Xenobiotics are compounds that are foreign to a living system. Toxic xenobiotics interfere with critical metabolic processes, cause structural damage to cells, or alter cellular genetic material. The specific biochemical sites of actions that disrupt metabolic processes are well characterized for many xenobiotics although mechanisms of cellular injury are not. This chapter reviews the biochemical principles that are relevant to an understanding of the biotransformation enzymes and their clinical implications and the damaging effects of toxic xenobiotics.
The capacity of a xenobiotic to produce injury is affected by many factors, including its absorption, distribution, elimination, genotypic/phenotypic states, synergistic/antagonistic coingestants, site of activation or detoxification, and site of action. This section focuses on how the route of exposure (absorption) and the ability to cross membranes in order to access particular organs (distribution) affect toxicity.
The route of exposure to a xenobiotic often confines damage primarily to one organ, for example, pulmonary injury that follows inhalation of an irritant gas or gastrointestinal (GI) injury that follows ingestion of a caustic. However, most xenobiotics have lipophilic properties that facilitate absorption across cell membranes of organs that are portals of entry into the body: the skin, GI tract, and lungs. Systemically circulating xenobiotics, such as cyanide or carbon monoxide, affect toxicity in multiple organs. The liver is particularly susceptible to xenobiotic-induced injury as it receives blood supply from both the portal venous system and from the hepatic artery containing xenobiotics absorbed from multiple sites of exposure.
Once systemically absorbed, various factors affect the distribution of a xenobiotic to a particular organ, including its molecular weight, protein binding, plasma pH/drug pKa, lipophilicity, in addition to the presence of membrane transporters, and physiologic barriers (Chap. 9). Restriction of distribution or preferential distribution into a target organ determines the ability of a xenobiotic to mediate tissue injury. For example, many potentially toxic xenobiotics fail to produce central nervous system (CNS) injury because they cannot cross the blood–brain barrier. The negligible CNS effects of the mercuric salts when compared with organic mercury compounds are related to their relative inability to penetrate the CNS. With respect to target organs, 2 potent biologic xenobiotics—ricin (from Ricinus communis) and α-amanitin (from Amanita phalloides)—block protein synthesis through the inhibition of RNA polymerase. However, they result in different clinical effects because they access different tissues. Ricin has a special binding protein that enables it to gain access to the endoplasmic reticulum in GI mucosal cells, where it inhibits cellular protein synthesis and causes severe diarrhea. α-Amanitin is transported into hepatocytes by bile salt transport systems, where inhibition of protein synthesis results in cell death. Finally, the electrical charge of a xenobiotic at tissue pH affects its ability to enter a cell. Unlike the ionized (charged) form of a xenobiotic, the un-ionized (uncharged) form is often lipophilic and passes through lipid cell membranes to enter the cells. The pKa of an acidic xenobiotic (HA⇌A– + H+) is the pH at which 50% of the molecules are ionized (A– form) and 50% is un-ionized (HA form). A xenobiotic with a low pKa is more likely to be absorbed in an acidic environment in which the un-ionized form predominates. This conept is the basis for urine alkalinization and ion trapping in the management of salicylate toxicity (Chap. 37).
The capability to detoxify and eliminate both endogenous toxins and xenobiotics is crucial to the maintenance of physiologic homeostasis. A simple example is the detoxification of cyanide, a potent cellular poison that is common in the environment and is also a product of normal metabolism. Mammals have evolved to have the enzyme rhodanese, which combines cyanide with thiosulfate to create the less toxic, renally excreted compound thiocyanate.7
The liver has the highest concentration of enzymes that metabolize xenobiotics. Enzymes found in the cytosol of hepatocytes that are specific for alcohols, aldehydes, esters, or amines act on many different substrates within these broad chemical classes. Enzymes that act on more lipophilic xenobiotics, including the cytochrome enzymes, are embedded in the lipid membranes of the cytosol-based endoplasmic reticulum. Cytochromes are a class of hemoprotein enzymes whose function is electron transfer, using a cyclical transfer of electrons between oxidized (Fe3+) or reduced (Fe2+) forms of iron. One type of cytochrome is cytochrome P450 (CYP) whose nomenclature derives from the spectrophotometric characteristics of its associated heme molecule. When bound to carbon monoxide, the maximal absorption spectrum of the reduced CYP (Fe2+) enzyme occurs at 450 nm.79 These CYP enzymes commonly split the 2 oxygen atoms of an oxygen molecule, incorporating one into the substrate and one into water and thus are called mixed-function oxidases or monooxygenases (Fig. 11–1). This differs from dioxygenases that incorporate both oxygen atoms into the substrate.79,108
Microsomes are the pieces of the endoplasmic reticulum that result when cells are disrupted. When cells are mechanically disrupted and centrifuged, these membrane-bound enzymes are found in the pellet, or microsomal fraction; hence, they are called microsomal enzymes. Enzymes located in the liquid matrix of cells are called cytosolic enzymes and are found in the supernatant when disrupted cells are centrifuged.22
The study of xenobiotic metabolism was established as a scientific discipline by the seminal publication of Williams in 1949.120 Biotransformation is the physiochemical alteration of a xenobiotic, usually as a result of enzyme action. Most definitions also include that this action converts lipophilic substances into more polar, excretable substances.70,108 Most xenobiotics undergo some biotransformation, the degree of which is affected by their chemical nature. The hydrophilic nature of ionized compounds such as carboxylic acids enables the kidneys to rapidly eliminate them. Very volatile compounds, such as enflurane, are expelled promptly via the lungs. Neither of these groups of xenobiotics undergo significant enzymatic metabolism.
