Central Nervous System
The central nervous system in the neonate differs from that in the older child: the myelination of nerve fibers is incomplete, and the cerebral cortex is less developed; its cellular elements continue to increase during the first years of life. Reflexes not seen in older children may be elicited. In the preterm, brain stem evoked potentials are prolonged, and the normalization of these coincides with maturation of respiratory control mechanisms.
Sensitivity to Pain
Until quite recently, little was understood of the ability of infants and small children to appreciate pain. As a result there was an unfortunate tendency to ignore the need for analgesia during painful procedures; even during and after surgical operations. It is now well established that neonates, including those born prematurely, may have increased sensitivity to pain and will react to it with tachycardia, hypertension, increased intracranial pressure, and a neuroendocrine response that exceeds that reported in adults. Infants demonstrate measurable behavioral responses to pain (e.g., crying, grimacing, restlessness); these responses have been used as a basis for pain scoring systems. Evidence suggests that infants who are subjected to painful procedures (e.g., circumcision) without adequate analgesia may experience an increased sensitivity to pain as older children. This has been attributed to the persistence of alterations in the infant’s central processing of painful stimuli. Control of intraoperative and postoperative pain, by modifying stress responses, may possibly even improve survival in infants with critical illness. It is for these reasons that we provide optimal analgesia or anesthesia for all infants and children during and after any painful procedure with the same care as we do for adults. As children grow from infancy into early childhood, their pain threshold remains reduced compared with that of older children or adults.
Suggested Reading
Cranium and Intracranial Pressure
The skull is less rigid in infants than in adults. As a result, an increase in the volume of its contents—blood, CSF, and brain tissue—can be accommodated to some extent by expansion of the fontanelles and separation of the suture lines. Palpation of the fontanelles can be used to assess intracranial pressure in infants.
CEREBRAL BLOOD FLOW AND INTRAVENTRICULAR HEMORRHAGE
Autoregulation of cerebral blood flow is impaired in sick neonates and blood flow is pressure dependent. Hypotension may lead to cerebral ischemia, and pressure fluctuations are transmitted to the capillary circulation. In the preterm infant, the cerebral vessels are very fragile, especially in the region of the germinal matrix overlying the caudate nucleus. Rupture of these vessels leads to intracerebral hemorrhage, which often extends into the ventricular system as an IVH.
Small preterm infants are very prone to IVH, which usually occurs during the first few days after birth and is a leading cause of morbidity and mortality. Potential predisposing factors to IVH include hypoxia, hypercarbia, hypernatremia, fluctuations in arterial or venous pressure, low hematocrit, overtransfusion, and rapid administration of hypertonic fluids (e.g., sodium bicarbonate).
The anesthesiologist should avoid precipitating these factors in the small preterm infant: airway manipulations, including awake endotracheal intubation and suctioning have been shown to increase blood pressure and anterior fontanelle pressure to similar extents. “Awake” intubation should be avoided whenever possible (even though a definite relationship between “awake” tracheal intubation and IVH has never been established). If the airway is known or appears to be difficult to secure or maintain by facemask, then an “awake” or preferably sedated tracheal intubation is a reasonable choice. If an awake tracheal intubation is planned, topical analgesia to the mouth and palate (using weight appropriate doses of local anesthetic [see page 145 ]) may attenuate the infant’s physiologic responses.
To further prevent blood pressure fluctuations during surgery or painful procedures, adequate anesthesia and analgesia should be provided. Rapid injections of undiluted hypertonic solutions, such as dextrose or sodium bicarbonate, should be avoided. Care should be taken to replace blood losses accurately. Severe anemia and/or coagulopathy should be corrected promptly. Periventricular white matter injury is a major cause of persistent brain injury in small preterm infants, and may follow IVH or may be a direct consequence of prematurity, hypoxia, ischemia, and inflammation. Indomethacin therapy may reduce the incidence of severe IVH.
