a marker of anaerobic metabolism and therefore of ischemia. Elevated lactate and decreased NAA are associated with worse neurologic outcome in neonates with asphyxial injury. , Although MRS is useful for diagnosis and prognosis, it is not currently practical as a method to guide minute-to-minute patient management.
Blood mitochondrial DNA
Under conditions of hypoxemia, ischemia, inflammation, and oxidative stress, mitochondrial damage can lead to fragmentation of mtDNA and release into the circulation. Therefore, it has been proposed that blood levels (both cellular and free plasma concentrations) of mtDNA may be a biomarker of mitochondrial dysfunction and overall severity of illness. , Increased levels of blood mtDNA have been reported in sepsis, trauma, and brain injury. , , Circulating mtDNA may also serve as DAMP, propagating inflammation and contributing to distant organ injury, as noted previously.
Mitochondrial- and bioenergetic-targeted therapy in critical illness
With increasing recognition of the central role of altered cellular respiration—and mitochondrial dysfunction in particular—in the pathogenesis of organ failure in critical illness, there has been emerging interest in the potential for metabolic- and mitochondrial-targeted therapeutic strategies to improve patient outcomes. A therapeutic strategy that partners an upregulation or downregulation of oxygen utilization to better match D o 2 may restore bioenergetic homeostasis and improve cellular—and thus organ—function. Several existing and novel agents have demonstrated potential to optimize cellular respiration and improve mitochondrial function, though questions of clinical utility remain largely unanswered at this time.
Antioxidants
As discussed earlier, ROS and RNS play essential roles in cell signaling. As such, their activity is normally tightly regulated by a network of intracellular antioxidants. In sepsis, cardiac arrest, and other severe illnesses, the production of ROS and RNS increases sharply (largely due to alterations of the mitochondrial ETS), leading to a wide array of local cellular damage and systemic inflammation. Several exogenous antioxidant supplements have been studied in animal models and humans with critical illness, including vitamin A, vitamin E, vitamin C, coenzyme A, selenium, melatonin, and N -acetylcysteine. Although antioxidant supplementation can successfully reduce oxidative stress, clinical trials thus far have failed to convincingly demonstrate an outcome benefit. A strategy that better targets antioxidants to the mitochondria has shown some promise in preclinical studies and may be better suited for use in critically ill patients. , Similarly, a series of small synthetic, positively charged peptides less than 10 amino acids in length, termed Szeto-Schiller (SS) peptides , can freely penetrate cell and mitochondrial membranes and have been shown to be effective in some ischemia-reperfusion oxidative stress models. Several early-phase human trials of mitochondrial-targeted antioxidants and SS peptides are ongoing.
Glycemic control
Hyperglycemia and insulin resistance are common findings in critically ill patients. The inability of cells to use glucose to generate pyruvate can lead to downstream limitations of oxidative phosphorylation and results in mobilization of fat and protein stores. Since the 1990s, there has been substantial interest in the use of exogenous insulin to restore normoglycemia in adult and pediatric critical illness. Despite widespread enthusiasm following an adult trial of insulin to achieve tight glycemic control (80–110 mg/dL) that reduced ICU mortality from 8% to 4.6%, subsequent studies have failed to replicate the overall benefit, including three randomized pediatric trials. , Interestingly, insulin has been shown to stimulate mitochondrial ATP production in human skeletal muscle ex vivo, and maintenance of normoglycemia with insulin preserves hepatic mitochondrial structure and function in adult critical illness. Thus, the potential for targeted insulin therapy and glycemic control to improve cellular bioenergetic homeostasis remains unclear (see also Chapter 84 ).
Substrate provision
Several studies suggest that, despite decreased activity of complex I of the mitochondrial ETS, complex II is relatively preserved in sepsis. Complex II transfers electrons from FADH 2 produced from the oxidation of succinate to coenzyme Q. Consequently, supplementation of succinate may help to restore overall ETS activity and ATP production, as has been suggested in animal models of sepsis. However, different insults may be prone to distinct pathophysiology and thus variable therapies. For example, in a porcine model of pediatric traumatic brain injury, mitochondrial ETS complex II exhibited the most profound decrease in activity. Alternative fuels for cellular respiration, including carnitine supplementation, have shown promising results in animal models, but direct evidence for benefit in humans has not yet been demonstrated. Similarly, supplementation with coenzyme Q, cytochrome c, and caffeine improves mitochondrial function in vitro and in animal models. , , , Thiamine supplementation has also been proposed as part of a metabolic resuscitation strategy. Thiamine (vitamin B 1 ) is an essential cofactor for PDH, and acute thiamine deficiency in critical illness may cause an acquired form of PDH deficiency. Several studies have tested the therapeutic benefits of thiamine in critically ill adults with septic shock, some with encouraging results that are awaiting confirmation in larger trials. ,
Mitochondrial biogenesis and mitophagy
All cells that undergo oxidative phosphorylation have robust quality control mechanisms to ensure a full complement of healthy mitochondria. Cells optimize the overall mitochondrial number, distribution, and function through a network of interrelated processes of biogenesis, fission, fusion, and mitophagy. Mitochondrial biogenesis is the process of synthesizing new functional mitochondria and can be induced by oxidative stress and inflammation. Data from both animal studies and septic patients have shown that mitochondrial biogenesis is associated with recovery of organ function and survival. Haden et al. showed that mitochondrial biogenesis is evident over 1 to 3 days following a nonlethal exposure to Staphylococcus aureus in a rodent model, with a subsequent recovery of oxidative phosphorylation. The same group further showed that sepsis survival could be improved in rodents treated with daily exposure to a low dose of carbon monoxide (CO), which stimulates mitochondrial biogenesis. A recent phase I trial in adults with acute respiratory distress syndrome demonstrated safety and tolerability of low-dose inhaled CO.
