Abstract
In the 16th century, Paracelsus recognised that any medicinal substance has the potential to do harm, defining the dose as the determinant of whether the substance is a remedy or a poison. Despite this prescient and succinct observation, the intervening 450 years have failed to yield an agreed definition of an adverse drug reaction, although they all encompass the notion that intended therapeutic use of a medicine has resulted in unintended harm. We now know that not all adverse drug reactions are dose-related and the distinction between dose-related and non-dose-related reactions is commonly used in classification systems. Dose-related adverse drug reactions reflect inter-individual pharmacokinetic and pharmacodynamic variability. The risks of such reactions occurring with a particular drug depend on the difference between the effective therapeutic dose and the toxic dose, often described as the therapeutic index or certain safety factor. Although dose-related adverse drug reactions are more common during anaesthetic management than non-dose-related reactions, the latter can be the most dangerous, for example malignant hyperthermia or anaphylaxis. Across all branches hospital practice the greatest incidence of adverse drug reactions (total incidence and fatal outcomes) arises from drug administration errors. In anesthetic practice there have extensive analyses of the causes of drug errors and proposals to mitigate the human factors responsible: these include several technological innovations.
Keywords
adverse drug reactions, anesthetics, medication errors, malignant hyperthermia, butyrylcholinesterase, anaphylaxis
Chapter Outline
Types of Adverse Drug Reactions
Type A Reactions: Augmented (Dose-Related)
Type B Reactions: Bizarre (Drug Idiosyncrasy, Non–Dose-Related)
Type C Reactions: Chronic (Direct Organ Damage, Dose-Related, and Time-Related)
Type D Reactions: Delayed (Time-Related)
Definition
No medicine is risk free, yet the definition of an adverse drug reaction may not be straightforward. The World Health Organization (1972 Technical Report No. 498) defines an adverse drug reaction as “A response to a drug which is noxious and unintended, and which occurs at doses normally used in man for the prophylaxis, diagnosis, or therapy of disease, or for the modifications of physiological function.” One particular problem with this vague definition is that it assumes that all individuals are the same and hence the “normal dose” of a drug is to be comparable within a population. It does not consider differences in pathophysiology within individuals that can alter the way a drug behaves in terms of its pharmacokinetics or pharmacodynamics. Another problem is that it can be difficult to distinguish an adverse drug reaction from what is commonly known as a “side effect.” A side effect can occur within or outside the normal dose of a medication. One particular aspect of a side effect, which may differ from an adverse drug reaction, is that the effect can be beneficial. An example in anesthetic practice is the use of lidocaine as an antiarrhythmic. This drug was originally licensed for its use as a local anesthetic, yet its inhibitory effects on cardiac sodium channels can be used to dampen cardiac conduction. Further confusion with the definition of an adverse drug reaction is when a drug is being researched or is under surveillance after its initial commercial release. The European Medicines Agency good clinical practice guidelines state that “In the pre-approval clinical experience with a new medicinal product or its new usages, particularly as the therapeutic dose(s) may not be established: all noxious and unintended responses to a medicinal product related to any dose should be considered adverse drug reactions.”
The majority of adverse drug reactions can be attributed to a drug interacting with another or several other drugs. The practice of anesthesia involves the coadministration of several drugs alongside any drugs a patient may already be taking. The potential for drug interaction is therefore very high and this topic is covered elsewhere in this book (see Chapter 6 ).
With regard to anesthetic adverse drug reactions, a large analysis of more than 11,000 adverse drug reactions in more than 6600 patients in the United Kingdom showed a mortality of 9% with the largest proportion (>40% of deaths) associated with inhalation anesthetics and the smallest proportion (8% of deaths) from local anesthetics. The most frequently reported adverse drug reactions were associated with the use of intravenous induction agents.