Biotransformation usually results in “detoxification,” a reduction in the toxicity, by the conversion to hydrophilic metabolites of the xenobiotic that can be renally eliminated.70 However, this is not always the case. Many parent xenobiotics are inactive and must undergo “metabolic activation.”72 When metabolites are more toxic than the parent xenobiotic, biotransformation is said to have resulted in “toxification.”108 Biotransformation via acetylation or methylation enhances the lipophilicity of a xenobiotic. Biotransformation is accomplished by impressively few enzymes, reflecting broad substrate specificity. The predominant pathway for the biotransformation of an individual xenobiotic is determined by many factors, including the availability of cofactors, changes in the concentration of the enzyme caused by induction, and the presence of inhibitors. The predominant pathway is also affected by the rate of substrate metabolism, reflected by the Km (Michaelis–Menten dissociation constant) of the biotransformation enzyme (Chap. 9).108
Biotransformation is often divided into phase I and phase II reactions, terminology first introduced in 1959.120 Phase I reactions prepare lipophilic xenobiotics for the addition of functional groups or actually add the groups, converting them into more chemically reactive metabolites. This is usually followed by phase II synthetic reactions that conjugate the reactive products of phase I with other molecules that render them more water soluble, further detoxifying the xenobiotics and facilitating renal elimination. However, biotransformation often does not follow this stepwise process.52 Some xenobiotics undergo only a phase I or a phase II reaction prior to elimination. Additionally, phase II reactions can precede phase I. While virtually all phase II synthesis reactions cause inactivation, a classic exception is fluoroacetate being metabolized to fluorocitrate, a potent inhibitor of the citric acid (tricarboxylic acid, or Krebs) cycle (Chap. 113).90
Biotransformed xenobiotics cannot be eliminated until they are moved back across cell membranes and out of the cells. Membrane transporters are proteins that move endobiotics/xenobiotics across the membranes without altering their chemical compositions Most membrane transporters are in the adenosine triphosphate binding cassette (ABC) family of transmembrane proteins that use energy from adenosine triphosphate (ATP) hydrolysis to move xenobiotics.14,45,52 This family includes the P-glycoprotein family and the glutathione S-conjugate export pump.103 The process of transport is sometimes termed a “phase III reaction.” However, membrane transport does not always occur after phase I or II reactions, and some parent compounds, such as digoxin, are transported across membranes without any biotransformation at all.25
Oxidation is the predominant phase I reaction, adding reactive functional groups suitable for synthetic conjugation during phase II. These groups include hydroxyl (–OH), sulfhydryl (–SH), amino (–NH2), aldehyde (–CHO), or carboxyl (–COOH) moieties. Noncarbon elements such as nitrogen, sulfur, and phosphorus are also oxidized in phase I reactions. Other phase I reactions include hydrolysis (the splitting of a large molecule by the addition of water that is divided among the 2 products), hydration (incorporation of water into a complex molecule), hydroxylation (the attachment of –OH groups to carbon atoms), reduction, dehalogenation, dehydrogenation, and dealkylation.70,108
The CYP enzymes are the most numerous and important of the phase I enzymes. A common oxidation reaction catalyzed by CYP enzymes is illustrated by the hydroxylation of a xenobiotic R–H to R–OH (Fig. 11–1).30 Membrane-bound flavin monooxygenase (FMO), a nicotinamide adenine dinucleotide phosphate (NADPH)-dependent oxidase located in the endoplasmic reticulum, is an important oxidizer of amines and other compounds containing nitrogen, sulfur, or phosphorus.70
The alcohol, aldehyde, and ketone oxidation systems use predominantly cytosolic enzymes that catalyze these reactions using nicotinamide adenine dinucleotide (NADH/NAD+), but alcohol oxidation can also occur in microsomes via CYP enzymes and in peroxisomes via the enzyme catalase (Fig. 11–2).65,108,123 Two classic phase I oxidation reactions are the metabolism of ethanol to acetaldehyde by alcohol dehydrogenase (ADH) followed by the metabolism of acetaldehyde to acetic acid by aldehyde dehydrogenase (ALDH). Alcohol dehydrogenase, which oxidizes many different alcohols, is found in the liver, lungs, kidneys, and gastric mucosa.65 Variations in these enzymes have clinical implications. For example, young women have less ADH in their gastric mucosa than young men. This results in decreased first-pass metabolism of ethanol and increased ethanol absorption. Some populations, particularly Asians, are deficient in ALDH, resulting in increased acetaldehyde concentrations and symptoms (Chap. 78).65
FIGURE 11–2.
The conversion of ethanol to acetaldehyde by three pathways. In the microsomal ethanol oxidizing system (MEOS), CYP2E1 uses nicotinamide adenine dinucleotide phosphate (NADPH) and oxygen for the conversion. In the cytosol, alcohol dehydrogenase uses NAD+. In peroxisomes, catalase uses H2O2. This illustrates how NAD+ and NADPH can function in oxidation reactions in both their oxidized and reduced forms. Alcohol dehydrogenase has a low Km for ethanol and is the predominant metabolic enzyme in moderate drinkers. (Adapted with permission from Zakhari S: Overview: how is alcohol metabolized by the body? Alcohol Res Health 2006;29(4):245-254.)

Biotransformation often results in the oxidation or reduction of carbon. A substrate is oxidized when it transfers electrons to an electron-seeking (electrophilic or oxidizing) molecule, leading to reduction of the electrophilic molecule. These oxidation-reduction reactions are usually coupled to the cyclical oxidation and reduction of a cofactor, such as the pyridine nucleotides, NADH/NAD+, or NADPH/NADP+. The nucleotides alternate between their reduced (NADPH, NADH) and oxidized (NADP+, NAD+) forms. Because xenobiotic oxidation is the most common phase I reaction, the newly created reduced cofactors must have a place to unload their electrons; otherwise, biotransformation ends. The electron transport chain serves as the major electron recipient.
Electrons resulting from the catabolism of energy sources are extracted primarily by NAD+, forming NADH. Within the mitochondria, NADH transports its electrons to the cytochrome-mediated electron transport chain. This results in the production of ATP, the reduction of molecular oxygen to water, and the regeneration of NAD+—all parts critical to the maintenance of oxidative metabolism. Nicotinamide adenine dinucleotide phosphate, generated by the hexose monophosphate shunt, is used in the synthetic (anabolic) reactions of biosynthesis (especially fatty acid synthesis). Nicotinamide adenine dinucleotide phosphate is also the cofactor in the reduction of glutathione, a molecule vital to the protection of cells from oxidative damage.
Biotransformation often results in the oxidation or reduction of carbon. The oxidation state of a specific carbon atom is determined by counting the number of hydrogen and carbon atoms to which it is connected. The more reduced a carbon, the higher the number of connections. For example, the carbon in methanol (CH3OH) has 3 carbon–hydrogen bonds and is more reduced than the carbon in formaldehyde (H2C=O), which has two. Carbon–carbon double bonds count as only one connection.