Similarly, removal of dysfunctional mitochondria—termed mitophagy —has also been shown to be protective in sepsis. For example, in both liver and kidney of hyperglycemic critically ill rabbits, biochemical markers indicating insufficient mitophagy were more pronounced in nonsurviving animals. Interestingly, after 3 and 7 days of illness, mitophagy was better preserved in animals treated with insulin to preserve normoglycemia, which correlated with improved mitochondrial function and less organ damage. Pharmacologic agents that induce mitochondrial biogenesis, such as pioglitazone, resveratrol, and recombinant human TFAM (transcription factor A, mitochondrial; rhTFAM) and that simulate mitophagy, such as rapamycin, are currently being explored as potential therapeutic strategies to recover mitochondrial function and restore bioenergetic homeostasis in critical illness.
Membrane stabilizers
Ultrastructural evidence of mitochondrial injury in critical illness includes damage to the inner mitochondrial membrane and organelle swelling. These features are associated with loss of the electrochemical gradient that drives ATP production through oxidative phosphorylation. Although the mechanisms underlying these physiologic changes remain incompletely understood, opening of the MPTP is believed to be involved. A number of compounds that inhibit pore opening, including cyclosporine A and melatonin, are being investigated as another approach to restore mitochondrial function through membrane stabilization. ,
Hibernation
Another potential strategy to restore bioenergetic homeostasis that could be pursued in the presence of impaired cellular respiration is to suppress metabolic energy expenditure. In many hibernating mammals, a purposive metabolic downregulation is a crucial response that facilitates tolerance to a lack of energetic substrates during harsh environmental conditions and promotes survival. , The hibernating state prevents a cellular bioenergetic crisis by reducing demand for ATP when substrate or oxygen supply is low and decreases mitochondrial oxidative stress. Although humans do not hibernate and have only a limited tolerance to diminished oxygen or substrate delivery to vital organs, the impairment in cytochrome oxidase activity observed during sepsis mimics that of true hibernation, suggesting that, at least early on, such impairment may be an adaptive response to an inflammatory insult. Following myocardial ischemia, myocardial hibernation has been well described, with subsequent functional recovery if adequate perfusion is promptly restored.
Attempts to reduce metabolic demand through induced moderate hypothermia after neonatal hypoxic-ischemic injury, cardiac arrest, traumatic brain injury, and hyperammonemic metabolic crises have demonstrated variable success. Ongoing challenges to induced hypothermia include unclear optimal timing, depth, and duration of this therapy.
Mitochondrial transplantation
Transplantation of autologous healthy mitochondria (e.g., from skeletal muscle) has been shown to act both extracellularly and intracellularly to enhance tissue V o 2 , improve bioenergetic homeostasis, and increase ATP synthesis in cells and tissues after ischemia-reperfusion injury. Studies have shown that exogenous mitochondria can be incorporated into cardiac cells ex vivo, with many eventually fusing with the endogenous mitochondria network. In five children with congenital heart disease requiring extracorporeal membrane oxygenation (ECMO) after myocardial ischemia, direct epicardial injection of isolated autologous mitochondria was followed by improved myocardial function within 24 to 48 hours and ECMO decannulation within a median of 4 days. The therapeutic potential for mitochondrial transplantation, although preliminary, is promising.
Conclusions
Although the restoration of tissue perfusion and oxygen/substrate delivery remains a primary goal in critical illness, alterations in cellular respiration contribute to a bioenergetic imbalance that result in cell and, ultimately, organ dysfunction. As discussed, impaired D o 2 can decrease the ability of cells to produce ATP. However, metabolic alterations that affect overall bioenergetic homeostasis may develop, and even persist, irrespective of the state of D o 2 . Indeed, it may well be the case that utilization of oxygen—or some other metabolic substrate—is of even greater importance to cell survival, organ function, and patient outcomes than normalization of D o 2 . Although shock is classically defined as an imbalance between oxygen (and substrate) delivery and demand, it is ultimately the state of cellular respiration rather than circulatory dysfunction that determines outcome from critical illness.
