33 Cardiopulmonary Cerebral Resuscitation
Between 1960—when closed-chest compressions were first introduced—and 2000, there was little or no change in long-term survival after cardiac arrest.1,2 However, regional efforts to improve resuscitation practices at multiple levels, including the emergency response and the post–cardiac arrest care, have resulted in significant improvements in meaningful survival over time.3,4 There is accumulating evidence about which aspects of post–cardiac arrest management influence final outcomes,5,6 and specific patterns of physiologic changes after cardiac arrest have been described.7 Improving outcome will require an integrated approach to immediate resuscitation and subsequent intensive care management. This chapter will review the epidemiology of cardiac arrest, the initial approach for reversing cardiopulmonary arrest, modifications of this approach appropriate for specific disease states, and post–cardiac arrest care designed to minimize brain injury.
Epidemiology
In industrialized countries, heart disease is the overall leading cause of death. Estimates of the incidence of cardiopulmonary arrest outside the hospital vary from 55 to 100 to 120 events per 100,000 people per year,8,9,10 with one large sample estimating a median incidence of 52.1 per 100,000 people per year.11 Likewise, median survival after out-of-hospital cardiac arrest is estimated at 8.4%,11 but the range varies from several large U.S. cities that reported survival rates less than 2%12,13 to exemplary systems with survival over 16%.11 The incidence of cardiac arrest in the hospital is about 0.17 events per hospital bed per year.14 For inpatients experiencing cardiac arrest, survival to hospital discharge is estimated at 17%. Less than half of cardiac arrests occur in an intensive care unit (ICU) setting, and survival does not appear to be related to the location of collapse.15 As many as 17% of episodes of respiratory compromise in the hospital may progress to cardiac arrest.16
Demographic features of sudden cardiac death are similar to the characteristics of cardiovascular disease. Sudden cardiac death is more common in males than females both outside the hospital10 and in the hospital.14 However, the incidence of cardiac arrest is higher in women (6%) than in men (4.4%) who are admitted to the hospital for acute myocardial infarction (MI).17 Cardiac arrest outside the hospital affects blacks more than whites or Asians.10,12 In addition, cardiac arrest is more common in areas with lower socioeconomic status,18 and survival may be worse for individuals in regions with lower property values.19 While sudden death can affect patients of all ages, the mean age for sudden cardiac arrest is between 65 and 70 years in most studies.10,11,14
Two temporally and mechanistically separate processes contribute to mortality: cardiopulmonary collapse and neurologic injury. In evidence of the first process, only one-third of patients who collapse outside of the hospital have restoration of circulation long enough to be admitted to the hospital. Likewise, only 44% of patients who collapse in the hospital have return of circulation.14 In evidence of the second process, two-thirds of patients admitted to the hospital after out-of-hospital collapse20 and 60% of patients resuscitated from cardiac arrest in the hospital14 die prior to discharge from the hospital. The most common reason for death among patients admitted to the ICU after out-of-hospital cardiac arrest is postischemic brain injury, whereas multiple organ failure is more common for patients after in-hospital cardiac arrest.21 Failure to awaken contributes to withdrawal of care and in-hospital death for as many as 44% to 68% of patients after initial restoration of circulation.14,22
Restoring Circulation
Acute treatment of cardiac arrest consists of two concurrent, goal-directed activities: (1) artificial circulation (usually chest compressions augmented by peripheral vasoconstrictors) to circulate oxygenated blood to heart and brain and (2) electric shock to terminate ventricular fibrillation (VF) and unstable tachyarrhythmias. There is increasing recognition that continuous, uninterrupted chest compressions are critically important for restoring circulation.23 Electrical rescue shocks are used only when appropriate. Rescue shock is the only procedure for which interruption of artificial circulation is absolutely necessary and justified.
Continuous reassessment of the patient can be reduced to constant awareness of two parameters (Figure 33-1). The organization of the electrocardiogram (ECG) and the presence of pulses will prompt appropriate selection of therapy. The recommended division of time and prioritization of activities to accomplish these goals is depicted in Figure 33-2. All other activities, including medications and advanced airway maneuvers, are designed to supplement these two core activities. Optimization of resuscitation requires that any interruption in the two core activities, especially artificial circulation, be minimized.
The American Heart Association and European Resuscitation Council provide consensus scientific statements about the acute management of cardiac arrest.24 Those guidelines have detailed review of specific drugs and procedures. The following section provides an overview of airway management, circulation support, rescue shock for defibrillation, and drug therapy during cardiac arrest.
Airway and Ventilation
Obstruction of the airway can occur in any patient with impaired consciousness including cardiac arrest.25 If uncorrected, this obstruction prevents oxygenation and ventilation, leading to or perpetuating cardiopulmonary collapse. In patients who are comatose because of primary cardiac arrest, the airway usually is not patent. Animal models typically do not mimic ventilation through the human airway, because the most common research animals (dogs and swine) have straight oral-tracheal passages.