Historically, adverse drug reactions were classified into two distinct categories; type A and type B. Type A reactions were classed as “dose-related” and included pharmocokinetic and pharmacodynamic variations within populations as well as drug interaction. Type B described reactions that were not dose related but idiosyncratic or allergic and often in genetically susceptible individuals. Type A reactions are more common than type B reactions and count for more than 80% of all reactions. This classification has evolved into one described neatly by Edwards and Aronson and a modification is shown in Table 7.1 . This chapter focuses on the general principles of the mechanisms of adverse drug reactions that can be applied to a wide range of drugs used in the practice of anesthesia. For ease, the layout of the chapter reflects the classification as described in Table 7.1 .
Mnemonic | Type of Reaction | Features |
---|---|---|
A: Augmented | Dose-related | Common Related to pharmacologic action of the drug Predictable Low mortality |
B: Bizzare | Drug idiosyncrasy, non–dose-related | Uncommon Not related to pharmacologic action of the drug Unpredictable High mortality |
C: Chronic | Dose-related, time-related, direct organ damage | Uncommon Usually dose-related |
D: Delayed | Time-related | Uncommon Usually dose-related Becomes apparent sometime after the administration of the drug |
E: End of use | Withdrawal | Uncommon Occurs soon after withdrawal of the drug |
F: Failure | Unexpected failure of therapy | Common Dose-related Often caused by drug interactions |
Drug Administration Errors
Adverse drug reactions can occur if the drug is incorrectly administered to a patient. Note that this does not fall into the classification described previously. Over the past 2 decades, NHS England (and formally the National Patient Safety Agency [NPSA]) and the Institute of Medicine in the United States have both compiled massive data highlighting that this is a serious and widespread issue in hospitals and drug administration errors are the single most preventable cause of patient harm. Although the majority of these reported errors lead to minimal or no harm, in anesthesia they have the potential to cause devastating effects. A U.K. study of 12,606 reported incidents showed medication errors occurred in 1,120 patients. Of these, only 15 (1.3%) resulted in severe harm or even death. A further 6-month analysis of reports to the NPSA regarding drug errors in intensive care showed of the 2428 incidents reported, 355 different drugs were involved, with morphine, gentamicin, and norepinephrine the most common. A review of anesthetic drug errors states that an error can happen as frequently as every 133 anesthetics. Much work is being done to prevent administration errors and recent evidence suggests that double-checking of drugs with a second person may reduce errors.
Drug errors need not be as late as the administration phase but can occur during the prescription. Illegible handwriting, inaccurate medication history, confusion with the drug name, inappropriate use of decimal points, use of abbreviations, and use of verbal orders have all been implicated in prescribing errors.
For some drugs, inaccurate knowledge of dosing information among prescribers can lead to erroneous dosing. A drug developed in a nonanesthetic context could have its dosing regimen inappropriately applied to the perioperative situation. This applies to some drugs that were introduced into clinical practice at a time when licensing legislation was less vigorous. A good example of this is morphine, for which the traditional quoted dose for severe postoperative pain is 0.15 mg/kg given intramuscularly. This dose was deemed the effective dose for battlefield casualties. However, one can now accept that battlefield casualties are an inappropriate model for postoperative pain as the pain tolerance of those injured in battle is high as a result of the psychological and neurohumoral response to the situation.
Another example is antiemetic medication. None of the currently available antiemetics was developed primarily for perioperative use. Many were first introduced as treatments for motion sickness, vestibular disorders, migraine, or for the treatment of side effects of radiation therapy or cytotoxic chemotherapy. The butyrephenone droperidol was introduced into anesthetic practice as a neuroleptic agent in a dose of approximately 0.1 mg/kg. Neuroleptanesthesia as a technique is now rarely performed; however, it does still demonstrate the antiemetic efficacy of droperidol. This efficacy is maintained at doses 10 times lower than those used for the neuroleptic effect. It may well be that more commonly used antiemetics such as phenothiazines and antihistamines (see Chapter 34 ) are also being used in inappropriately high doses in the perioperative setting with potentially avoidable side effects.
