Abstract
Inhaled anesthetics as single agents provide all of the essential features of general anesthesia, including amnesia, unconsciousness, and immobility. Each of these components results from agent-specific actions on distinct neuronal pathways in the central nervous system. The characteristic immobilizing effect of volatile anesthetics involves a site in the spinal cord. Amnesia and unconsciousness involve incompletely understood supraspinal mechanisms. The precise mechanisms by which inhaled anesthetics alter synaptic transmission have not been fully elucidated despite a number of promising candidate molecular targets. The typical anesthetic binding site is hydrophobic and amphipathic. In contrast to intravenous anesthetics, inhaled anesthetics can act at a number of membrane bound ion channels and receptors at clinically relevant effect-site concentrations. Volatile anesthetics have potentiating effects on inhibitory GABA A and glycine receptors and K + channels, and inhibitory effects on excitatory Na + and Ca 2 + channels. The gaseous anesthetics nitrous oxide and xenon have predominant NMDA-type glutamate receptor blocking effects. Inhaled anesthetics have a diverse range of clinically significant effects on multiple organ systems, both beneficial and potentially adverse. Potentially beneficial effects include organ protection, while adverse effects include respiratory and cardiovascular depression. Inhaled anesthetics have agent-specific pharmacologic, pharmacokinetic, and side-effect profiles that determine their optimal clinical applications based on patient and procedure specific applications. Anesthetic-induced neurotoxicity occurs in animal models at critical periods in early brain development with unclear clinical significance.
Keywords
Meyer-Overton, anesthetic preconditioning, anesthetic neuroprotection, anesthetic neurotoxicity, binding site, ion channel, molecular mechanisms of anesthetic action, postoperative cognitive dysfunction
Chapter Outline
Structure-Activity Relationships
From Lipid-Based to Protein-Based Mechanisms
Diversity of Molecular Targets
Molecular and Cellular Sites of Action
Unique Features of Individual Agents
Common Clinical Indications and Considerations
Anesthesia and Neurodegenerative Disease
Neuroinflammation and Cognitive Dysfunction
Inhaled Anesthetic Sensitivity: Triple Low
Closed-Loop Anesthetic Delivery
This chapter focuses on the pharmacology of the inhaled anesthetics, including their mechanisms of action and clinical effects (pharmacodynamics). Related pharmacokinetic principles of inhaled anesthetics are covered in Chapter 3 .
Historical Perspective
Discovery of Inhaled Anesthetics
The German physician and botanist Valerius Cordus (1515–1544) described a method for preparing ether by combining sulfuric acid with alcohol, which he called oleum dulci vitrioli or “sweet oil of vitriol.” Paracelsus (1493–1541), the father of toxicology, recognized the analgesic properties of ether:
[I]n diseases which are treated by allaying the pain, it cures all of the disorders, relieves all of the pains, reduces the fever, and prevents the disagreeable complications of all sicknesses.
Paracelsus was unknowingly close to the discovery of the anesthetic effects of ether. Two centuries later in the 18th century, despite flammability issues and the lack of appropriate delivery systems, Friedrich Hoffmann of Halle (1660–1742) recommended the introduction of ether into medicine.
In 1772 nitrous oxide (N 2 O) was discovered by the English philosopher and chemist Joseph Priestley (1733–1804). He and other scientists such as Joseph Black (1728–1799) and Antoine Lavoisier (1743–1794) identified many other atmospheric gases, including oxygen, hydrogen, and carbon dioxide, pioneering the field of “pneumatic medicine” and elucidating the basic properties of gases. In 1799 Thomas Beddoes (1760–1808) established the Pneumatic Institution for Relieving Diseases by Medical Airs in England. Together with young assistant Humphry Davy (1778–1829), who ultimately became more successful than him, Beddoes experimented with N 2 O. Davy performed numerous experiments on himself and discovered both the mood-enhancing effects, leading to the name laughing gas, and the analgesic effect of N 2 O. Despite these pain-relieving effects and its potential use for surgical procedures, N 2 O did not meet expectations as a cure for diseases, and the Pneumatic Institution soon closed.