Agonal respirations occur after acute cardiac arrest for an additional 1 to 2 minutes.26 These respirations may confuse lay people, delaying recognition of cardiac arrest. It is unclear whether agonal respirations can generate sufficient ventilation to support life. The presence of gasping is associated with survival, but it also may be a surrogate marker for brief collapse-to-resuscitation intervals.27 Regardless, the amplitude and frequency of agonal respirations declines over 1 to 2 minutes, necessitating artificial ventilation for all patients requiring more than momentary resuscitation efforts.
Simple maneuvers can open the human airway. Extension of the neck (head tilt) and forward displacement of the mandible (chin lift) straightens and opens the pharynx. The tongue can be displaced from the posterior pharynx by insertion of an oropharyngeal airway. With these steps, positive-pressure ventilation can be provided using mouth-to-mouth or bag-valve-mask ventilation. A positive-pressure breath of as little as 400 mL in adults (6-7 mL/kg) delivered over 2 to 3 seconds will cause the chest to rise.28 Recent studies have indicated that hyperventilation or hyperexpansion of the chest can impair venous return and decrease circulation during resuscitation.29 In addition, minute ventilations that are smaller than those required for long-term support probably provide adequate gas exchange during cardiac arrest.
The optimal rate and timing for positive-pressure breathes during resuscitation in humans has not been established. The need for gas exchange must be balanced against the fact that interrupting chest compressions even for a few seconds can reduce coronary perfusion pressure (Figure 33-3).30 In swine, comparison of different ratios of chest compressions to ventilation suggests that 2 breaths per 50 chest compressions or more may be optimal for resuscitation.31 An extreme point of view is that chest compressions without any artificial ventilation may be sufficient to accomplish resuscitation in certain individuals.32 This position is contrary to early work demonstrating that the human airway collapses in most unconscious subjects, and that compressions alone are unable to provide ventilation.25 Recent studies in humans indicate that chest compressions generate tidal volumes that are likely less than the physiologic dead space.33 Nevertheless, passive insufflation of oxygen without positive-pressure ventilation has been reported to be beneficial in humans.34 As a compromise, some medical systems employ continuous chest compressions without pauses for positive-pressure ventilation. In these systems, positive-pressure breathes are delivered asynchronously by bag-mask during chest compressions, and the actual minute ventilation achieved is unknown. Therefore, the most recent guidelines recommend a ratio of 30 chest compressions to 2 ventilations, but even higher ratios or asynchronous ventilation may be optimal. Certainly, the duration of any pauses to deliver breaths must be minimized.
Waveform capnography is an extremely useful monitor during resuscitation, both for confirming ventilation and for monitoring adequacy of circulation. During cardiac arrest, end-tidal CO2 measurement is related to cardiac output and pulmonary blood flow.35 Therefore, CO2 levels may be very low (<10 mm Hg) at the onset of resuscitation. Adequate artificial circulation will cause CO2 levels to increase, and these levels may be used as a feedback to improve or modify chest compressions. Data from emergency department patients suggest that an end-tidal CO2 level greater than 15 to 16 mm Hg is associated with successful cardiac resuscitation.36,37 Conversely, end-tidal CO2 less than 10 mm Hg after 20 minutes of resuscitative efforts appears to confirm failure of resuscitation.38 However, drugs commonly used during resuscitation can disrupt the association between capnography readings and pulmonary blood flow. For example, epinephrine infusion reduces CO2 levels, and sodium bicarbonate infusion produces a transient but profound elevation of CO2 levels. An abrupt increase in end-tidal CO2 levels, usually to levels over 35 mm Hg, accompanies the return of spontaneous circulation. This finding may be useful for recognizing return of circulation, thereby minimizing any interruptions of chest compressions for pulse checks (Figure 33-4).
Airway Devices
A second difficulty with BVM ventilation is insufflation of the stomach.39 Excessive air in the stomach can promote emesis, and the abdominal distension may impair venous return and lung compliance.40 The esophagus prevents air entry into the stomach unless upper airway pressures exceed 15 to 20 cm H2O.41 However, esophageal muscle tone declines during cardiac arrest, allowing air to enter the stomach with upper airway pressures over 5 to 8 cm H2O.42 If the upper airway is not patent, providers may try to ventilate with increased pressure to achieve chest rise. Furthermore, rapid squeezing of the bag during the excitement of the situation results in too-high upper airway pressures. To avoid these problems, rescuers should emphasize gentle and well-paced inflation of the lungs during resuscitation.
Tracheal intubation can secure the airway definitively. A cuffed tracheal tube protects from emesis and maintains airway patency. However, laryngoscopy requires an interruption in chest compressions, and the tracheal tube by itself does not correct cardiac arrest. Observations of paramedics have documented extremely long interruption of chest compressions during “uncomplicated” tracheal intubation.43 Therefore, consideration should be made to delay tracheal intubation during initial resuscitation to reduce delays or interruptions of other life-saving interventions. After restoration of circulation, patients with coma or continued respiratory failure will require tracheal intubation.