Types of Adverse Drug Reactions
Type A Reactions: Augmented (Dose-Related)
Paracelsus (1493-1541) once noted “All substances are poisons: there is none which is not a poison. The right dose differentiates a poison and a remedy.” Despite the administration of a drug in its intended therapeutic dose range, some patients exhibit an adverse effect. This may occur as a result of variation of an individual’s drug response within a unimodal population variation in response. This phenomenon is termed drug intolerance. The causes of drug intolerance are multifactorial with environmental and genetic factors involved. The intolerance of the drug is invariably related to the dose administered and there are numerous examples of these in anesthesia. One example is the intrathecal administration of a local anesthetic that can result in either a desired, low, or high block level dependent on patient characteristics. The most common dose-related reactions seen with anesthetic agents (inhalation or intravenous) are dampening of the normal cardiovascular and respiratory functions, and indeed these reactions are sometimes used for clinical benefit. This relationship between desired effect and unwanted effect remains at the forefront of toxicology.
Conventionally we measure the propensity for adverse effects of drugs by the therapeutic index. In the preclinical testing of new drugs, the therapeutic index provides a ratio of the LD 50 (the dose that causes death in 50% of animals) to the ED 50 (the dose that produces the desired effect in 50% of animals). The LD 50 and ED 50 are calculated from cumulative quantal dose-response curves as illustrated in Fig. 7.1 . Although widely established within toxicology, the therapeutic index does have its limitations. Consider two drugs A and B with the same ED 50 value. If the population variability on response to drug B is greater than drug A ( Fig. 7.2 ), then there will be a larger ED 95 (dose that produces the desired effect in 95% of subjects) value in drug B. Clinically the ED 95 or even the ED 99 is far more useful than the ED 50 as we would want as many patients as possible being treated with a beneficial effective dose, yet the dose must not reach levels that produce important unwanted effects. It also becomes clear that the ED 50 is not an ideal dose for clinical purposes as one would not want to produce an unwanted/lack of effect in 50% of patients. Figs. 7.3 and 7.4 further illustrate this principle. A more useful pharmacologic concept revolves around the certain safety factor . This is the ratio of the dose to produce an unwanted effect in a defined proportion of the population (usually 1%) to the dose to produce a desired effect in a proportion 100 minus that defined. In other words, the certain safety factor is most commonly described as TD 01 /ED 99 , where TD is the toxic dose. The certain safety factor can be adjusted if the unwanted effect of the drug is particularly serious; hence TD 0.1 /ED 99.9 or TD 0.01 /LD 99.99 , and so on could be used.




The doses used in the calculation of the certain safety factor also define the limits of the therapeutic window. Dose-response relationships remain a popular area for anesthetic research. Newer drugs, combinations of drugs, and techniques to administer them are slowly being introduced in all areas of anesthetic practice and each will undoubtedly have the ability to cause harm.
Type B Reactions: Bizarre (Drug Idiosyncrasy, Non–Dose-Related)
Some adverse drug reactions are not dose related and occur within individuals with genetic susceptibility. Pharmacogenetic variation has been identified in drug metabolism (acetylation, cytochrome P450 variants, plasma cholinesterase variants), inability to compensate for drug effects (glucose 6-phosphate dehydrogenase deficiency, acute porphyrias) in drug effects themselves (malignant hyperthermia), or even immune mediated responses (allergies). Each of these examples is discussed separately (also see Chapter 4 ).
Acetylator Status
Drugs such as isoniazid, hydralazine, procainamide, some sulfonamides, sulfasalazine, nitrazepam, and caffeine undergo acetylation, which is a phase 2 nonmicrosomal conjugation reaction. The enzyme group responsible are the N-acetyltransferases. There are two different isozymes in humans, encoded by the genes NAT1 and NAT2 . NAT1 is ubiquitously expressed and has 28 polymorphisms described to date, most of which have a similar acetylation rate as the reference gene. Currently there are 88 polymorphisms of NAT2 recognized. NAT2 is expressed in the liver, small intestine, and colon and is thus regarded as the enzyme responsible for processing xenobiotics. These polymorphisms are responsible for the observed enzyme processing behavior, which defines human individuals as fast, intermediate, or slow acetylators. The prevalence of slow acetylation can be as high as 60% in Caucasians and 10% to 20% in Asians. Slow acetylation, resulting in a prolonged half-life of the drug, can predispose individuals to a greater risk of side effects such as peripheral neuropathy (isoniazid), lupus syndrome (hydralazine and procainamaide), allergic reactions and hemolysis (sulfonamides), and gastrointestinal effects (sulfasalazine).