Suggested Reading
Cerebrospinal Fluid and Hydrocephalus
The CSF, which occupies the cerebral ventricles and the subarachnoid spaces surrounding the brain and spinal cord, is formed by choroid plexuses in the temporal horns of the lateral ventricles, the posterior portion of the third ventricle, and the roof of the fourth ventricle. Meningeal and ependymal vessels and blood vessels of the brain and spinal cord also contribute small volumes of CSF.
The choroid plexuses are cauliflower-like structures consisting of blood vessels covered by thin epithelium through which CSF continuously exudes. The rate of secretion is about 750 ml/day in the adult (i.e., about five times the intracavity volume). Except for the active secretion of a few substances by the choroid plexus, CSF is similar to interstitial fluid.
CSF flow is initiated by pulsation in the choroid plexus. From the lateral ventricles, CSF passes into the third ventricle via the foramen of Monro and along the aqueduct of Sylvius into the fourth ventricle, each ventricle contributing more fluid by secretion from its choroid plexus. CSF then flows through the two lateral foramina of Luschka and the midline foramen of Magendie into the cisterna magna and throughout the subarachnoid spaces. CSF is reabsorbed into the blood by hydrostatic filtration through the arachnoid villi, which project from the subarachnoid space into the venous sinuses. Hydrocephalus is an abnormal accumulation of CSF within the cranium that may be either obstructive or nonobstructive.
Obstructive hydrocephalus is caused by a blockage in the flow of CSF. It may be communicating (e.g., when the CSF pathway into the subarachnoid space is open, as after chronic arachnoiditis) or noncommunicating (e.g., when the fluid’s pathway proximal to the subarachnoid space is obstructed, as in aqueduct stenosis or Arnold-Chiari malformation).
Nonobstructive hydrocephalus is caused by a reduction in the volume of brain substance, with secondary dilation of the ventricles; by overproduction of CSF (e.g., in choroid plexus papilloma); or by diminished reabsorption of CSF due to scarring.
EYES
Retinopathy of Prematurity
Retinopathy of prematurity (ROP), which is caused by retinal vessel proliferation and retinal detachment, is a leading cause of blindness. Improved survival of very-low-birth-weight infants increased the incidence of this condition. ROP is most common in neonates weighing less than 1500 g. Increased oxygen tension in the retinal arteries was thought to be the principal cause of ROP, although more recent research points to an effect on the processes of angiogenesis and vasculogenesis as the leading cause. These processes are stimulated by hypoxia. Exposure of the retina of the preterm to tissue oxygen levels in excess of the usual fetal levels interrupts normal vasculogenesis and may lead to subsequent hypoxic-ischemic tissue damage. At this stage additional oxygen may not cause further damage.
The cause of ROP is multifactorial; risk factors include hypoxia, hypercarbia, or hypocarbia; blood transfusion; exposure to light; recurrent apnea; sepsis; and other systemic illness. Occasionally ROP occurs in infants who have never been given supplemental oxygen and even in infants with cyanotic congenital heart disease. A major multi-institutional study of tight control of oxygen administration failed to reduce the incidence of ROP. Nevertheless, the inspired oxygen concentration should be carefully controlled in all preterm infants to avoid unnecessary hyperoxia. The safe level of Pa o 2 is now considered to be 50 to 70 mm Hg. Large fluctuations in inspired oxygen concentrations should be avoided. Monitoring oxygen saturation at a preductal site (right hand or earlobe), maintaining the SaO 2 at 90% to 95%, and avoiding major fluctuations in oxygenation are recommended. On occasion, it may still be necessary to err on the side of safety and administer high inspired oxygen concentrations. Major surgical procedures do not appear to predispose infants to ROP.
Suggested Reading
The Respiratory System
The respiratory system is of special interest to the anesthesiologist since this is the route of administration of inhaled anesthetic agents, and its functions may be significantly compromised during and after anesthesia. Changes in the respiratory system occur continuously from infancy to about age 12 years as the child grows to maturity.
Anatomy
There are major anatomic differences in the airway between the neonate, the small infant, and the adult that are important for the safe conduct of anesthesia:
- 1.
The head is relatively large and the neck is short, which may contribute to difficulty in laryngoscopy.