Alternative supraglottic airway adjuncts such as double-lumen combination tracheal-esophageal tubes (e.g., Combi-tube), laryngeal tubes (e.g., King-LT), or laryngeal mask airways (LMA) can be used to temporarily manage the airway during resuscitation.44,45 These devices have the advantage that they can be inserted blindly in seconds without laryngoscopy and without interruption of chest compressions.39 The degree to which these devices can protect from aspiration is debated, but it is clear that they can allow adequate control of the airway to achieve resuscitation during cardiac arrest. Clinicians should strongly consider using these supraglottic airways as the first advanced airway during resuscitation because of the advantage of these devices for reducing interruptions in chest compressions.
Artificial Circulation
In the patient without pulses, circulation of blood can be accomplished by mechanical compression of the heart and chest. The critical parameter for restoring spontaneous circulation is the development of adequate coronary perfusion pressure (CPP). CPP is quantified by the pressure gradient between the aorta and the inside of the ventricles (usually approximated by the pressure in the right atrium or the central venous pressure [CVP]). Measurement of CPP in clinical practice is difficult unless the patient has invasive monitoring prior to cardiopulmonary collapse. CVP can be estimated from a central line, and peripheral arterial pressures developed approximate aortic pressures. In the spontaneously beating heart, most blood flows through the ventricular walls during diastole, when the ventricular pressure is lowest. With mechanical compression of the heart and chest during resuscitation, the primary perfusion of the heart occurs during the relaxation phase (see Figure 33-3). Therefore, CPP is usually measured at the end of the relaxation phase. CPP is highly correlated with myocardial perfusion, and consequently with the likelihood of resuscitation.46 In humans, return of circulation requires that the developed CPP exceed 15 to 20 mm Hg. It is likely that with CPP less than 15 mm Hg, perfusion is inadequate to replete the energy state of the myocardium during cardiac arrest.
Even brief interruptions in chest compressions can result in decreased CPP. This fact has clinical importance, as evidenced by the fact that more uninterrupted chest compression are highly associated with restoring circulation and survival.23 When chest compressions are measured during actual resuscitations by paramedics or hospital providers, interruptions and pauses are frequent.47,48 These observations have prompted the development of monitor-defibrillators with features to measure and record chest compressions, and even to provide real-time feedback to providers.49 These devices often employ an accelerometer in the defibrillation pads or in a separate attachment that is placed on the sternum of the patient. It is unknown if real-time feedback can improve resuscitation success, but it is clear that the continuity and quality of chest compressions are important parameters to maximize for resuscitation to succeed. Recent literature has used the chest-compression fraction to quantify this parameter (Figure 33-5).
Direct cardiac compression via a thoracotomy is more effective than external chest compressions, producing roughly threefold increases in CPP.50,51 This approach also allows recognition of cardiac tamponade and treatment by pericardiotomy. Mechanical activity and fibrillation are immediately visible, and electrical rescue shocks or pacing can be applied directly to the heart. In the setting of cardiopulmonary collapse due to exsanguination, thoracotomy also allows aortic compression to shunt blood to heart and brain, as well as direct control of intrathoracic bleeding. Until the 1960s, thoracotomy was the standard approach for treatment of sudden cardiac arrest, but this procedure has now been supplanted by closed-chest compressions. Cases series describe how this technique continues to be successful, and its use should be considered when closed-chest compressions are ineffective.50 Open-chest cardiac massage is most likely to succeed if initiated early during resuscitation.52
Delivery of chest compressions is often inadequate, and uninterrupted chest compressions are critical for restoration of circulation.23,30,53,54 A variety of mechanical devices have been developed to provide more consistent and continuous chest compressions.55 Some of these devices exploit circumferential compression or active compression/decompression of the chest to increase the efficiency of artificial circulation. Clinical trials comparing these devices have not demonstrated any benefit versus manual chest compressions, and one trial found a trend towards worse neurologic outcome.56 While no current device is poised to solve this challenge, the constant attention of industry to this area illustrates the need for strategies to improve delivery of chest compressions.
Extracorporeal perfusion for restoration of circulation is highly effective and can be used to resuscitate subjects for whom chest compressions have failed.57,58,59 With extracorporeal support, more time becomes available to address the primary cause for cardiac arrest. However, this approach requires specialized technical skill and has increased cost and risk. Logistical issues include limited availability of perfusion equipment, setup time for circuit priming, and delays in establishing adequate venous and arterial access. Development of portable cardiopulmonary bypass devices that can be primed quickly, along with improved techniques for rapid vascular access, could broaden the use of this technology. At present, this approach is used only in specialized centers that have devoted institutional resources to set up a dedicated program.
ECG Monitoring
VF and asystole lie along a continuum of not-organized ECG. Arbitrary peak-to-peak amplitude of the ECG is usually used to distinguish asystole (amplitude < 0.1-0.2 mV) from VF (amplitude > 0.2 mV).60 However, VF also exhibits temporal structure that may be absent in asystole.61 VF is a chaotic electrical activity formed by multiple interacting waves of activation within the heart.62 VF emerges from broken wavefronts that result from an area of ischemia (as in MI), an area of prolonged refractoriness (as in drug-induced or inherited prolonged QT intervals), or too-rapid succession of activation potentials (as in tachycardia or an “R on T” premature beat). As the organization and amplitude of these waves decline, because of ischemia or hypoxemia, the amplitude of the ECG also declines. Reperfusion of the heart in asystole may restore VF. Furthermore, the amplitude and organization of the VF increase with reperfusion, providing a marker of adequate artificial perfusion.