Cytochrome enzymes perform many functions. The biotransformation CYP enzymes are bound to the lipid membranes of the smooth endoplasmic reticulum. They execute 75% of all xenobiotic metabolism and most phase I oxidative biotransformations of xenobiotics.39 A second role for CYP enzymes is synthetic: biotransforming endobiotics (chemicals endogenous to the body) to cholesterol, steroids, bile acids, fatty acids, prostaglandins, and other important lipids. Cytochromes also act within the mitochondrial electron transport chain.41,78
Although more than 6,000 CYP genes exist in nature, the human genome project determined that the number of human CYP genes was 57.78 Cytochrome P450 enzymes are categorized according to the similarities of their amino acid sequences. They are in the same “family” if they are more than 40% comparable and same “subfamily” if they are more than 55% similar.77 Families are designated by an Arabic numeral (n), subfamilies by a capital letter (X), and each individual enzyme by another numeral (m), resulting in the nomenclature CYPnXm for each enzyme. For example, CYP3A4 is enzyme number 4 of the CYP3A subfamily of the CYP3 family.74 Genes coding for these enzymes contain sequence variations, designated by italicized style and an asterisk followed by an Arabic numeral (ie, CYP2D6*4), that lead to functional differences.49,74 An allele is a form of a gene that often arises through mutation. If the same gene variation exists in greater than 1% of the human population, then the allele is termed a genetic polymorphism. Most xenobiotic metabolism is done by the CYP1, CYP2, and CYP3 families, with a small amount done by the CYP4 family.13,117 Although 15 CYP enzymes metabolize xenobiotics,86 nearly 90% is done by 6 CYP enzymes: 1A2, 2C9, 2C19, 2D6, 2E1, and 3A4 (Table 11–1).78
CYP Enzyme | 1A2 | 2B6 | 2C9 | 2C19 | 2D6 | 2E1 | 3A4 |
---|---|---|---|---|---|---|---|
Percent of liver CYPs | 4%–16% | 2%–5% | 5%–29% | 1%–4% | 1%–4% | 6%–17% | 15%–37% |
Contribution to enterocyte CYPs | None | None | Minor | Minor | Minor | Minor | 70% |
Organs other than liver with enzyme | Lung | Kidney | Small intestine, nasal mucosa, heart | Small intestine, nasal mucosa, heart | Small intestine, kidney, lung, heart | Lung, small intestine, kidney | Much in small intestine; some in kidney, nasal mucosa, lung, stomach |
Percent of metabolism of typically used pharmaceuticals | 9% | 7% | 13% | 7% | 20% | 3% | 30 % |
Polymorphismsa | No | Yes | Yes | Yes | Yes | No | No |
Allelic Frequency | |||||||
Decreased Activity | |||||||
African American | 38%–62% | 0%–3% | 10%–17% | 14%–30% | |||
Asian | — | 14%–25% | 2%–8% | 25%–39% | 47%–94% | — | — |
Caucasian | 23%–39% | 16%–23% | 6%–16% | 31%–45% | |||
Increased Activity | |||||||
African American | 0%–25% | 15%–27% | |||||
Asian | — | 5%–15% | — | 0%–2% | 1% | — | — |
Caucasian | 6% | 21%–25% | 1%–9% | ||||
Ethiopian | 30% |
Most CYP enzymes are found in the liver, where they comprise 2% of the total microsomal protein.86 High concentrations are also found in extrahepatic tissues, particularly the GI tract and kidney.26,80 The lungs,126 heart,84 and brain27 have the next highest amounts. Each tissue has a unique profile of CYP enzymes that determines its sensitivity to different xenobiotics.26 The CYP enzymes in the enterocytes of the small intestine actually contribute significantly to “first-pass” metabolism of some xenobiotics.56,78 Corrected for tissue mass, the CYP enzyme system in the kidneys is as active as that in the liver. The activity of the renal CYP enzymes is decreased in patients with chronic kidney disease, with relative sparing of CYPs 1A2, 2C19, and 2D6 compared with 3A4 and 2C9.13
In vitro models are used to define the specificities of CYP enzymes for their substrates and inhibitors. However, activity in a test tube does not always correlate with that in a cell. These models use substrate and inhibitor concentrations that are much higher than would be encountered in vivo, and the mathematical models that extrapolate to clinically relevant processes yield conflicting results. Discrepancies in reported substrates, inhibitors, and inducers of specific CYP enzymes results.119
Most CYP enzymes involved in xenobiotic biotransformation have broad substrate specificity and can metabolize many xenobiotics.39 This is fortunate because the number of xenobiotic substrates likely already exceeds 200,000 and continues to grow.64 Broad substrate specificity often results in multiple CYP enzymes being able to biotransform the same xenobiotic. This enables the ongoing biotransformation despite an inhibition or deficiency of an enzyme. When a substrate is biotransformed by more than one enzyme, the enzyme with the highest affinity for the substrate usually predominates at low substrate concentrations, whereas enzymes with lower affinity become important at high concentrations. This transition is usually concomitant with, but not dependent on, the saturation of the catalytic capacity of the primary enzyme as it reaches its maximum rate of activity.39 The Km, which is defined as the concentration of substrate that results in 50% of maximal enzyme activity, describes this property of enzymes. For example, ADH in the liver has a very low Km for ethanol, making it the primary metabolic enzyme for ethanol when concentrations are low.65 Ethanol is also biotransformed by the CYP2E1 enzyme, which has a high Km for ethanol and only functions when ethanol concentrations are high. The CYP2E1 enzyme metabolizes little ethanol in moderate drinkers but accounts for significantly more biotransformation in alcoholics, as it is also inducible. As another example, diazepam is metabolized by both CYP2C19 and CYP3A4 enzymes. However, the affinity of CYP3A4 for diazepam is so low (ie, the Km is high) that most diazepam is metabolized by CYP2C19.44
The substrate selectivity of some CYP enzymes is determined by molecular and physicochemical properties of the substrates. The CYP1A subfamily has greater specificity for planar polyaromatic substrates such as benzo[a]pyrene. The CYP2E enzyme subfamily targets low-molecular-weight hydrophilic xenobiotics, whereas the CYP3A4 enzyme has increased affinity for lipophilic compounds. Substrates of CYP2C9 are usually weakly acidic, whereas those of CYP2D6 are more basic.64 High specificity also results from key structural considerations such as stereoselectivity. Some xenobiotics are racemic mixtures of 2 stereoisomers that are substrates for different CYP enzymes and have distinct affinities for the enzymes, resulting in different rates of metabolism. For example, R-warfarin is biotransformed by CYP3A4 and CYP1A2, whereas S-warfarin is metabolized by CYP2C9.97
The CYP enzymes that biotransform a specific xenobiotic cannot be predicted by its drug class. For example, the selective serotonin reuptake inhibitors (SSRIs) fluoxetine and paroxetine are both major substrates and potent inhibitors of CYP2D6, but sertraline is not extensively metabolized by this enzyme and exhibits minimal interaction with other antidepressants.5 Most β-hydroxy-β-methylglutarylcoenzyme A (HMG-CoA) reductase inhibitors are metabolized by CYP3A4 (lovastatin, simvastatin, and atorvastatin); however, fluvastatin is metabolized by CYP2D6, and pravastatin undergoes virtually no CYP enzyme metabolism at all.69 Among angiotensin-II receptor blockers, losartan and irbesartan are metabolized by CYP2C9, whereas valsartan, eprosartan, and candesartan are not substrates for any CYP enzyme. In addition, losartan is a prodrug whose active metabolite provides most of the pharmacologic activity, whereas irbesartan is the primary active compound. For these 2 drugs, the inhibition of CYP2C9 is predicted to have opposite effects.31
Adverse reactions to medications and drug–drug interactions are common causes of morbidity and mortality, the risk of which increases with the number of drugs taken (Chaps. 134 and 135). As many as 50% of adverse reactions are related to pharmacogenetic factors.34 The most significant interactions are mediated by CYP enzymes.78 The impacts of genetic polymorphism and enzyme induction or inhibition are addressed below.