Key references
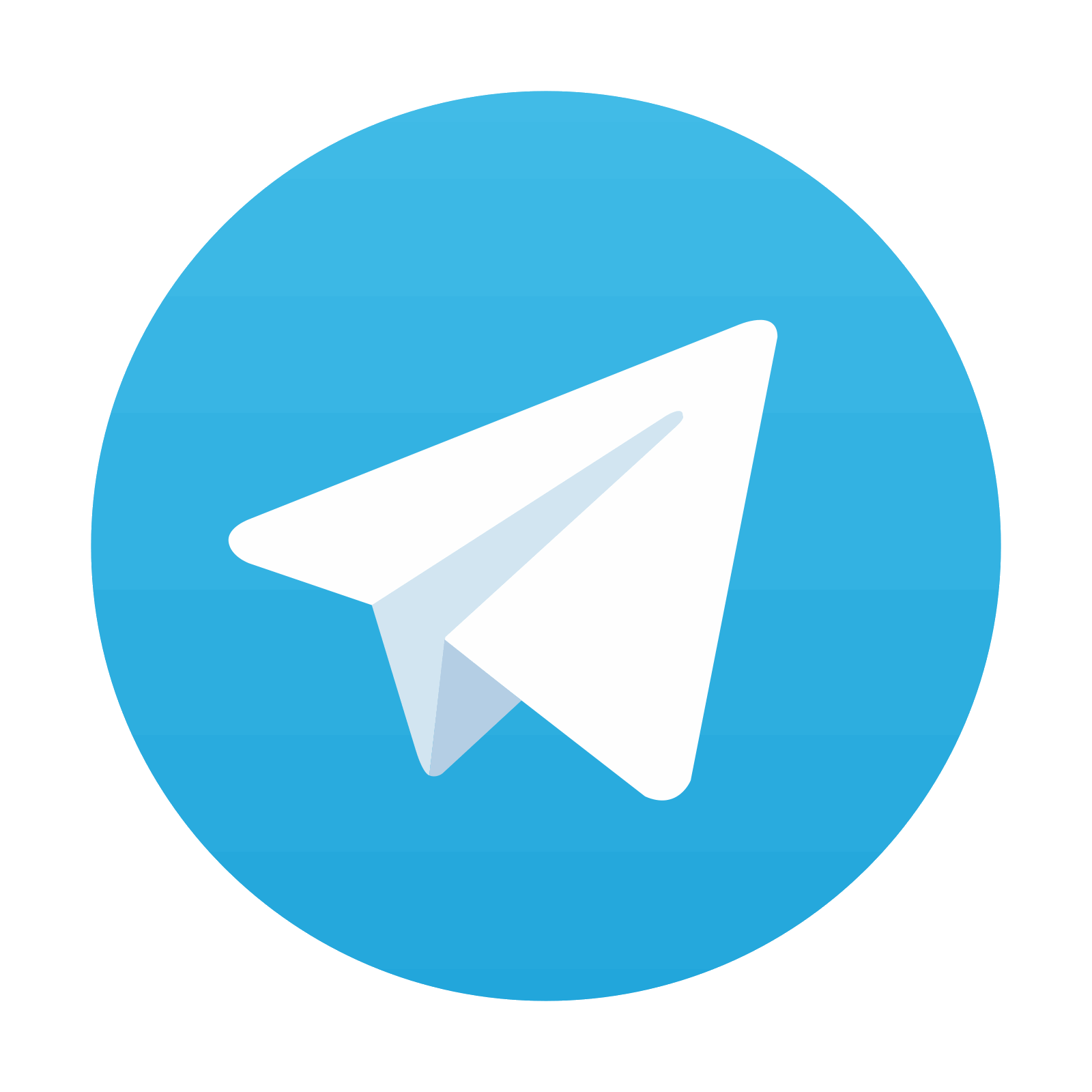
Stay updated, free articles. Join our Telegram channel
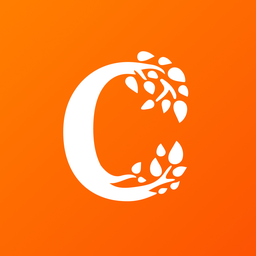
Full access? Get Clinical Tree
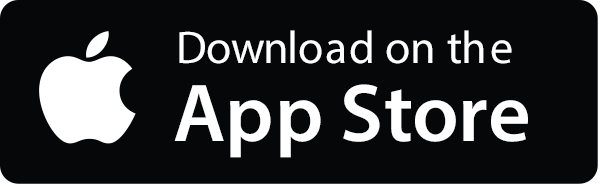
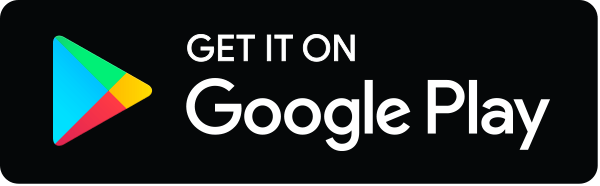
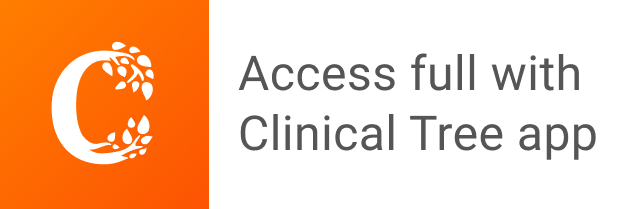