- 2.
The tongue is relatively large and readily blocks the pharynx during and after anesthesia: therefore, an oropharyngeal airway or a jaw thrust is often needed. The large tongue may also hamper attempts to visualize the glottis at laryngoscopy.
- 3.
The nasal passages are narrow and may be blocked by secretions or edema, which may cause serious problems. Neonates were previously described as “obligate nose-breathers,” but whether this is always true has been questioned. It is certain that some neonates may not immediately convert to mouth breathing if the nasal passages are obstructed. Upper airway obstruction is more likely to occur when the neck is flexed. The anesthesiologist should extend the infant’s neck after extubation until the infant completely recovers from anesthesia. Insertion of a nasogastric tube (NGT) increases total airway resistance; if the nares are of unequal size, the NGT should be inserted into the smaller nostril where it will have the least adverse effect.
- 4.
The larynx is situated more cephalad (C4) and anteriorly, and its long axis is directed inferiorly and anteriorly. The high cervical level of the larynx in the infant means that elevation of the head to the “sniffing position” during laryngoscopy will not assist in visualization of the glottis as it does in the adult. In the infant, if the head is elevated, the larynx also moves anteriorly (See page 94 ).
- 5.
The airway is narrowest at the level of the cricoid cartilage just below the vocal cords. This cartilage is the only solid circumferential component of the airway. The lining of the airway here is pseudostratified, ciliated epithelium that is loosely bound to the underlying tissue. Trauma to these tissues causes edema, and even a small amount of circumferential edema significantly compromises the already small cross-sectional area of the infant’s airway. The net effect is an increase in the resistance to airflow (which is turbulent [i.e., Reynold’s number >2500]) resulting in stridor, where: R α1/r 5 , (R is the resistance and r is the radius of the airway). Insertion of an ETT similarly encroaches on this space, as the internal diameter of the ETT becomes the area for gas flow; hence, airway resistance increases. This is more significant in smaller children (<3 yr). This is the reason that thinner uncuffed tubes used to be advocated in pediatric cases. Today, the wall thickness of cuffed and uncuffed tubes is the same.
- 6.
The epiglottis is relatively long and stiff. It is U-shaped and projects posteriorly at an angle of approximately 45 o above the glottis. Often, it must be lifted by the tip of a laryngoscope blade before the glottis can be seen. For this reason, a straight-blade laryngoscope is recommended for use in infants and children.
- 7.
The trachea is short (approximately 5 cm in the neonate), so precise placement and firm fixation of the ETT are essential. The tracheal cartilages are soft and can easily be compressed by the fingers of an anesthesiologist holding a mask or collapsed (dynamic compression) by the infant’s vigorous attempts to breathe against an obstructed airway.
- 8.
The right main bronchus is larger than the left and is less acutely angled at its origin. For this reason, if the ETT is advanced too far, it almost invariably enters the right main bronchus. This most often occurs while taping the tube in place or with changes in position for surgery after tracheal intubation. It is therefore essential to reassess the equality of bilateral breath sounds after repositioning for surgery (see Chapters 4 and 8 ).
- 9.
Because the ribs are almost horizontal, ventilation is mainly diaphragmatic. The abdominal viscera are bulky and can hinder diaphragmatic excursion, especially if the gastrointestinal tract is distended.
Table 2-1 lists the approximate airway dimensions in infants and children.