The CYP enzymes are involved in many types of drug interactions. The ability of potential new drugs to induce or inhibit enzymes is an important consideration of industry. Drug development focuses on the potential of new xenobiotics to induce or inhibit other drugs or enzymes during the drug discovery phase. Various in vitro models enable this early determination.66
Many xenobiotics interact with the CYP enzymes. St. John’s wort, an herb marketed as a natural antidepressant, induces multiple CYP enzymes, including 1A2, 2C9, and 3A4. The induction of CYP3A4 by St. John’s wort is associated with a 57% decrease in effective serum concentrations of indinavir when given concomitantly.88 Xenobiotics contained in grapefruit juice, such as naringin and furanocoumarins, are both substrates and inhibitors of CYP3A4. They inhibit the first-pass metabolism of CYP3A4 substrates by inhibiting CYP3A4 activity in both the GI tract and the liver.21 Polycyclic hydrocarbons found in charbroiled meats and in cigarette smoke induce CYP1A2. Thus, for smokers who drink coffee, concentrations of caffeine, a CYP1A2 substrate, will increase following a permanent cessation of smoking.30
The response to xenobiotics and to coadministration of inhibitory or inducing xenobiotics is highly variable. The translation of DNA sequences into proteins results in the phenotypic expression of the genes. When a genetic mutation occurs, the changed DNA may continue to exist, be eliminated, or propagate into a polymorphism. A polymorphism is a genetic change that exists in at least 1% of the human population.34,74 A polymorphism in a biotransformation enzyme may change its rate of activity. The heterogeneity of CYP enzymes contributes to the differences in metabolic activity among patients.34 Differences in biotransformation capacity that lead to toxicity, once thought to be “idiosyncratic” drug reactions, are likely caused by these inherited differences in the genetic complement of individuals.
An individual with 2 CYP alleles of “normal” catalytic speed is called extensive metabolizer, which usually represents a majority of the population. There are 3 major metabolizer phenotypes due to polymorphism: poor, intermediate, and ultrarapid. Poor and intermediate metabolizers have either loss-of-function variants or absent CYP alleles. Poor metabolizers have 2 inactive or absent alleles, whereas intermediate metabolizers will have either 2 reduced function alleles or one “normal” allele with one inactive or absent allele. On the other hand, an ultrarapid metabolizer will have either gain-of-function variants or gene duplication.34,125 The CYP2C19 and CYP2D6 genes are highly polymorphic (Table 11–1). The CYP2D6 gene, which has 157 alleles, is associated with both ultrarapid and poor metabolism.49 The CYP2C19 and CYP2C9 genes are both associated with polymorphisms, resulting in poor metabolizers.13,78
The clinical implications of polymorphisms are vast. Prodrugs have limited activation in patients who are poor metabolizers. For example, clopidogrel mediates antiplatelet effects via an active thiol metabolite generated by CYP enzymes. Individuals with the loss-of-function CYP2C19*2 allele have decreased generation of the active metabolite, decreased antiplatelet effects, and increased cardiovascular events while on clopidogrel.55,99 Conversely, some ultrarapid metabolizers generate toxic concentrations of an active metabolic. Codeine (inactive) metabolizes to morphine (active) via CYP2D6. Ultrarapid metabolizers with CYP2D6 can have 2 to 13 copies of the gene leading to rapid conversion of codeine to morphine and as a consequence toxicity.67 Finally, some active drugs with inactive metabolites fail to reach therapeutic concentrations in patients who are ultrarapid metabolizers.34
Polymorphisms exist for enzymes other than CYP enzymes. A classic example is the inheritance of rapid or slow “acetylator” phenotypes. Acetylation is important for the biotransformation of amines (R–NH2) or hydrazines (NH2–NH2). Slow acetylators are at increased risk of toxicity associated with the slower biotransformation of certain nitrogen-containing xenobiotics such as isoniazid, procainamide, hydralazine, and sulfonamides.101 Genetic polymorphism of UDP-glucuronosyl transferase 2B17 (UGT2B17) contributes to a bimodal distribution of urinary conjugated testosterone excretion. Since the testosterone isomer, epitestosterone, has little genetic variation and is not affected by testosterone metabolism, an elevated testosterone:epistestosterone (T:E) is indicative of the exogenous administration of testosterone.115 The World Anti-Doping Agency sets the suspicious threshold ratio of T:E ratio at 4:1 in response to genetic variations.95,121 Athletes with homozygote deletions of UGT2B17 have less conjugation of testosterone and have had false negative testing using the previous ratio of 6:1 (Chap. 41).51
Polymorphic genes that code for enzymes in important metabolic pathways affect the toxicity of a xenobiotic by altering the response to, or the disposition of, the xenobiotic. An example occurs in patients with glucose-6-phosphate dehydrogenase (G6PD) deficiency. Glucose-6-phosphate dehydrogenase is a critical enzyme in the hexose monophosphate shunt, a metabolic pathway located in the red blood cell (RBC) that produces NADPH, which is required to maintain RBC glutathione in a reduced state. In turn, reduced glutathione prevents hemolysis during oxidative stress.15 In patients deficient in G6PD, oxidative stress produced by electrophilic xenobiotics results in hemolysis.
Biotransformation by induced CYP enzymes results in either increased activity of prodrugs or enhanced elimination of drugs. Stopping an inducer results in the opposite effects. Either way, maintaining therapeutic concentrations of affected drugs is difficult, resulting in either toxic or subtherapeutic concentrations. Interestingly, not all CYP enzymes are inducible. The inducible enzymes include CYP1A, CYP2A, CYP2B, CYP2C, CYP2E, and CYP3A.66
Although varied mechanisms of induction exist, the most common and significant is nuclear receptor (NR)-mediated increase in gene transcription.66 Nuclear receptors are the largest group of transcription factors (proteins) that switch genes on or off.113 They regulate reproduction, growth, and biotransformation enzymes, including CYP enzymes.109 Nuclear receptors exist mostly within the cytoplasm of cells. The CYP families 2 and 3 both have gene activation triggered through the NR pregnane X receptor (PXR) and the constitutive androstane receptor (CAR). The CYP1A subfamily uses the aryl hydrocarbon receptor (AhR) as its NR. Ligands, molecules that bind to and affect the reactivity of a central molecule, are typically small and lipophilic, enabling them to enter cells. Many xenobiotics are themselves ligands. Ligands bind the NRs, resulting in structural changes that enable the NR–ligand complexes to be translocated into the cell nucleus. Within the nucleus, NR–ligand complexes bind to proteins to create a heterodimer such as the retinoid X receptor (RXR) with either the PXR or the CAR. Similarly, the AhR interacts with the AhR nuclear translocator (Arnt) to create the nuclear heterodimer. This new heterodimer complex then interacts with specific response elements of DNA, initiating the transcription of a segment of DNA, and translation of its RNA resulting in the phenotypic expression of the respective CYP enzyme.