Rational Use of Rescue Shocks for Defibrillation
Delivery of immediate transthoracic electric (rescue) shocks to patients in VF can convert VF into an organized cardiac rhythm. Rescue shocks are highly effective when VF is of very brief duration (<1-2 minutes). These shocks may work by depolarizing the heart, canceling the original wavefronts, or by prolonging the refractory periods.62 Although rescue shocks can successfully restore an organized rhythm, repeated shocks may directly damage the myocardium. The precise magnitude of this damage is still unclear.63 Nevertheless, optimal therapy should provide rescue shocks at the lowest effective energy while minimizing the number of unsuccessful rescue shocks.
Rescue shocks are more likely to fail when cardiac arrest has lasted more than a few minutes. In the out-of-hospital setting when the collapse is not witnessed by the paramedic, only 9% to 12% of rescue shocks restore an organized ECG.64,65 Furthermore, resuscitation is less likely after rescue shocks that convert VF into asystole.66 In one model for defibrillation, a “critical mass” of the heart must be depolarized by a rescue shock to ensure that VF activation potentials are extinguished.62 If a critical mass is not defibrillated, chaotic activity in the remaining regions will spread throughout the heart, rekindling VF. However, even when the entire heart is depolarized by the shock, VF may recur, perhaps because of heterogeneous areas of refractoriness or persistent foci.67 Regardless, shocks must be of sufficient intensity to deliver depolarizing current to the majority of the heart.
Several maneuvers can facilitate electrical defibrillation. Multiphasic shock waveforms that produce more effective depolarization of individual myocytes (biphasic waveforms, for example) tend to accomplish defibrillation with less energy than monophasic waveforms.62 Consequently, most defibrillators available now deliver biphasic waveforms. Increased pressure of paddles from 0.5 to 8 kg on the chest will decrease transthoracic impedance by as much as 14%, increasing delivery of current to the heart.68,69 This advantage of paddles must be weighed against the increased safety and convenience afforded by hands-free self-adhesive defibrillation pads, and most settings now have adopted the hands-free pads. In the past, multiple shocks would be delivered in rapid succession to decrease chest impedance. However, repetitive shocks decrease chest impedance only about 8% or less in actual patients,68,70,71 which does not justify the interruption of artificial circulation to deliver “stacked” shocks. Minimal interruption of chest compressions to deliver a single shock, followed by immediate resumption of chest compressions, is now recommended. Reducing the delay from the last chest compression to the delivery of the rescue shock has also been associated with greater resuscitation success.72 Therefore, chest compressions should continue while the defibrillator is charging and only stop at the last moment prior to shock. Rescue shocks of sufficient energy with multiphasic waveforms should be delivered singly to the patient in VF using firm paddle pressure on the chest or hands-free self-adherent pads.
For VF that has lasted more than a 3 to 4 minutes, preclinical data suggest that delaying rescue shocks until after a few minutes of chest compressions will improve rescue shock success. In animals, reperfusion prior to rescue shocks appears to be preferable to immediate rescue shock after more than 5 minutes of untreated VF.73–76 To date, two clinical studies in out-of-hospital cardiac arrest have found that either 90 seconds or 3 minutes of chest compressions prior to delivery of the initial rescue shock improved resuscitation rates for subjects with VF outside the hospital, particularly when rescuer response intervals are longer than 4 minutes.2,77 However, a third study found no difference in outcomes with 5 minutes of chest compressions prior to shock,78 while a fourth study found no difference in outcomes with 3 minutes of chest compressions prior to shock.79 Finally, a large multicenter trial comparing immediate rescue shock to 3 minutes of chest compressions prior to rescue shock recently stopped enrollment, reportedly finding no difference between groups.80 Taken together, the clinical data suggest that the first rescue shock for VF should be delivered as soon as possible within 3 to 5 minutes as long as chest compressions are started immediately, but that there is no reason to intentionally delay the rescue shock.
Quantitative analysis of the VF waveform can distinguish early VF from late VF and may be useful for estimating the likelihood of rescue shock success.81 Larger amplitude of VF suggests early VF and is associated with more successful resuscitation.82 However, amplitude can be affected by body habitus and other recording conditions. Frequency-based measures, as well as nonlinear dynamical measures, also can be used to quantify VF and estimate the probability of rescue shock success and are less dependent on recording conditions.83–86 All these measures, or some combination of these measures, are likely to be implemented in future generations of defibrillators. These devices may provide real-time, semiquantitative estimates of the probability that a rescue shock will succeed in restoring an organized rhythm. Using this information, the clinician will be able to choose to shock VF when the probability of shock success is high, or to concentrate on improving the situation with artificial perfusion when the probability of shock success is low.