Age (yr) | Tracheal Length (cm) | Trachea (AP) | Diameter (mm) | |
---|---|---|---|---|
Right Bronchus | Left Bronchus | |||
>0.5 | 5.9 | 5.0 | 4.5 | 3.5 |
0.5–1 | 7.2 | 5.5 | 4.8 | 3.7 |
1–2 | 7.5 | 6.3 | 5.1 | 3.9 |
2–4 | 8.0 | 7.5 | 6.4 | 4.9 |
4–6 | 8.6 | 8.0 | 6.7 | 5.3 |
6–8 | 9.5 | 9.2 | 7.9 | 6.1 |
8–10 | 10 | 9.75 | 8.4 | 6.5 |
10–12 | 11.5 | 10.5 | 9.2 | 6.8 |
12–14 | 13.5 | 11.5 | 9.8 | 7.5 |
14–16 | 14.5 | 13.2 | 11.5 | 8.8 |
Suggested Reading
Physiology
Breathing movements begin in utero and are characteristically rapid, irregular, and episodic during late pregnancy. Normally, they are present 30% of the time in the third trimester, vary with the sleep state of the fetus and are subject to diurnal variation. Fetal breathing movements may play a role in lung development and provide exercise for the muscles of respiration. Monitoring of these movements may provide information on fetal health: hypoxemia leads to a decrease in fetal breathing, and severe hypoxemia leads to gasping movements. The fetal lungs are filled with fluid, which is moved by this respiratory muscle activity. After 26 to 28 weeks of gestation, production of surface-active substances (surfactants) is established in the type II pneumocytes. Surfactant is secreted into the lung and can be detected in amniotic fluid samples, providing a diagnostic index of lung maturity and hence neonatal prognosis.
Passage of the fetus through the birth canal compresses the thorax, forcing much of the fluid from the lungs through the nose and mouth. On delivery, this compression is relieved and some air is sucked into the lungs. Peripheral (cold, touch, temperature, etc.) and biochemical (respiratory and metabolic acidosis) stimuli have been thought to initiate the onset of regular, continuous breathing. Other factors may be important, such as an increase in the Pa o 2 or removal of central biochemical inhibitors. The first few spontaneous breaths are characterized by increased transpulmonary pressures (more than 50 cm H 2 O). They establish the FRC of the neonate’s lungs. Remaining lung fluid is removed over the first few days of life by the pulmonary lymphatics and blood vessels. Infants who are delivered by cesarean section are not subjected to the same thoracic squeeze and may have more residual fluid in the lungs. This may cause them to have transient respiratory distress (transient tachypnea of the neonate).
The stability of the alveolar matrix in the neonate depends on the presence of adequate amounts of surfactant, which may be deficient in the preterm infant. Lack of surfactant leads to collapse of alveoli, maldistribution of ventilation, impaired gas exchange, decreased lung compliance, and increased work of breathing (i.e., RDS). Not surprisingly, pneumothorax occurs more commonly during the neonatal period than at any other age.
Control of Respiration in the Neonate
Control of respiration, which involves biochemical and reflex mechanisms, is well developed in the healthy full-term neonate but exhibits several differences from adults. Respiration in the infant in relation to body mass is greater for any given arterial carbon dioxide tension (PaCO 2 ), reflecting a greater metabolic rate. The ventilatory response to hypercapnia is less in the neonate than in older infants, and less still in the preterm neonate. Any increase in the work of breathing is not well sustained. The slope of the carbon dioxide response curve is decreased in infants displaying episodes of apnea and hypoxemia decreases the response of the neonate to hypercapnia.
The neonate is sensitive to changes in arterial oxygen tension (PaO 2 ). Administration of 100% oxygen decreases ventilation, indicating the existence of tonic chemoreceptor activity. The ventilatory response of the neonate to hypoxia is modified by many factors, including gestational and postnatal age, body temperature, and sleep state. Preterm and full-term infants younger than 1 week of age who are awake and normothermic usually demonstrate a biphasic response to hypoxemia—a brief period of hyperpnea followed by ventilatory depression. Hypothermic infants and very small preterm infants respond to hypoxemia with ventilatory depression without the initial hyperpnea. This depression of ventilation has been attributed to the central effects of hypoxia on the cortex and medulla. The peripheral chemoreceptors, although active in neonates, are unable to maintain a significant influence on this hypoxic response. Infants show a less sustained response to hypoxia during REM sleep. In neonates, hypoxia also depresses the ventilatory response to carbon dioxide. Hypoxia may induce periodic breathing in infants, and this may be abolished by oxygen administration. Full-term infants older than 2 to 3 weeks of age demonstrate hyperpnea in response to hypoxia, probably as a result of maturing of the chemoreceptor function.