The ligand-binding domain of the PXR is very hydrophobic and flexible, enabling this pocket to bind many substrates of varied sizes and reflecting why the PXR is activated by a broad group of ligands.85,109 For example, xenobiotic ligands that bind the NR PXR that targets the CYP3A4 gene include rifampin, omeprazole, carbamazepine, and troleandomycin. Phenobarbital, a classic inducing xenobiotic, is a ligand that binds the CAR.113 The induction of CYP1A subfamily enzymes is through the interaction with the NR AhR. Exogenous AhR ligands are hydrophobic, cyclic, planar molecules. Classic AhR ligands include polyaromatic hydrocarbons (PAHs) such as 2,3,7,8-tetrachlorodibenzo-p-dioxin and benzo[a]pyrene.85
Induction requires time to occur because it involves de novo synthesis of new proteins. Similarly, withdrawal of the inducer results in a slow return to the original enzyme concentration.66 Polyaromatic hydrocarbons (PAHs) result in CYP1A subfamily induction within 3 to 6 hours with a maximum effect within 24 hours.104 The inducer rifampin does not affect verapamil trough concentrations maximally until one week; followed by a 2-week return to baseline steady state after withdrawal of rifampin.66 Xenobiotics with long half-lives require longer periods of time to reach steady-state concentrations that maximize induction. Phenobarbital and fluoxetine, which have long half-lives, fully manifest induction only after weeks of exposure. Conversely, xenobiotics with short half-lives, such as rifampin or venlafaxine reach maximum induction within days.13
Inconsistency in CYP induction exists in all organs and among individuals.66,85 In an in vitro study of the effects of inducing xenobiotics on 60 livers, differences in enzyme induction ranged from 5-fold for CYP3A4 and CYP2C9 up to more than 50-fold for CYP2A6 and CYP2D6.66 The inconsistency likely results from both polymorphisms and multiple environmental factors including diet, tobacco, and pollutants.66 There is variation in the extent to which inducers generate new CYP enzymes. Identical dosing regimens with rifampin resulted in induction of in vivo hepatic CYP3A4 with up to 18-fold differences between subjects.66 There is an inverse correlation of the degree of inducibility of an enzyme and the baseline enzyme concentration. Patients with a relatively low baseline concentration of a CYP enzyme will be more inducible than those with a high baseline concentration. Interestingly, the maximum concentrations of CYP enzymes seem to be quantitatively similar among individuals, suggesting a limit to which enzymes can be induced.66
Although the focus of this section is on CYP enzymes, it appears that most phases of xenobiotic metabolism are regulated by NRs.11 Also, just as genetic polymorphisms exist for CYP enzymes, they exist for NRs including the AhR, the CAR, and the PXR. This results in varied sensitivities to the ligands that complex with the NRs, ultimately resulting in differences in CYP enzyme induction.66,113
Inhibition of CYP enzymes result in either increased bioavailability of a drug or decreased bioactivation of a prodrug, and is the most common cause of harmful drug–drug interactions.86 Severe inhibition of CYP enzymes by a coadministered xenobiotic resulted in the removal of many medications from the market, including terfenadine, mibefradil, bromfenac, astemizole, cisapride, cerivastatin, and nefazodone.119 The appendix at the end of this chapter includes a comprehensive listing of CYP enzyme substrates, inhibitors, and inducers.
Inhibition mechanisms include irreversible (mechanism-based inhibition) and, the more common, reversible processes. The most common type of reversible inhibition is competitive, where the substrate and inhibitor both bind the active site of the enzyme.86,119 Binding is weak and is formed and broken down easily, resulting in subsequent enzyme liberation. It occurs rapidly, usually beginning within hours.13 Because the degree of inhibition varies with the concentration of the inhibitor, the time to reach the maximal effect correlates with the half-life of the xenobiotic in question.13 A competitive inhibitor can be overcome by increasing the substrate concentration. Each substrate of a CYP enzyme is an inhibitor of the metabolism of all the other substrates of the same enzyme, thereby increasing their concentrations and half-lives. Reversible, noncompetitive inhibition occurs when an inhibitor binds a location on an enzyme that is not the active site, resulting in a structural change that inhibits the active enzyme site. For example, noncompetitive inhibitors of CYP2C9 include nifedipine, tranylcypromine, and medroxyprogesterone.100 Another reversible mechanism results from competition between one xenobiotic and a metabolite of a second xenobiotic at its CYP enzyme substrate binding site. For example, the metabolites of clarithromycin and erythromycin produced by CYP3A inhibit further CYP3A activity. The effect is reversible and usually increases with repeated dosing.100 Some reversible inhibitors bind so tightly to the enzyme that they essentially function as irreversible inhibitors.86
Irreversible inhibitors have reactive groups that covalently, and thus permanently, bind the enzyme. They display time-dependent inhibition because the amount of active enzyme at a given concentration of irreversible inhibitor will be different depending on how long the inhibitor is preincubated with the enzyme. Because the enzyme will never be reactivated, inhibition lasts until new enzyme is synthesized.86 One measure of inhibitor potency is the inhibitory concentration, Ki, the concentration of the inhibitor that produces 50% inhibition of the enzyme. The more potent the inhibitor, the lower the value.104 Values below 1.0 μmol/L are regarded as potent.82 The azole antifungals are very potent, with Ki values of 0.02 μmol/L.104
The impact of an inhibitor is also affected by the fraction of the substrate that is biotransformed by the inhibited, target enzyme. The inhibition of a CYP enzyme will have little impact if the enzyme only metabolizes a fraction of the affected xenobiotic.82 Conversely, xenobiotics that are primarily metabolized by a single CYP enzyme are more susceptible to interactions.86 Simvastatin is biotransformed primarily by CYP3A4. The potent and specific CYP3A inhibitor itraconazole prevents its metabolism, resulting in an increased risk of rhabdomyolysis.1
Whereas 1A1 is located primarily in extrahepatic tissue, 1A2 is a hepatic enzyme and is involved in the metabolism of 10% to 15% of xenobiotics.13,66 They both are very inducible by polycyclic aromatic hydrocarbons, including those in cigarette smoke and charred food. They bioactivate several procarcinogens, including benzo[a]pyrene.57 Xenobiotics activated by the CYP1 enzyme family in the GI tract are linked to colon cancer.78 A xenobiotic interaction through CYP1A2 involves the addition of ciprofloxacin, a moderate inhibitor of CYP1A2, to a patient using tizanidine for muscle spasms. Oral administration of tizanidine, an α2 adrenergic agonist, yields low serum concentration because of extensive first-pass metabolism through CYP1A2. With concurrent ciprofloxacin dosing, serum tizanidine concentration increases significantly, and the interaction causes a clinically significant hypotension.37