Decades later Gardner Quincy Colton (1814–1898), a self-proclaimed professor of chemistry, went on tour with his (lucrative) “scientific exhibits” and public demonstrations of N 2 O. In 1844 dentist Horace Wells (1815–1848) attended one of Colton’s demonstrations of the effects of laughing gas and observed its analgesic effects in a fallen volunteer. The next morning, a fellow dentist removed one of Wells’ molars with only little pain while Colton administered N 2 O. Wells saw potential in N 2 O and scheduled a demonstration at Harvard Medical School in 1845 with help of his assistant William T.G. Morton (1819–1868). During the demonstration the patient cried out and moaned, probably owing to failure of continuous delivery of the gas, an incident that discredited N 2 O as an anesthetic. Wells later committed suicide.
Morton was searching for another suitable substance to relieve pain during tooth extractions. He met Charles T. Jackson (1805–1880), a Harvard professor in chemistry who introduced him to ether. Morton’s experiments on himself and on his pet animals were promising, and in September 1846 he performed a painless tooth extraction. This caught the attention of Harvard surgeon Henry J. Bigelow (1818–1890), who helped schedule a successful public demonstration at Massachusetts General Hospital on October 16, 1846, which is considered by many the beginning of the era of anesthesia. Morton did not reveal the identity of the substance he called Letheon, which was ether he masked with additional fragrances and colors. Most likely he saw a potential for profit and unsuccessfully attempted to patent Letheon. Under pressure from the hospital, Morton revealed the identity of the main ingredient ether, and after further procedures with ether followed, Bigelow published the results of ether as a suitable agent for use in surgical procedures. The term anesthesia is derived from Greek αn – “without” and aisthētikos “perception” or “sensation.”
Controversy regarding who first discovered anesthesia arose with contenders including Wells, Jackson, and especially Crawford W. Long (1815–1878), a physician in rural Georgia who had used ether anesthesia since 1842 but did not publish his work until after Bigelow’s report. Morton with his perseverance is generally credited with the discovery of anesthesia, although there are regional sentiments favoring Wells or Long. The use of both ether and N 2 O was quickly adopted by many institutions, revolutionizing surgery and defining the field of anesthesia.
The science of anesthesia began with the introduction and development of chloroform as an anesthetic by John Snow (1813–1858) in England. He conducted experiments on the physical and pharmacologic properties of chloroform and other inhaled anesthetics, developed methods for their administration by introducing the chloroform inhaler, studied their side effects and complications, and developed specific clinical applications. His work established the scientific basis of anesthesia by determining the relationships between anesthetic solubility, vapor pressure, and potency. He also described five stages of etherization in response to increasing doses, presaging Guedel’s description, and the first dose-response relationship for an anesthetic. These are among the seminal foundation studies of inhalational anesthesia.
Development of Modern Inhaled Anesthetics
The development of the modern potent inhaled anesthetics was facilitated by developments in fluoride chemistry that accelerated with the atomic weapons program in the mid-20th century. This allowed the synthesis and testing of many fluorinated alkane and ether compounds that were safer and less combustible than ether or cyclopropane. Robbins demonstrated the potential of fluorinated hydrocarbons as nonflammable inhaled anesthetics with greater therapeutic ratios than ether or chloroform. This work led to the synthesis and testing of the polyhalogenated alkane halothane, which showed outstanding anesthetic properties in animals and humans. This was followed by the pioneering work of Ross Terrell (1925–2010) and colleagues who synthesized and evaluated more than 700 fluorinated compounds that included the essential agents of the past 40 years of modern inhalational anesthesia, the fluorinated methyl ethyl ethers: enflurane, isoflurane, sevoflurane, and desflurane ( Fig. 11.1 ).

Structure-Activity Relationships
Meyer-Overton Correlation
At the beginning of the 20th century, Meyer and Overton independently observed that the potency of volatile anesthetics for sedating tadpoles is closely correlated with their solubility in olive oil ( Fig. 11.2A ). Subsequent investigations confirmed that for a majority of anesthetic agents potency is proportional to lipid solubility as measured by the oil-gas partition coefficient. Remarkably, this correlation holds across multiple animal phyla from invertebrates to humans and spans over five orders of magnitude in potency. Another way of stating this correlation is that the product of the oil-gas partition coefficient and anesthetic potency defined as minimum alveolar concentration (MAC; see Chapter 3 ) is constant between anesthetics. This implies that at MAC the concentration of inhaled anesthetic molecules in the hydrophobic phase is approximately 50 mM. This observation formed the basis for a unitary hypothesis that argued that the mechanism of action of inhaled anesthetics was derived from their effects on bulk lipid membrane properties. A more modern, mechanistic form of the lipid-based hypothesis suggests that anesthetic-induced changes in the bilayer lateral pressure profile of lipids in the vicinity of membrane proteins alter their functional properties.