Drug Therapy
All drug therapy in cardiac arrest can be divided into three categories: pressors, antidysrhythmics, and metabolic drugs. There is good evidence that pressors improve artificial circulation, making restoration of pulses more likely. However, recent data in out-of-hospital cardiac arrest accentuate the point that no drug therapy has been demonstrated to improve long-term survival.87 Antidysrhythmic drugs are effective for preventing dysrhythmias and therefore have a role in stabilizing the heart once circulation is restored. The value of antidysrhythmic drugs for terminating VF or reversing asystole is less clear. Metabolic drugs, primarily bicarbonate, can be used to reverse acidosis or other electrolyte problems when they are recognized. However, there are no data to support routine use of these drugs for all patients.
Pressors used during resuscitation include epinephrine and vasopressin. Both of these drugs can increase CPP via actions on α-adrenergic (epinephrine) or vasopressin receptors (Figure 33-6).88,89 Epinephrine is usually administered in 1-mg (~0.015 mg/kg) increments. In laboratory studies, the pressor effects of epinephrine during cardiac arrest are brief (~5 minute). Vasopressin has been administered as 40-unit boluses (~0.5 units/kg) and produces a longer-lasting increase in CPP (~10 minutes). Both drugs should be titrated to improvement in clinical indicators (ECG waveform, mechanical activity, changes in end-tidal CO2 or CPP). Direct comparisons between vasopressin and epinephrine have failed to demonstrate a clear superiority of one drug over the other or of the combination of the two over either alone.90–93
The 1-mg dose of epinephrine may be too small to restore pulses after circulatory arrest lasting more than a few minutes. However, trials in out-of-hospital patients comparing higher initial boluses of epinephrine (15 mg versus 1 mg) found a higher rate of restoration of pulses (13% versus 8%) and admission to the hospital (18% versus 10%),94 but overall survival was not different. Comparison of a lower dose (7 mg versus 1 mg) of epinephrine in both in-hospital and out-of-hospital cardiac arrest found no change in restoration of pulses or survival.95 Likewise, comparison of 0.02 mg/kg versus 0.2 mg/kg epinephrine found no change in restoration of pulses, or survival.96
It is possible that the β-adrenergic effect of these higher doses of epinephrine produces toxicity that limits long-term survival. Postarrest impairment of cardiac index and oxygen delivery has been related to epinephrine dose.97 Likewise, neurologic impairment has been related to epinephrine dose.98 No trial has completed a direct comparison of epinephrine with more selective α-adrenergic agents such as phenylephrine. However, one trial found no advantage from administration of 11 mg of norepinephrine.94
Vasopressin can increase CPP without complicating β-adrenergic effects. Resuscitation rates and survival are identical for patients resuscitated with vasopressin and standard doses of epinephrine after in-hospital90 or out-of-hospital cardiac arrest.91 However, post hoc analyses suggest vasopressin may be superior for resuscitation and survival of patients whose first ECG rhythm is asystole and for those subjects requiring multiple doses of vasopressors.91 Subsequent studies examined the combination of epinephrine with vasopressin versus epinephrine alone for treatment of cardiac arrest.92,93 These studies found no difference in outcomes with the combination of drugs. Therefore, use of either vasopressin or epinephrine is justified in the setting of cardiac arrest. None of the studies on these pressors standardized post–cardiac arrest care, and therefore all are limited for assessing drug effects on neurologic recovery.
Although preclinical and existing clinical data support the conclusion that vasoactive drugs during CPR can increase the probability of restoring spontaneous circulation, it is unclear whether these drugs actually improve overall survival. A recent trial in out-of-hospital cardiac arrest compared resuscitation without intravenous (IV) drugs to resuscitation with IV drugs.87 In this study, which accounted for many aspects of post–cardiac arrest care, IV drugs clearly increased the rate of restoration of pulses (32% versus 21%), but the rate of neurologically good survival did not differ (9.8% versus 8.1%). It is possible the study was underpowered to detect differences in long-term outcomes. However, the data raise the worrisome possibilities that when IV drugs are required to restore cardiac activity, severe brain injury has already occurred, or even that the drugs used add to brain injury.98
The role of antidysrhythmic drugs during cardiac arrest is equivocal.99,100 Atropine may relieve bradycardia when it is vagally mediated. However, nervous system influences on the heart are largely eliminated after more than 1 to 2 minutes of circulatory arrest. Therefore, there is little expectation that atropine will improve resuscitation from asystole or PEA. Lidocaine, procainamide, and bretylium have a long history of use in the treatment of VF. The basis for this use is principally the observation that these drugs can suppress dysrhythmias prior to cardiac arrest. Once VF is established, lidocaine can actually increase the electrical energy required to defibrillate by more than 50%.101 This effect is not true for agents that are less potent, as sodium channel antagonists. For example, administration of amiodarone (5 mg/kg) is superior to placebo102 and to lidocaine103 for restoration of pulses to out-of-hospital patients with VF that is not terminated by three rescue shocks. These studies did not control subsequent critical care and are thus not designed to determine any effect on long-term survival. In summary, antidysrhythmic drugs are commonly used during resuscitation, but only amiodarone use has supporting human data.