This enzyme metabolizes approximately 7% of xenobiotics with many overlapping substrates with other CYP enzymes, especially CYP3A4.43,125 CYP2B6 comprises between 4% and 14% of total hepatic CYP enzymes and is polymorphic with 38 allele variations.49,125 Cyclophosphamide and ifosfamide are commonly used nitrogen mustard class of chemotherapeutics that require bioactivation. Metabolism through CYP2B6 yields the therapeutic phosphamide mustard metabolite, whereas metabolism through CYP3A4 inactivates the compound. Preferential metabolism through the induction of CYP2B6 increases therapeutic efficacy of cyclophosphamide and ifosfamide.43
The CYP2 enzyme family, with its 60 CYP2C9 alleles and 35 CYP2C19 alleles, is one of the most polymorphic of the CYP enzymes families (Table 11–1).30,49,114 The CYP2C9 enzyme is the most abundant enzyme of the CYP2C enzyme subfamily, which, with CYP2C19, comprises approximately 10% to 20% of the CYP enzymes in the liver and is involved in 15% to 20% of all xenobiotic metabolism.30,114 CYP2C9 biotransforms S-warfarin, the more active isomer of warfarin. Warfarin is one of the most commonly reported causes of adverse drug events. There is an association between slow metabolism and an increased risk of bleeding in patients taking warfarin.16,97
This enzyme is of historical significance because the exploration of differences in pharmacokinetics among individuals taking the antihypertensive debrisoquine led to the discovery of CYP2D6 and marked the beginning of pharmacogenetics.48 Debrisoquine through its effects on blood pressure acts as a probe molecule for enzymatic variations of CYP2D6. In fact, CYP2D6 is highly polymorphic with more than 109 allelic variants.49 Twenty-five percent of pharmaceuticals, including 50% of the commonly used antipsychotics and antidepressants, are substrates for CYP2D6.42,48,78,83 Because CYP2D6 is the only drug-metabolizing CYP enzyme that is noninducible, the polymorphisms are the primary reason for the substantial interindividual variation in enzyme activity.50 CYP2D6 enzyme is strongly inhibited by common antidepressants such as bupropion, fluoxetine, and paroxetine. Between inhibitors and poor metabolizers, opioid substrates such as codeine, tramadol, and hydrocodone that are bioactivated through CYP2D6 have less clinical efficacy.42 Ultrarapid metabolizers have higher incidences of postoperative and chemotherapy-induced vomiting when given ondansetron, a commonly used antiemetic metabolized by CYP2D6.9
This enzyme comprises 7% of the total CYP enzyme content in the human liver.79 It metabolizes small organic compounds, including ethanol, carbon tetrachloride, and halogenated anesthetics.104 It also biotransforms low-molecular-weight xenobiotics, including benzene, acetone, and N-nitrosamines.104 Some of its substrates are procarcinogens, which are bioactivated by CYP2E1.78 The assessment for a relationship between CYP2E1 and cancer is intense because many of its substrates are environmental xenobiotics. The induction of CYP2E1 is associated with increased liver injury by reactive metabolites of carbon tetrachloride and vinyl chloride (Chap. 21).36 During the metabolism of substrates that include carbon tetrachloride, ethanol, acetaminophen (APAP), aniline, and N-nitrosodimethylamine, CYP2E1 actively produces free radicals and other reactive metabolites associated with adduct formation and lipid peroxidation (Chaps. 9 and 33).17 CYP2E1 is inhibited by acute elevations of ethanol, an effect illustrated by the acute administration of ethanol inhibiting the metabolism of APAP. The chronic ingestion of ethanol hastens its own metabolism through CYP2E1 induction.36
CYP3A4 is the most abundant CYP in the human liver, comprising 40% to 55% of the mass of hepatic CYP enzymes.13,30 The CYP3A4 enzyme is the most common one found in the intestinal mucosa and is responsible for much first-pass drug metabolism.13 It is involved in the biotransformation of 50% to 60% of all pharmaceuticals.79,127 It has broad substrate specificity because it accommodates large lipophilic substrates and can adopt multiple conformations. It can even simultaneously fit 2 relatively large compounds (ketoconazole, erythromycin) in its active site.96
Methadone, extensively metabolized by CYP3A4, is associated with many examples of adverse drug interactions.54 Torsade de pointes due to the methadone dose–related prolongation of the QTC interval is reported in patients who take methadone in addition to CYP3A4 inhibitors including ciprofloxacin,75 itraconazole,81 and the antiretrovirals atazanavir and ritonavir.29 Ketoconazole inhibits CYP3A4, causing a 15- to 72-fold increase in serum concentrations of terfenadine, which also causes torsade de pointes.78 Bioflavonoids in grapefruit juice decrease metabolism of some substrates by 5- to 12-fold.13,78 The CYP3A4 enzyme does not exhibit significant genetic polymorphism; however, there are large interindividual variations in enzyme concentrations that can affect metabolic rates.127
Phase II biotransformation reactions are synthetic, catalyzing the conjugation of products of phase I reactions or xenobiotics with endogenous molecules that are generally hydrophilic. Conjugation usually terminates the pharmacologic activity of xenobiotics and greatly increases their water solubility and excretability.70,108,124 Conjugation occurs most commonly with glucuronic acid, sulfates, and glutathione. Less common phase II reactions include conjugation with amino acids such as glycine, glutamic acid, and taurine as well as acetylation and methylation.
Glucuronidation is the most common phase II synthesis reaction.70 Glucuronyl-transferase has relatively low substrate affinity, but it has high capacity at higher substrate concentrations.108 The glucuronic acid, donated by uridine diphosphate glucuronic acid, is conjugated with the nitrogen, sulfhydryl, hydroxyl, or carboxyl groups of substrates. Smaller conjugates usually undergo renal elimination, whereas larger ones undergo biliary elimination.70 The process of glucuronidation is reversible via β-glucuronidases. Although the liver has an overall low concentration of β-glucuronidases, the gut flora have high β-glucuronidase activity. Cleavage of the glucuronide metabolite enhances the lipophilicity and thus reabsorption through intestinal walls. This process is termed enterohepatic circulation, which decreases elimination and prolong toxicity from xenobiotics such as carbamazepine and phenobarbital.3,124 Multiple-dose activated charcoal provides therapeutic benefit to break enterohepatic circulation (Antidotes in Depth: A1).
Sulfation complements glucuronidation because it is a high-affinity but low-capacity reaction that occurs primarily in the cytosol. For example, the affinity of sulfate for phenol is very high (the Km is low), so that when low doses of phenol are administered, the predominant excretion product is the sulfate ester. Because the capacity of this reaction is readily saturated, glucuronidation becomes the main method of detoxification when high doses of phenol are administered.70,124 Sulfate conjugates are highly ionized and very water soluble.