Reflexes that arise from the lung and chest wall are probably more important in maintaining ventilation in the neonate, possibly compensating for inadequacies in other control mechanisms. They primarily determine the rate (ƒ) and V t but are also important for maintaining lung volume (i.e., terminating expiration). The Hering-Breuer inflation reflex, which is active in the neonate, is even more powerful in the preterm infant. This reflex disappears during REM sleep and progressively fades during the early weeks of life. The paradoxical “Head” reflex, a large inspiration triggered by a small lung inflation, is active in the neonate. It may play a role in maintaining lung volumes in the neonate, and may be present during anesthesia.
Periodic breathing (rapid ventilation alternating with periods of apnea lasting 5 to 10 seconds) occurs in many preterm and some full-term infants. It is associated with increased peripheral chemoreceptor activity. In the preterm infant, the PaCO 2 is greater than normal during these episodes of periodic breathing, but the heart rate does not change significantly. In the full-term infant, hypocapnia may occur during periodic breathing, which seems to have no serious physiologic consequences and usually ceases by 44 to 46 weeks of postconceptual age. Periodic breathing generally only occurs about 3% of the time in full-term infants; a greater fraction of periodic breathing in a full-term infant is a warning sign of possible abnormal control of ventilation. Some preterm infants demonstrate far more serious and indeed life-threatening episodes of apnea. These commonly exceed 20 seconds and may be accompanied by bradycardia (possibly due to a chemoreceptor mediated reflex) and hemoglobin oxygen desaturation. Brief apnea spells (<20 seconds) may also be accompanied by significant bradycardia (<80 beats/min). The pathogenesis of apnea in preterm infants is not fully understood. Apnea may reflect an immature central respiratory control system because it tends to resolve as the brain matures. However, a variety of pathophysiologic mechanisms are involved. Apneic episodes may result from a failure of central control mechanisms (central apnea); in such instances, there is no ventilatory effort. It may also result from airway obstruction (obstructive apnea), in which case ventilatory effort may be observed but there is no gas exchange. Obstruction usually occurs in the infant’s nasopharynx, pharynx, or hypopharynx. Mixed apnea (a combination of central and obstructive) may also occur and one type may progress to another (i.e., obstructive apnea may progress to central apnea). Apnea may also result from failure of the ventilatory muscles. Many apneic episodes occur during REM sleep, when it is possible that fatigue of the ventilatory muscles is an important factor. Although neonatal apnea may be idiopathic, it may also be symptomatic of an underlying disease process, such as sepsis, intracranial bleeding, anemia, hypoglycemia, hypothermia, sensitivity to sedating medications, or patent ductus arteriosus.
Preterm infants must be carefully monitored to detect apneic episodes. Treatment is by tactile stimulation or, if this fails, by bag-mask resuscitation. The incidence of apneic episodes is decreased by therapy with aminophylline or caffeine (central stimulation) or by institution of continuous positive airway pressure (increased reflex activity of lung and chest wall reflexes and “splinting” of the airway). Preterm and former preterm infants up to 60 weeks postconceptual age, particularly those with anemia, are at risk for postoperative apnea even if apnea-free at the time of anesthesia. These infants will benefit from appropriate postoperative monitoring in an ICU or similar close observation unit with apnea monitoring.
Suggested Reading
Muscles of Respiration
The muscles of respiration in the neonate are subject to fatigue, a tendency that is determined by the types of muscle fiber present. In the diaphragm, 10% of the muscle fibers are type I (slow-twitch, highly oxidative, fatigue-resistant) in preterm infants, which increases to 25% in full-term infants, and reaches a maximum of 55% (the adult level) after 8 months postpartum. In the intercostals, 20%, 46%, and 65% of the fibers are type I for the same age groups, with the maximum reached by 2 months postpartum. Thus the preterm infant is prone to ventilatory muscle fatigue, a predisposition that progressively disappears with maturity. Ventilation is also affected by changes that occur during changing sleep states. The preterm infant spends 50% to 60% of this time in REM sleep, during which time, intercostal muscle activity is inhibited and paradoxical movement of the soft chest wall occurs. The reduced intercostal muscle activity is offset in part by an increase in diaphragmatic activity. Much of this activity is wasted when the ribs move paradoxically and may lead to diaphragmatic fatigue.