Despite its central position in mechanistic theories of anesthesia, the Meyer-Overton correlation had limitations. Although Meyer and Overton both used olive oil as their reference solvent, similar correlations have been obtained using other hydrophobic solvents that are more hydrophobic than olive oil, such as benzene, which do not produce as strong a correlation as olive oil. The amphipathic (having both hydrophobic and hydrophilic qualities) solvents methanol, octanol, and lecithin produce even better correlations than olive oil, correctly predicting that enflurane is less potent than isoflurane. However, lipid solubility alone does not predict anesthetic potency in that a number of chemically similar compounds with lipid solubilities comparable to known anesthetics lack anesthetizing capacity themselves. These so-called nonimmobilizers have been useful experimentally as a negative test in evaluating potential anesthetic targets. Although the Meyer-Overton correlation does constrain the possible mechanisms of action of anesthetics (the site at which anesthetics bind must be hydrophobic, and likely amphipathic), in practice this is not a particularly restrictive constraint on possible anesthetic binding sites because lipid bilayers, membrane proteins, and most water-soluble proteins have amphipathic domains. Functional studies indicate that volatile anesthetics do not alter bulk lipid bilayer properties at clinically relevant concentrations that do modify ion channel function.
Stereoselectivity
Isoflurane, enflurane, halothane, and desflurane all have a chiral carbon atom, and thus they exist as racemic mixtures of enantiomeric pairs that are mirror images of each other, but with identical physical and chemical properties other than their ability to polarize light in opposite directions (see Chapter 1 ). While they are synthesized as racemic mixtures, separation of the enantiomers of isoflurane and halothane by gas chromatography allowed testing of the anesthetic properties of the individual enantiomers, which have identical lipid solubilities. Rats are about 1.5-fold more sensitive to the (+)enantiomer than the (−)enantiomer of isoflurane, while its lipid perturbing effects are equivalent (nonstereoselective). A number of putative targets (see later text), including gamma-aminobutyric acid (GABA) A receptors and N-methyl- d -aspartate (NMDA) receptors, have demonstrated stereoselectivity in vitro, although not to the degree seen in vivo. Interestingly, L-type calcium ion (Ca 2+ ) channels (which partially mediate the cardiodepressive effects of volatile agents) do not demonstrate stereoselectivity in vitro, suggesting that the more potent enantiomer should have an increased therapeutic ratio (reduced cardiovascular depression) compared with the racemic mixture. Importantly, the stereoselectivity of anesthetic potency indicates that lipid solubility alone does not determine anesthetic potency.
Mechanisms of Action
From Lipid-Based to Protein-Based Mechanisms
The chemical diversity of the agents that can be used as general anesthetics historically favored a unitary mechanism to explain anesthesia. The Meyer-Overton correlation suggested that the effect sites of anesthetics were lipophilic or amphipathic. As a result, initial concepts of general anesthetic mechanisms favored effects on the bulk properties of lipid membranes, which allowed for the vast chemical diversity of agents found to produce an anesthetic state (see earlier text). However, no verifiable hypothesis of a lipid-based mechanism, which usually invoked effects on lipid membrane properties (e.g., bilayer fluidity, thickness, curvature, permeability), was developed. Moreover, comparable effects on membrane biophysical properties could be achieved by small changes in temperature that do not cause anesthesia. Subsequently, the nonimmobilizers were found to violate the basic Meyer-Overton correlation between potency and lipophilicity. The improved correlation between anesthetic potency and solubility in amphipathic rather than purely hydrophobic solvents is consistent with a protein-based mechanism, or perhaps a lipid bilayer with phospholipoproteins. The proof of concept for a protein as an anesthetic target was provided by Ueda and extended unambiguously by Franks and Lieb who reported that the bioluminescent function of the protein luciferase was inhibited by a large range of anesthetic molecules with potencies predicted by the Meyer-Overton correlation (see Fig. 11.2B ). This concept was strengthened by demonstration of stereoselective anesthetic effects, which imply specific anesthetic chiral binding sites.