Glutathione S-transferases are important because they catalyze the conjugation of the tripeptide glutathione (glycine-glutamate-cysteine, or GSH) with a diverse group of reactive, electrophilic metabolites of phase I CYP enzymes. The reactive compounds initiate an attack on the sulfur group of cysteine, resulting in conjugation with GSH that detoxifies the reactive metabolite. Of the 3 phase II reactions addressed, hepatic concentrations of glutathione by far account for the greatest amount of cofactors used. Although intracellular glutathione is difficult to deplete, when it does occur, severe hepatotoxicity often follows.124 Some GSH conjugates are directly excreted. More commonly, the glycine and glutamate residues are cleaved and the remaining cysteine is acetylated to form a mercapturic acid conjugate that is readily excreted in the urine. A familiar example of this detoxification is the avid binding of N-acetyl-p-benzoquinoneimine (NAPQI), the toxic metabolite of APAP, by glutathione.5,12
As with the CYP enzymes, many phase II enzymes are inducible. For example, UDP-glucuronosyltransferase, which performs glucuronidation, is inducible via the PXR, CAR, and AhR nuclear receptors after binding with rifampin, phenobarbital, and PAHs, respectively. Its activity varies 6- to 15-fold in liver microsomes.113 Similarly, UDP-glucuronosyltransferases are inhibited by xenobiotics such as amitriptyline, indinavir, phenylbutazone, and quinidine.63,111 Inhibition of glucuronidation exacerbates hyperbilirubinemia in patients with underlying Gilbert syndrome (reduced UDP-glucuronosyltransferase activity on bilirubin).111 Although inhibition of a high-capacity phase II metabolism step has theoretical implications and potential drug–drug interactions, the clinical implications are not well-studied.
Although the focus on drug disposition has traditionally been on biotransformation, membrane transporter proteins greatly impact drug disposition.45 Transporter proteins mediate the cellular uptake and efflux of endogenous compounds and xenobiotics.128 Their baseline physiologic role is to transport sugars, lipids, amino acids, and hormones so as to regulate cellular solute and fluid balance. Biotransformation cannot occur unless xenobiotics are taken up into the cells via transport proteins. After xenobiotics have undergone phase I and II metabolism, the metabolites undergo transport protein mediated efflux from the cell, an action that has been called phase III metabolism.52
Xenobiotic absorption, compartmentalization, and elimination are facilitated or prevented by the transport proteins.58 In the apical (luminal) membrane of the intestinal enterocytes, P-glycoprotein, part of the ABC family of transmembrane proteins, is important because it can actively extrude xenobiotics back into the intestinal lumen and effectively decreases absorption.14 The degree of phenotypic expression of P-glycoprotein affects the bioavailability of many xenobiotics, including paclitaxel, digoxin, and protease inhibitors.45 Transporter-mediated uptake of xenobiotics also occurs from the portal venous blood into the hepatocytes or from renal filtrate back into proximal tubular cells. α-Amanitin uses the sodium-dependent bile acid transporter, Na+-taurocholate cotransporting polypeptide, to enter hepatocytes, where it inhibits RNA-polymerase II and mediates cellular toxicity.40 Alternatively, transport proteins decrease xenobiotic entry into specific cells or compartments. P-glycoprotein in endothelial cells of the blood–brain barrier prevent CNS entry of substrate xenobiotics. Finally, transport proteins mediate xenobiotic elimination via hepatocyte efflux transporters that move biotransformed xenobiotics into bile and via efflux from renal proximal tubular cells into urine.14,45,128 Organs important for drug disposition have multiple transporters that have overlapping substrate capabilities, a redundancy that enhances protection.45
As with biotransformation enzymes and nuclear receptors, membrane transporters are also inhibited or induced. Digoxin, a high-affinity substrate for P-glycoprotein, has increased bioavailability when administered with P-glycoprotein inhibitors such as clarithromycin or atorvastatin.45 Loperamide is a substrate for P-glycoprotein that limits its intestinal absorption or CNS entry. Coadministration with quinidine, a P-glycoprotein inhibitor, or large dose ingestions (70–200 mg daily) that overwhelm the P-glycoprotein capacity result in increased opioid CNS effects of loperamide.24,45 As with the biotransformation enzymes, polymorphisms exist for membrane transporters. However, the clinical significance of these is not clear.19
Ideally and commonly, potentially toxic metabolites produced by phase I reactions are detoxified during phase II reactions. However, detoxification does not always occur. This section reviews mechanisms of cellular injury related to xenobiotic biotransformation.
Sometimes a xenobiotic is mistaken for an endogenous substrate by synthetic enzymes that biotransform it into an injurious compound. The incorporation of the rodenticide fluoroacetate into the citric acid cycle is an example of this mechanism of toxic injury (Fig. 11–3).90 Another example is illustrated by analogs of purine or pyrimidine bases that are phosphorylated and inserted into growing DNA or RNA chains, resulting in mutations and disruption of cell division. This mechanism is used therapeutically with 5-fluorouracil (5-FU), an antitumor pyrimidine base analog. When phosphorylated to 5-fluoro-dUTP and incorporated into growing DNA chains, it causes structural instability of the cellular DNA and inhibits tumor growth.94
FIGURE 11–3.
Pyruvate is converted to acetylcoenzyme A (acetyl-CoA), which enters the citric acid cycle as shown. Reducing equivalents, in the form of NADH and FADH, donate electrons to a chain of cytochromes beginning with NADH dehydrogenase. These reactions “couple” the energy released during electron transport to the production of ATP. Ultimately, electrons combine with oxygen to form water. The sites of action of xenobiotics that inhibit oxidative metabolism are shown. The sites where thiamine functions as a coenzyme are also illustrated.87 Dψm = mitochondrial membrane potential; DH = dehydrogenase. MPP+ =1-methyl-4-phenylpyridinium; PNU = N-3-pyridylmethyl-N-nitrophenyl urea; VPA = valproic acid.