Empirical treatments of metabolic disturbances during cardiac arrest are not supported by prospective human data. Bicarbonate or other buffers may improve acidemia resulting from ischemia, and systems with increased use of bicarbonate report higher rates of successful resuscitation.104 However, no trial has demonstrated that sodium bicarbonate administration improves outcome.105,106 Aminophylline has been proposed as an antagonist of adenosine released during ischemia. Adenosine is hypothesized to suppress cardiac electrical activity. Two prospective studies of aminophylline administration to subjects with PEA or asystole failed to demonstrate any improvement in resuscitation.107,108 Use of dextrose-containing fluids versus dextrose-free fluids did not alter outcome for out-of-hospital cardiac arrest.109 Other metabolic therapies including calcium and magnesium also lack supporting data.110,111 However, it is appropriate to consider specific use of these agents to correct known abnormalities that are contributing to cardiac arrest, such as known hyperkalemia, calcium channel blocker overdose, torsades, or hypomagnesemia.
Aspects of Cardiac Arrest in Specific Situations
The original etiology of cardiac arrest may not be known during acute resuscitation. However, if this information is available, treatment and prognosis can be individualized to the specific patient. Among out-of-hospital patients, as many as 66% have primary cardiac disturbances.112 For in-hospital patients experiencing cardiac arrest, dysrhythmia and cardiac ischemia account for 59% of events.14 This section reviews unique features of cardiac arrest resulting from both cardiac and noncardiac causes.
Primary Cardiac Events
Cardiac arrest is most commonly attributable to cardiac disease. A primary dysrhythmia or cardiogenic shock is the most common proximate cause of cardiac arrest.112,113 Patients undergoing angioplasty have 1.3% incidence of cardiac arrest, and survival in these patients resembles survival in other populations.114 Among patients admitted to the hospital with acute MI, cardiac arrest occurs in 4.8%.17 Dysrhythmias are common during the hours after reperfusion therapy,114 although reperfusion therapy reduces the overall risk of cardiac arrest.115 During acute MI, cardiac arrest is most likely in patients with lower serum potassium levels, more than 20 mm of total ST elevation, and a prolonged QTc interval during the first 2 hours of their event.115 With a mean follow up of 43 months, 3.3% of subjects surviving acute MI suffered sudden cardiac death.116 Abnormalities of the heart are present in most cases of cardiac arrest, with coronary artery disease present in at least 65% of autopsies.117 Taken together, these data suggest that most patients with cardiac arrest will have contributing cardiovascular disease.
An acute coronary syndrome is present in more than half of patients presenting with primary cardiac arrest outside of the hospital. When angiography was performed on consecutive patients resuscitated from cardiac arrest, coronary artery occlusion was identified in 48%.118 Similarly, 51% of initially resuscitated outpatients exhibited cardiac enzyme elevation or ECG evidence of acute MI.119 In one series, troponin T was elevated in 40% of out-of-hospital patients undergoing CPR, regardless of whether circulation was restored.120 The direct myocardial injury from defibrillation and CPR may cause spurious elevations of creatine kinase that are unrelated to cardiovascular disease.121 However, cardiac troponin elevations are believed to reflect acute MI rather than injury from electric shocks.122 Thus the 40% of subjects undergoing CPR with elevated troponin probably suffered myocardial injury prior to collapse.
The high likelihood of an acute coronary syndrome in the patient suffering cardiac arrest should prompt consideration of antiplatelet therapy, anticoagulation, beta-blockade, and nitrates during the post–cardiac arrest care. Unless a clearly noncardiac etiology for cardiac arrest is evident, acute coronary angiography may reveal an indication for angioplasty, thrombolysis, or other reperfusion therapy. Early angioplasty or reperfusion therapy is associated with improved survival and outcome.17,118,123,124 Primary angioplasty is safe in comatose patients undergoing hypothermia treatment, and good outcomes have been reported.125,126 Therefore, coma and its treatment should not delay emergent treatment of acute coronary syndromes if they are suspected.
Primary ventricular tachyarrhythmias are rapidly reversible and may be more likely than PEA or asystole for patients with a primary cardiac cause of collapse. Ventricular tachyarrhythmias are the initially recorded rhythm in 23% to 41% of out-of-hospital cardiac arrests10,11,127 and in 25% of in-hospital cardiac arrests.14 Because VF is rapidly reversible, patients with this rhythm comprise the majority of survivors of cardiac arrest. Data collected over 3 decades in one city noted that the prevalence of VF in out-of-hospital cardiac arrest has declined since 1978.10 This trend may reflect a change in preventive medicine or in the epidemiology of cardiovascular disease over time. Once defibrillation is accomplished, prophylactic treatment of ventricular dysrhythmias is usually not required and may be harmful, given the side effects of most drugs. However, recurrent malignant dysrhythmias may be treated with infusions of lidocaine, amiodarone, procainamide, or other antidysrhythmics while searching for and treating the underlying etiology. Current practice most often employs amiodarone or lidocaine.