Diversity of Molecular Targets
Anesthesia is a composite pharmacologic endpoint that is commonly defined as reversible amnesia, loss of consciousness, and immobility. It is not surprising then that these diverse effects result from multiple mechanisms, and that the relative potency and efficacy of various general anesthetics in producing each of these endpoints vary between specific anesthetics ( Fig. 11.3 ). The prevailing view is that inhaled anesthetics have multiple, agent-specific effects on a number of molecular targets critical to neuronal communication and excitability, as summarized in Table 11.1 . These multiple actions work in concert or individually to produce the pleiotropic effects characteristic of inhaled anesthetics.

Level | Site | Effect | Target(s) |
---|---|---|---|
Proteins | Amphipathic binding sites | Conformational flexibility, ligand binding | Ion channels, receptors, signaling proteins |
Action potential | Nervous system | Small reduction in amplitude | Na + channels |
Cardiovascular system | Reduced amplitude, duration | Ca 2+ channels, K + channels | |
Synaptic transmission | |||
Inhibitory | Presynaptic terminal | Enhanced transmitter release | |
Postsynaptic receptors | Enhanced transmitter effects | Glycine, GABA A receptors | |
Excitatory | Presynaptic terminal | Reduced transmitter release | Na + channels, Ca 2+ channels, K 2P channels |
Postsynaptic receptors | Reduced transmitter effects | NMDA receptors, nicotinic acetylcholine receptors | |
Neuronal networks | Neuronal circuit | Altered long-term potentiation/depression | Synaptic plasticity |
Neuronal integration | Altered rhythmicity, coherence | HCN channels, K 2P channels, extrasynaptic GABA A receptors, and so on | |
Central nervous system | Neocortex, hippocampus, amygdala | Sedation, amnesia | θ rhythms, γ rhythms, synchrony |
Diencephalon (thalamus), brainstem (reticular formation) | Unconsciousness | γ-band transfer entropy? Thalamic deafferentation? | |
Spinal cord | Immobility | Nocifensive reflex | |
Cardiovascular system | Heart | ||
Myocardium | Negative inotropy | Excitation-contraction coupling | |
Conduction system | Dysrhythmias | Cardiac action potential | |
Vasculature | Vasodilation | Direct and indirect vasoregulation | |
Pulmonary system | Vasculature | Vasodilation, reduced | Direct and indirect vasoregulation |
Bronchioles | Bronchodilation | Direct and indirect bronchiolar smooth muscle tone |
Criteria for identifying the molecular targets of inhaled anesthetics are shown in Table 11.2 . Volatile anesthetics at clinically relevant concentrations positively modulate inhibitory GABA A receptors and inhibitory glycine receptors, inhibit excitatory NMDA-type glutamate receptors and neuronal nicotinic acetylcholine receptors (nAChRs), activate certain two-pore domain potassium (K 2P ) and leak potassium ion (K + ) channels, and inhibit multiple voltage-gated sodium ion (Na + ) channel (Na v ) and Ca 2+ channel (Ca v ) subtypes. The gaseous inhaled anesthetics (cyclopropane, N 2 O, xenon) at clinically relevant concentrations do not affect GABA A receptors but block NMDA receptors and activate K 2P channels.
|
Putative anesthetic targets are not distributed evenly throughout the nervous system. For example, multiple subtypes of GABA A receptors exist, each with its own expression profiles at the regional, cellular, and subcellular levels. Specific receptor isoforms appear to mediate various anesthetic endpoints, as shown for certain intravenous anesthetics (see Chapter 9 ).