Cytochrome P450 Variants
The cytochrome P450 family of enzymes is responsible for microsomal phase I oxidation reactions. They exist in four classes (CYP 1-4), each with several subgroups. Most drugs can be metabolized by one or more of these subgroups. However, important polymorphisms have been found in four enzymes; therefore drugs metabolized predominantly by one of these enzymes could show a reduced clearance. Enzymes of particular interest to anesthesiologists include CYP 2D6, CYP 2C9, CYP 2C19, CYP 3A4-5, and CYP 2E1. The CYP 2D6 group is responsible for 25% of drug metabolism—most notably metoprolol, propranolol, amiodarone, flecainide, tricyclic and selective serotonin reuptake inhibitor antidepressants, phenothiazines, butyrophenones, and opioids. There is racial variation; 6% of Caucasians and 1% of Asians have reduced activity. The CYP 2C9 enzyme group is important for warfarin metabolism and individuals with reduced activity of CYP 2C9 are prone to hemorrhagic complications. CYP 2C19 is involved in the oxidation of diazepam and proton pump inhibitors with reduced activity more prevalent in Asians (20% vs. 3% in Caucasians). Important for the metabolism of midazolam, lidocaine, fentanyl, and alfentanil is CYP 3A4-5, which is responsible for 50% of drug oxidation reactions and has reduced activity in 6% of Caucasians. The metabolism of acetaminophen and the fluorinated volatile anesthetics are predominantly by the CYP 2E1 enzyme.
Plasma Cholinesterase Variants
Plasma cholinesterase (also known as pseudocholinesterase, serum cholinesterase, nonspecific cholinesterase, butyrylcholinesterase, S-type cholinesterase) is most notably responsible for the hydrolysis of the depolarizing muscle relaxant succinylcholine to succinyl monocholine and choline. It also accounts for the metabolism of the nondepolarizing muscle relaxant mivacurium and, to a lesser extent, ester local anesthetics, diamorphine, aspirin, and methylprednisolone. Genetic variation has accounted for reduced activity of this enzyme and is most clinically relevant to prolonged succinylcholine paralysis or scoline apnea. Investigating scoline apnea involves using inhibitors of plasma cholinesterase such as dibucaine (cinchocaine) and sodium fluoride and quantifying the reduction in activity that one should encounter in a normal enzyme. Four alleles—usual normal (Eu), atypical dibucaine-resistant (Ea), silent absent (Es), and fluoride-resistant (Ef)—have been identified on chromosome 3. Single–amino acid substitutions have resulted in each of these variants. Although most individuals are homozygous for Eu, up to 4% of the population have at least one deleterious allele, thus increasing the risk of a prolonged neuromuscular block, which can last between 10 minutes and several hours.
Glucose-6-Phosphate Dehydrogenase Deficiency
Erythrocytes are obligate glucose users and rely on the pentose phosphate pathway for its metabolism. Glucose-6-phosphate dehydrogenase (G6PD) is the rate-limiting enzyme in this pathway converting glucose-6-phosphate to 6-phosphoglucono-δ-lactone. The reaction usually provides energy for the cell in the form of reduced nicotinamide adenine dinucleotide phosphate, which is needed for the maintenance of reduced glutathione levels ( Fig. 7.5 ). Reduced glutathione has an important role in combating hydrogen peroxide produced within the cell during periods of oxidative stress. Individuals with G6PD deficiency therefore can have dangerously high levels of hydrogen peroxide (resulting in protein damage and cell death) under periods of oxidative stress. This can occur when oxidizing drugs such as primaquine, chloroquine, chloramphenicol, some sulfonamides, and vitamin K analogs are administered in susceptible individuals with the ultimate consequence being hemolytic anemia. The highest prevalence of G6PD deficiency appears in falciparum malaria zonal populations as this deficiency appears to confer a survival advantage against the parasite.