Suggested Reading
Respiratory Mechanics
The specific CL increases slowly after birth as fluid is removed from the lung. The chest wall compliance of the infant (especially the preterm infant) is great, so that total compliance approximates CL. This highly compliant chest wall provides a relatively weak force to maintain the FRC and to oppose the action of the diaphragm. The FRC of the small infant is maintained by a rapid respiratory rate, the point of termination of expiration, controlled expiration (“laryngeal braking”), and the tonic activity of the ventilatory muscles. This being so, it is not surprising that large decreases in FRC occur with apnea and during anesthesia when inhalation agents depress intercostal muscle function.
These large decreases in FRC are accompanied by airway closure and impaired oxygenation. Intercostal muscle inhibition during REM sleep or with inhaled anesthetic agents compounds the weakness of the chest wall and results in paradoxical movement. This paradoxical chest wall movement is markedly augmented by any airway obstruction. It may be inferred that infants generally require controlled ventilation during anesthesia and benefit from rapid respiratory rates or use of positive end-expiratory pressure to maintain the lung volume, avoid airway closure, and pharyngeal collapse during mask ventilation. As the child grows through infancy and childhood, the rib cage stiffens so that it becomes better able to oppose the action of the diaphragm and less reliant on intercostal muscle tone.
The transpulmonary pressures needed to optimally inflate the lungs are remarkably similar in healthy infants, children, and adults. During artificial ventilation, peak inspiratory pressures of 15 to 20 cm H 2 O are normal.
The nasal air passages contribute up to 50% of the total airway resistance in infants and slightly less in African American infants. Insertion of an NGT increases this resistance by as much as 50%. The nasal passages are usually of unequal size; if an NGT is inserted, it should be placed through the smaller nostril, so as to have a lesser effect on total nasal airway resistance. The resistance of the neonate’s peripheral airways is small but increases with age.
Lung Volumes
In the full-term infant, TLC is approximately 160 ml; the FRC is about half this volume. The V t is approximately 16 ml (6 to 7 ml/kg), and V D is about 5 ml (30% of the V t ). Relative to body size, all of these volumes are similar to adult values. Note, however, that any dead space in anesthesia or ventilator circuits is much more significant in relation to the small volumes of the infant (e.g., a 5 ml apparatus dead space would increase the total effective V D by 100%).
In contrast to the static lung volumes, V A is proportionally much greater in the neonate (~100 to 150 ml/kg/min) than in the adult (~60 ml/kg/min). This high V A in the infant results in a V A :FRC ratio of 5:1, compared with 1.5:1 in the adult. Consequently, the FRC is a much less effective “buffer” in the infant, so that changes in the concentration of inspired gases (including anesthetic gases) are more rapidly reflected in alveolar and arterial values.
The CV is relatively greater in infants and young children than in young adults; it may exceed the FRC to encroach on the V t during normal respirations. Airway closure during normal respirations may explain the reduced normal values for PaO 2 in infants and neonates ( Table 2-2 ). A decrease in FRC, which usually occurs during general anesthesia and persists into the postoperative period, further increases the significance of the large CV and increases the A-aDO 2 . The younger the infant or child, the greater the decrease in FRC. The intraoperative decrease in FRC may be partially reversed by continuous positive airway pressure.
Age | Normal Arterial Oxygen (mmHg) in Room Air |
---|---|
0–1 wk | 70 |
1–10 mo | 85 |
4–8 yr | 90 |
12–16 yr | 96 |
The total surface area of the air-tissue interface of the alveoli is small in the infant (2.8 m 2 ). When this area is related to the high metabolic rate for oxygen, it is apparent that the ratio between surface area and rate of oxygen consumption is smaller in the infant than the adult. As a result, the infant has a reduced reserve capability for gas exchange. This developmental fact assumes greater significance in the presence of congenital pulmonary hypoplasia or lung damage (e.g., from meconium aspiration). In such cases, the remaining healthy lung tissue may be inadequate to sustain life.