Long-term antidysrhythmia treatment should be considered for patients who survive sudden cardiac arrest. At a minimum, treatment should be considered for patients with decreased left ventricular function or primary dysrhythmia without a reversible cause.128 Importantly, subjects surviving a life-threatening ventricular dysrhythmia had a 15% to 20% risk of death during a mean of 16 months of follow-up, even when a reversible cause of the dysrhythmia such as electrolyte disturbance or hypoxia can be identified.129 Implantable defibrillators have been found to be superior to antidysrhythmic drugs for reducing the risk of subsequent death.130 This benefit is primarily in subjects with a left ventricular ejection fraction (LVEF) less than 0.35.131 Implantable defibrillators were not better than antidysrhythmic drugs in a European trial that enrolled subjects resuscitated from cardiac arrest secondary to ventricular dysrhythmia without regard to LVEF.132 Nevertheless, these devices offer significant hope of preventing sudden cardiac death, and identification of patients they may benefit is an active area of research. At present, implantable defibrillators should be discussed for patients who recover from coma with LVEF less than 0.35 or survive a ventricular arrhythmia in the absence of clearly reversible causes.
Asphyxia
Asphyxia-induced cardiac arrest can result from drowning, choking, asthma, progressive respiratory failure with hypoxemia, or traumatic coma with hypoventilation. Acute asphyxia causes transient tachycardia and hypertension, followed by bradycardia and hypotension, progressing to PEA or asystole. This period of blood flow with severe hypoxemia prior to cardiac arrest may make asphyxiation a more severe injury than VF or other rapid causes of circulatory arrest.133 Brain edema is more common on CT scans after resuscitation when cardiac arrest was caused by pulmonary rather than cardiac etiologies.134
During cardiac arrest, pulmonary edema develops from redistribution of blood into the pulmonary vasculature.135 Thus, oxygenation is only worsened in the asphyxiated patient. Attention to the primary cause of asphyxia, as well as to maneuvers that will increase oxygenation (e.g., increased end-expiratory pressure or increased inspiration to expiration time ratios), may be necessary.
Pulmonary Embolism
Pulmonary emboli may occur in the postsurgical patient, as well as in medical patients with impaired mobility.136 In one series, pulmonary emboli were present in 10% of in-hospital deaths,137 and the prevalence among out-of-hospital deaths was similar.138 Pulmonary emboli can result in rapid cardiopulmonary collapse and should be considered as a possible etiology of cardiac arrest in the proper clinical setting or when collapse is preceded by sudden shortness of breath, hypoxemia and/or pleuritic chest pain.
Administration of bolus fibrinolytic drugs (tissue plasminogen activator, streptokinase, or urokinase) has been speculated to help acutely during resuscitation of a patient with a suspected pulmonary embolism. Smaller pulmonary emboli can lead to cardiac arrest due to hypoxemia, and resuscitation may be possible prior to fibrinolysis if adequate oxygen exchange can be restored. Tenecteplase has been used with reported success in non-randomized trials during resuscitation of undifferentiated patients,139 but it failed to demonstrate benefit in a larger randomized trial of undifferentiated patients in cardiac arrest.140 Likewise, a randomized trial of tissue plasminogen activator to patients with out-of-hospital cardiac arrest and an initial rhythm of PEA failed to demonstrate any benefit, although drug administration was late during resuscitation.141 There are few data about treatment of cardiac arrest resulting from massive pulmonary emboli, and certainly the overall prognosis in this setting is dismal. Individual decisions to use fibrinolytics in this setting must be tempered by the low probability of success.
Electrolyte Disturbances
Potassium disturbances are the most likely electrolyte disturbance to result in cardiac arrest. In cardiac patients, hypokalemia has been linked to the incidence of VF after MI.115,142 Hypokalemia also may account for the increased incidence of sudden death in patients taking large doses of diuretics. VF is rare in patients where serum potassium is maintained over 4.5 mEq/L. Conversely, hyperkalemia can prolong repolarization increasing the likelihood of VF initiation. Hyperkalemia may also suppress automaticity in the myocardial electrical system, leading to bradycardic PEA or asystole. Interestingly, cardiac arrest occurring during hemodialysis is not associated with high or low potassium levels but is more common when patients are dialyzed against a low (0 or 1 mEq/L) potassium dialysate.143 These data suggest that rapid changes in potassium rather than the absolute value are important triggers of cardiac arrest in this population. Derangements of calcium and magnesium may produce similar or synergistic changes in cardiac conduction.
Poisoning
Cardiac arrest can result from drug overdose. Therapy does not change except when specific antidotes or countermeasures to the poison are available. For example, calcium channel blocker overdose may be countered by administration of IV calcium.144 Beta-blocker toxicity may require large doses of inotropic agents145 or may respond to glucagon.146 Digoxin overdose may respond to digoxin-binding antibodies.147 In the case of narcotic-induced respiratory depression, subsequent cardiac arrest usually is a specific case of asphyxia rather than specific cardiotoxic effects. Case reports have suggested treating local anesthetic toxicity with 1 to 3 mL/kg of Intralipid.148 This intervention is likely to be explored for other lipid-soluble poisonings.149 One principle of poisonings is that the patient was often healthy prior to the event, and may recover well once the poison is eliminated. This potential for a better outcome may justify longer and more aggressive efforts at resuscitation.