Many toxic products result from metabolic activation (Table 11–2).53 The CYP enzymes most associated with bioactivation are 1A1, 1B1, 2A6, and 2E1, whereas 2C9 and 2D6 yield little toxic activation.39
Enzyme | CYP Substrate | Toxicity |
---|---|---|
1A1 | Benzo[a]pyrene (PAH) | IARC Group 1 |
1A2 | Acetaminophen Aflatoxin B (Aspergillus mycotoxin) 2-Naphthylamine (azo dye production) NNK (nitrosamine in tobacco) N-Nitrosodiethylamine (gas and lubricant additive, copolymer softener) | Hepatotoxicity IARC Group 1 IARC Group 1 IARC Group 1 IARC Group 2A |
2B6 | Chrysene (PAH) Cyclophosphamide Dapsone | IARC Group 2B IARC Group 1 Methemoglobinemia |
2C8,9 | Cyclophosphamide Phenytoin Valproic acid | IARC Group 1 IARC Group 2B Hepatotoxicity |
2C19 | Dapsone | Methemoglobinemia |
2D6 | NNK Dapsone | IARC Group 1 Methemoglobinemia |
2F1 | Acetaminophen 3-Methylindole (in perfumes, cigarettes for flavor) Valproic acid | Hepatotoxicity Pneumotoxicity Hepatotoxicity |
2E1 | Acetaminophen Acrylonitrile Benzene Carbon tetrachloride Chloroform Ethylene dibromide (former gas additive and fumigant) Ethyl carbamate (former antineoplastic) Halothane Methylene chloride N-Nitrosodimethylamine (formerly in rocket fuel) Styrene Trichloroethylene Vinyl chloride | Hepatotoxicity IARC Group 2B IARC Group 1 IARC Group 2B IARC Group 2B IARC Group 2A IARC Group 2A Hepatotoxicity IARC Group 2B IARC Group 2A IARC Group 2A IARC Group 2A IARC Group 1 |
3A4 | Acetaminophen Aflatoxin B1 (Aspergillus mycotoxin) Chrysene (PAH) Cyclophosphamide Dapsone 1-Nitropyrene (PAH) Senecionine (pyrrolizidine alkaloid) Sterigmatocystin (Aspergillus mycotoxin) | Hepatotoxicity IARC Group 1 IARC Group 2B IARC Group 1 Methemoglobinemia IARC Group 2B Hepatotoxicity IARC Group 2B |
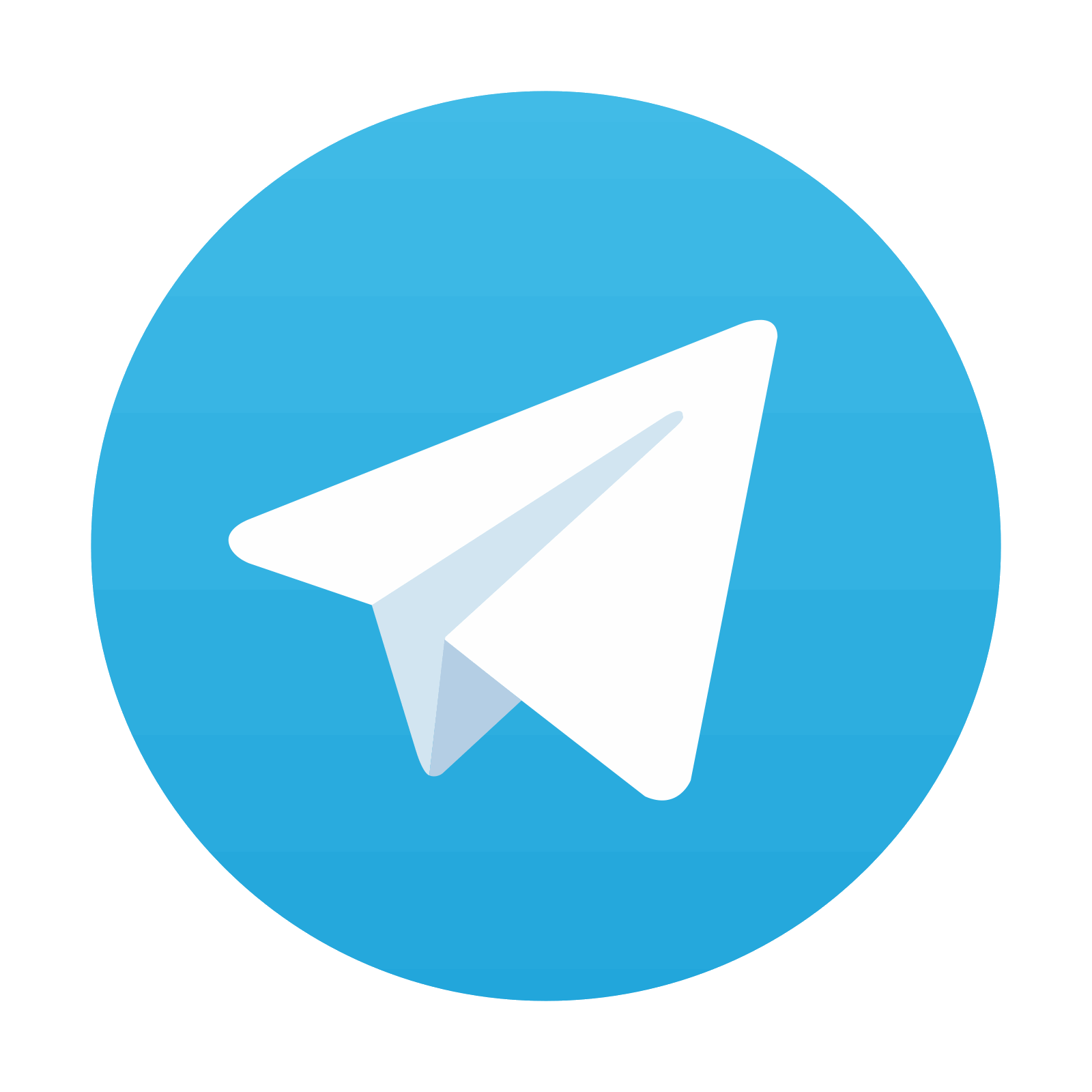
Stay updated, free articles. Join our Telegram channel
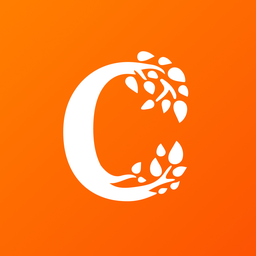
Full access? Get Clinical Tree
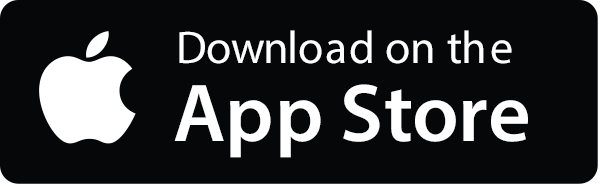
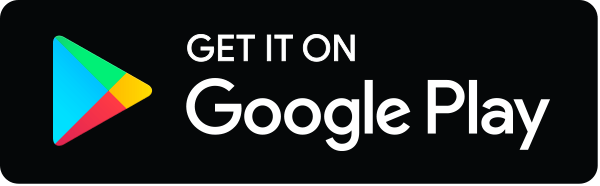
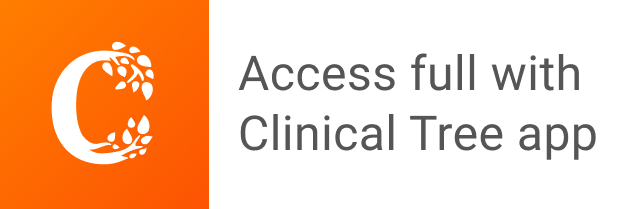