The Porphyrias
The porphyrias are a group of rare conditions caused by the excessive buildup of porphyrinogens, which are the precursors of heme. In affected individuals these porphyrinogens are excreted in urine and on exposure to light change color, resulting in the classic purple urine. The metabolic pathway for heme synthesis is shown in Fig. 7.6 . Under normal circumstances heme tightly controls its own production by feedback inhibition of the first of the seven enzymes in the pathway, δ-aminolevulinic acid (ALA) synthetase. This feedback inhibition can be disrupted in periods of bleeding or metabolic stress (infection, dehydration, fasting) resulting in induced ALA synthetase activity. A block in any of the enzymes in the pathway will result in accumulation of porphyrin precursors. An acute attack can result in porphyrinogen disrupting the nervous system leading to motor, sensory and autonomic dysfunction. Of particular importance to anesthesia is the potential for many drugs to induce the activity of ALA synthetase. Drugs such as barbiturates, etomidate, halothane, clonidine, metoclopramide, lidocaine, prilocaine, diclofenac, and ranitidine have all been implicated in precipitating an acute attack in susceptible individuals. There is some controversy surrounding the porphyrinogenicity of propofol. Animal experiments have shown it to induce ALA synthetase activity, and propofol infusions have resulted in raised porphyrin levels in humans with acute porphyria. Nevertheless, there are anecdotal reports of its safe use in patients with porphyria.

Malignant Hyperthermia
Malignant hyperthermia (MH) is a potentially lethal pharmacogenetic disorder attributable to faulty skeletal muscle intracellular calcium homeostasis. The disorder is genetically heterogeneous, but the final common pathophysiologic path is a loss of regulation of the calcium-release units of the myocytes. Fundamental to normal functioning of the calcium-release units is a tightly regulated bidirectional interaction between the voltage sensor of the T-tubular sarcolemmal membrane (dihydropyridine receptor [DHPR]) and the calcium release channel of the sarcoplasmic reticulum (ryanodine receptor isoform 1 [RyR1]). A majority of families with MH subjected to genetic investigations have potentially causative variants in RYR1 , the gene encoding RyR1. In other families, pathologic variants in RYR1 have been excluded. A small minority of these have variants in CACNA1S , the gene encoding the α 1 -subunit of DHPR. In the remaining 10% to 25% of families the gene or genes underlying MH remain to be identified. Potential candidate genes encoding proteins known to interact with RyR1 and DHPR number more than 30, but there is currently no evidence that any of these are implicated in MH. Further elucidation of the genetic etiology of MH will require genome-wide “next-generation” sequencing approaches.
Direct intracellular calcium measurements of muscle cells from humans and transgenic mouse models suggest, contrary to previous assumptions, that calcium cycling is abnormal even without exposure to triggering agents. Even though calcium sequestration is upregulated, cytoplasmic resting calcium ion concentration is elevated and evidence is emerging that this may predispose to sarcopenia or even frank myopathy in the long term. The most obvious manifestation of MH susceptibility is, however, a pharmacologically triggered massive and persistent increase in cytoplasmic calcium ion concentration (see Fig. 7.6 ). This results in metabolic stimulation and sustained contractile activity with disruption of sarcolemmal integrity. The clinical consequences are increased oxygen consumption, carbon dioxide production and tachycardia, hyperthermia, acidosis, rhabdomyolysis, and muscle rigidity. A critical stage appears to be related to mitochondrial failure brought about by mitochondrial calcium accumulation secondary to the cytoplasmic calcium accumulation. Death results from any one, or a combination, of hyperkalemia, disseminated intravascular coagulopathy (secondary to hyperthermia), profound acidosis, or hyperthermia per se.