Work of Breathing
The muscles of respiration generate the force necessary to overcome the resistance to airflow and the elastic recoil of the lungs and chest wall. These two factors dictate an optimal rate of ventilation and a V t that delivers a given V A while expending minimal muscular energy for each child. Because the time constant of the infant’s lung is relatively small, efficient alveolar ventilation can be achieved at high respiratory rates. In the neonate, a respiratory rate of 37 breaths/min has been calculated to be most efficient, a rate that is close to the rate in healthy neonates. Full-term infants are similar to adults in that they require 1% of their metabolic energy to maintain ventilation; the oxygen cost of breathing is 0.5 ml / 0.5 L of ventilation. The preterm infant has a greater oxygen cost of breathing (0.9 ml/0.5 L), which is greatly increased if the lungs are diseased, as in RDS or bronchopulmonary dysplasia.
Ventilation-Perfusion Relationships in the Neonatal Lung
Ventilation (V) and perfusion (Q) are imperfectly matched in the neonatal lung. This may be in part a result of gas trapping in the lungs. <SPAN role=presentation tabIndex=0 id=MathJax-Element-1-Frame class=MathJax style="POSITION: relative" data-mathml='V./Q.’>V./Q.V./Q.
V . / Q .
mismatch is evident in the alveolar-arterial nitrogen difference (A-aDN 2 ), which is 25 mm Hg immediately after birth and declines to about 10 mm Hg within the first week. The normal PaO 2 in an infant breathing room air is about 50 mm Hg just after birth and increases to 70 mm Hg by 24 hours of age. The large A-aDO 2 in infants is mainly caused by persisting anatomic shunts (see page 26 ) and the relatively large CV.
Lung Surfactant
Surfactants in the alveolar lining layer stabilize the alveoli, preventing their collapse on expiration. Reducing the surface tension at the air-liquid interface in the alveoli also reduces the force required for their re-expansion. The principal surfactant in the lung is lecithin, which is produced by type II pneumocytes. The quantity of lecithin in the fetal lung increases progressively, beginning at 22 weeks of gestation and increasing sharply at 35 to 36 weeks as the lung matures. The lecithin production of the lung can be assessed by determining the lecithin/sphingomyelin (L/S) ratio in amniotic fluid and this is used as a measure of lung maturity and predictor of RDS. The L/S ratio is usually less than 1 until 32 weeks gestation, reaching 2 by 35 weeks, and 4 to 6 by full term.
Preterm infants with inadequate pulmonary lecithin production suffer from RDS. The biochemical pathways for surfactant production may also be depressed by hypoxia, hyperoxia, acidosis, or hypothermia; therefore, early correction of these abnormalities in the sick neonate is vitally important. Inhaled anesthetic agents seem to have little effect on surfactant production. Maturation of biochemical processes in the lungs of the fetus in utero may be accelerated by the administration of corticosteroids to the mother. The use of exogenous surfactant therapy to treat RDS is now well established.