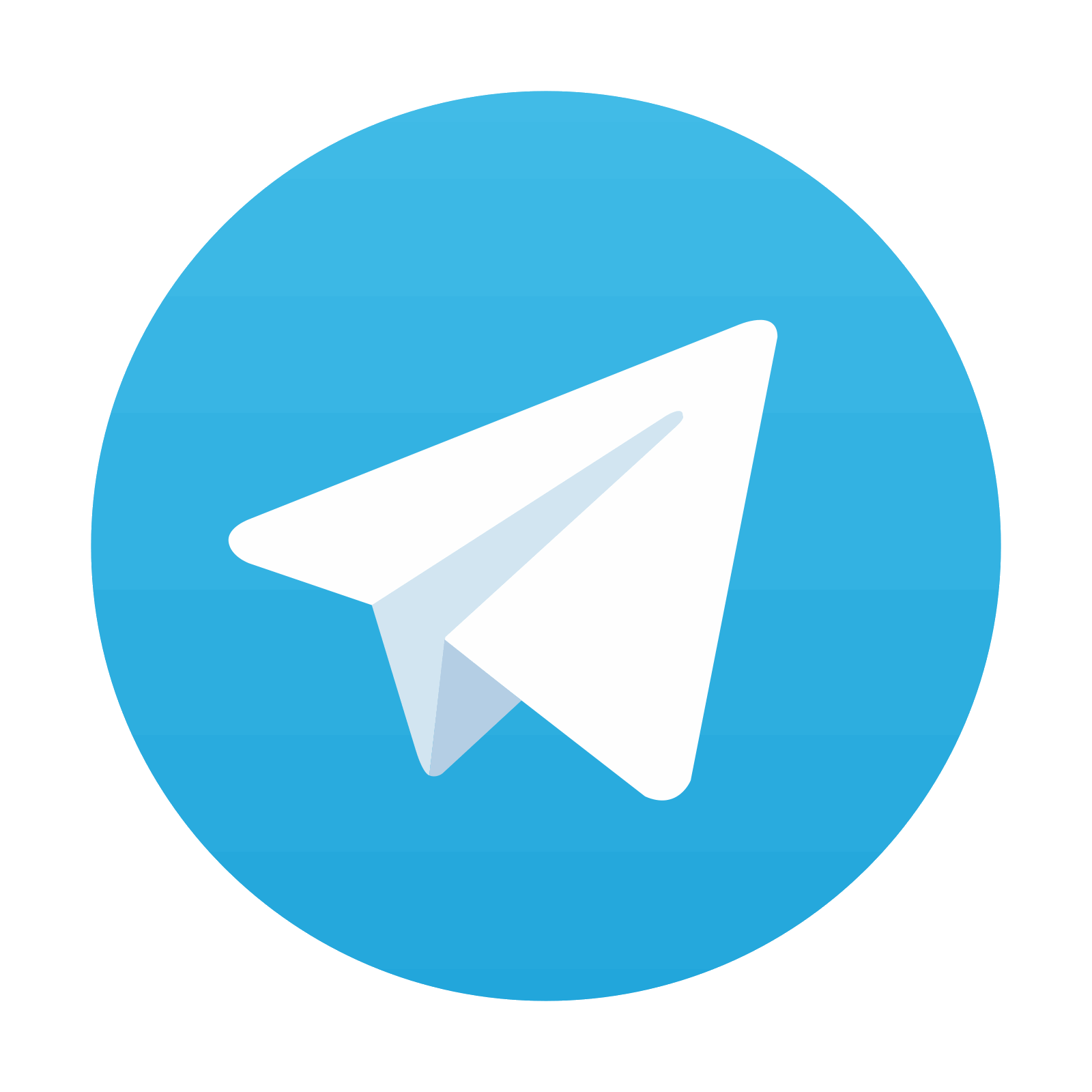
Stay updated, free articles. Join our Telegram channel
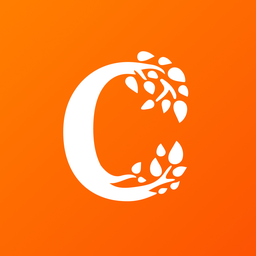
Full access? Get Clinical Tree
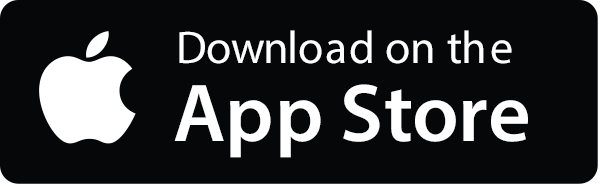
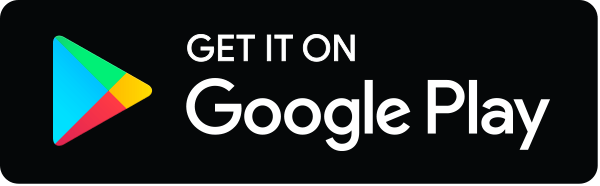