The pharmacology of MH triggering has been reviewed. Essentially, the primary triggers of MH are the potent inhalation anesthetics, including all the currently available agents (isoflurane, sevoflurane, desflurane). They appear to act on RyR1 by overriding the physiologic magnesium inhibition of the channel. The clinical effect may be almost immediate and rapidly progressive or more insidious with signs in some reports delayed for more than 6 hours into anesthesia. Succinylcholine is likely to cause generalized or jaw muscle rigidity and also seems to enhance the onset and severity of a reaction triggered by subsequent exposure to a volatile anesthetic, but at worst is only a weak trigger of the hypermetabolic MH response when administered without a potent inhalation agent. There is no convincing evidence that any other drugs can trigger MH.
Successful treatment of MH depends on early recognition of the evolving reaction. A combination of unexplained tachycardia and an unexplained increase in carbon dioxide (CO 2 ) production makes the diagnosis likely. An increasing body temperature may be observed almost simultaneously or can be delayed for several minutes. Treatment involves discontinuation and enhancement of elimination of volatile anesthetics (including the use of adsorbents such as charcoal filters inserted in the anesthetic breathing circuit), hyperventilation with 100% oxygen (O 2 ), body cooling, and administration of dantrolene, a drug that reduces sarcoplasmic reticulum calcium release. General supportive measures are used to treat acidosis, hyperkalemia, myoglobinemia, arrhythmias, and disseminated intravascular coagulation.
Allergic Drug Reactions
Allergic reactions to drugs administered intravenously are acute type I hypersensitivity reactions. This requires an allergen to be exposed to an individual for a second or subsequent time. Epidemiologic data for anesthetic allergic reactions are divergent as some confusion remains as to the definitions of allergy and anaphylaxis. Indeed, some reactions that are accepted as side effects of certain drugs—for instance, histaminergic reactions owing to atracurium and mivacurium—may be underreported. The European Academy of Allergy and Clinical Immunology defines allergy as follows:
Allergy is a hypersensitivity reaction initiated by immunological mechanisms. Allergy can be antibody- or cell-mediated. In the majority of cases the antibody typically responsible for an allergic reaction belongs to the IgE isotype and these individuals may be referred to as suffering from an IgE-mediated allergy. Not all IgE associated ‘allergic’ reactions occur in ‘atopic’ subjects. In non-IgE-mediated allergy the antibody can belong to the IgG isotype, e.g. anaphylaxis due to immune complexes containing dextran, and the classical, nowadays rare, serum sickness previously referred to as a Type III reaction. Both IgE and IgG antibodies are found in allergic bronchial pulmonary aspergillosis (ABPA). Allergic contact dermatitis is representative of allergic diseases mediated by lymphocytes.
Anaphylaxis is essentially a severe, life-threatening, generalized or systemic hypersensitivity reaction. It has recently been reclassified as a spectrum of disease, and for the purposes of recent national audits in the United Kingdom, was categorized into five grades based on severity of reaction and need of intervention ( Table 7.2 ). The clinical features (hypotension, tachycardia or bradycardia; cutaneous flushing, rash or urticaria; bronchospasm; hypoxia; angioedema and cardiac arrest) usually occur within a few minutes but may be delayed for up to an hour. True allergic anaphylactic reactions involve mast cell degranulation resulting from production of allergen-specific immunoglobulin (Ig) E, IgG, or complement activation. Nonallergic anaphylactic reactions can occur through a direct drug action on the mast cell itself. True allergic anaphylaxis can occur after exposure to the smallest dose of antigen. Direct mast cell degranulation (non–IgE-mediated response) is probably dependent on the mass of drug and its rate of administration. However, in these circumstances there is unlikely to be any response to a test dose, but the full dose will cause the reaction. It is advisable, therefore, to administer intravenous drugs slowly.
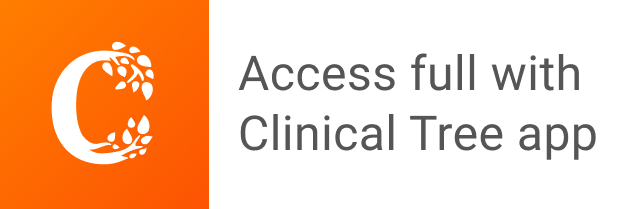