Suggested Reading
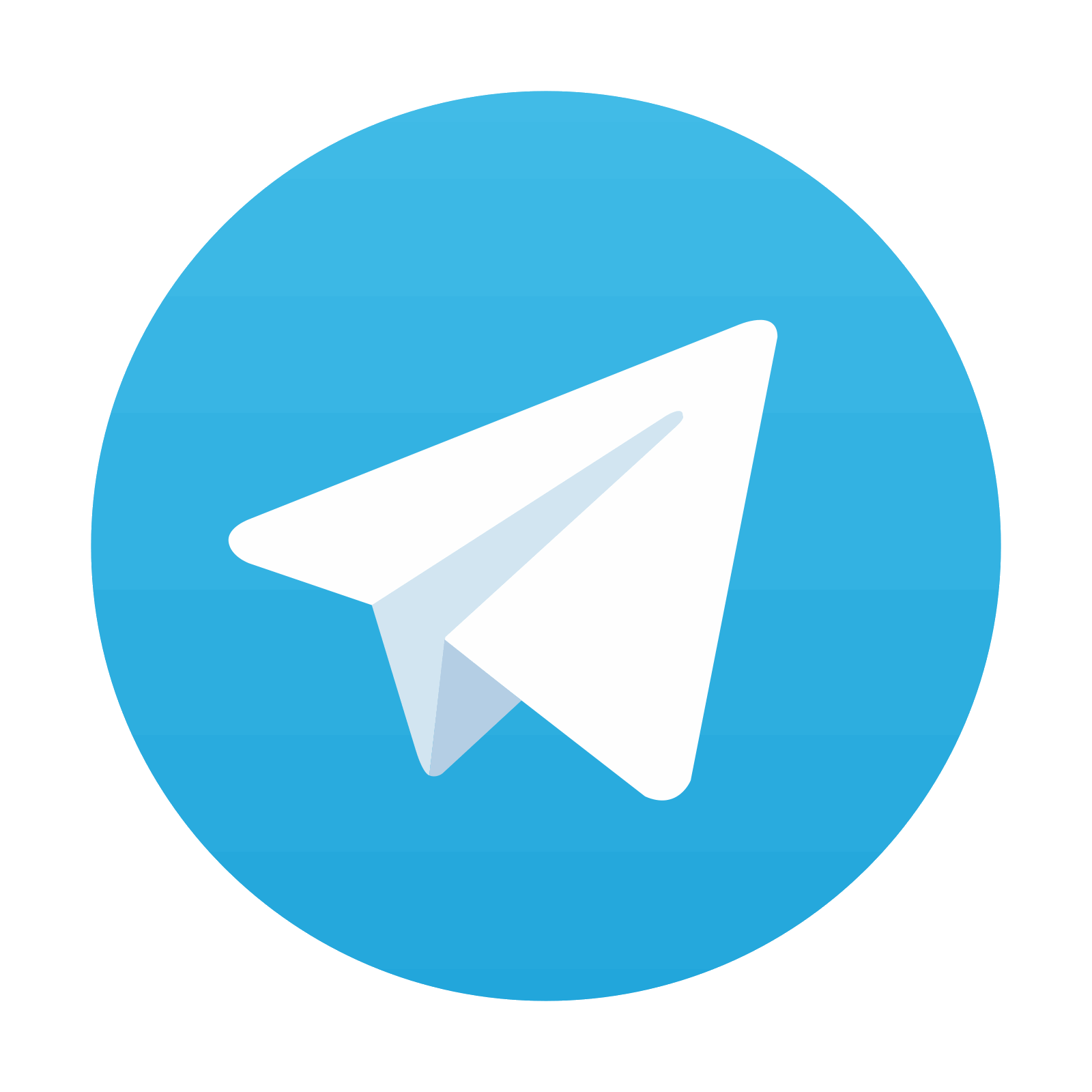
Stay updated, free articles. Join our Telegram channel
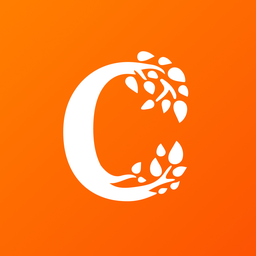
Full access? Get Clinical Tree
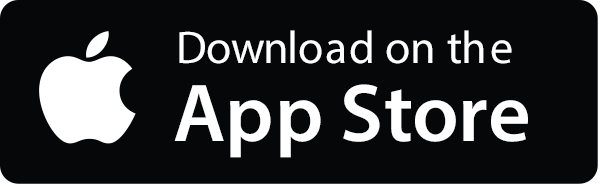
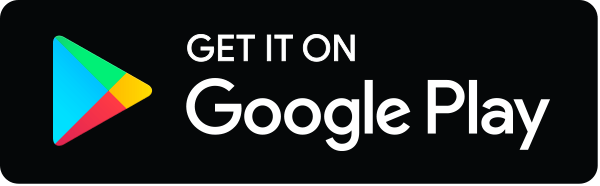