Multiple Behavioral Endpoints
Anesthesia is a drug-induced comatose state with multiple behavioral components, including the essential triad of unconsciousness, amnesia, and immobility; in some cases analgesia and autonomic stability occur, but these endpoints are not universal. These effects are dose-related: amnesia at low doses is followed by sedation, unconsciousness, and eventually immobility ( Fig. 11.4A ). Current evidence favors the hypothesis that the multiple behavioral endpoints of anesthesia result from multiple distinct molecular targets and distinct anatomic sites of action (see Fig. 11.4B ). Unconsciousness is likely due to enhanced inhibition at multiple sites in the cerebral cortex, thalamus, and brainstem involved in arousal. Although the neuronal correlates of consciousness remain unclear, it has been proposed that anything that sufficiently perturbs activity in thalamocortical loops, and hence long-range cortical connectivity, could disorder consciousness. Moreover, individual lesions to brainstem arousal areas and intralaminar thalamus result in transient coma, suggesting that pharmacologic inhibition of these areas is also likely to induce a state of unarousable unresponsiveness.

Amnesia is likely due to altered neurotransmission in the frontal cortex, hippocampus, and amygdala critical to memory acquisition and storage. Immobility is due to effects on spinal cord networks, likely through suppression of central pattern generators critical to coordinated movement. Analgesia is likely due to disruption of spinothalamic tract transmission and possible effects on peripheral nociception. A shortcoming of the volatile anesthetics is their poor control of the autonomic nervous system response to painful stimuli. In the modern era, inhalation anesthesia is routinely supplemented with opioids to achieve optimal anesthetic conditions.
Molecular and Cellular Sites of Action
Because of the ability to precisely define and monitor immobilization, this anesthetic endpoint has been investigated more completely than amnesia and unconsciousness. Inhaled anesthetics produce immobilization at increasing doses primarily via effects on the spinal cord. A series of elegant experiments in vivo showed that the potency of volatile anesthetics for immobilization was determined by effects on the spinal cord. Volatile anesthetic immobility does not appear to be mediated by GABA A receptor effects. Thus the GABA A receptor antagonist bicuculline does not antagonize the immobility induced by isoflurane. This is in contrast to the intravenous anesthetics propofol and etomidate, whose immobilizing effects are mediated primarily by GABA A receptors, as evident in their effective antagonism by bicuculline (see Chapter 10 ). In contrast, mice harboring mutations that reduce sensitivity of GABA A receptor α 1 or β 3 subunits to volatile anesthetics exhibit only slight resistance to the immobilizing and hypnotic effects of volatile anesthetics, whereas analogous mutations that greatly reduce the effects of propofol or etomidate on the β 3 GABA A receptor subunit essentially abolish their hypnotic and immobilizing effects. This indicates that other targets likely play a more important role in these volatile anesthetic actions.
The amnestic effect of volatile anesthetics, like that of the intravenous anesthetics, probably involves potentiation of specific GABA A receptor subtypes ( Fig. 11.5 ). For example, amnesia during anesthesia has been attributed to enhanced activation of α 5 -subunit–containing GABA A receptors, which are known to regulate hippocampal-dependent synaptic plasticity and short-term memory. Long-term potentiation (LTP), a cellular model of hippocampal memory, is suppressed by isoflurane, an effect that is reversed by the GABA A receptor antagonist bicuculline. The finding that both β 3 – and α 4 -subunit knockout mice are resistant to the amnestic effects of isoflurane indicates a role for GABA A receptors containing these subunits in the amnestic action of volatile anesthetics.

Glycine receptors, members of the ligand-gated ion channel superfamily that are closely related to GABA A receptors both structurally and functionally, are likewise potentiated by volatile anesthetics (see Fig. 11.5 ). Their high level of expression in the spinal cord makes glycine receptors putative targets for the immobilizing effects of anesthetics. Partial antagonism of the immobilizing effect of isoflurane by the glycine receptor antagonist strychnine provides pharmacologic evidence supporting a contribution to this anesthetic endpoint.
Neuronal nAChRs are, like GABA A and glycine receptors, pentameric cys-loop ligand-gated ion channels, but in contrast they are cation-selective excitatory channels (rather than anion-selective inhibitory channels) and are inhibited (rather than potentiated) by volatile anesthetics. Mecamylamine (an antagonist of nAChRs) does not affect MAC for several inhaled anesthetics, suggesting that nAChRs do not contribute to inhaled anesthetic immobility. However, they might contribute to the amnestic and hyperalgesic effects of very low doses of volatile anesthetics. This is consistent with the roles of nAChRs in learning and memory in that isoflurane and nonimmobilizers, both of which produce amnesia, block nAChRs.
Voltage-gated Na + channels are integral to all facets of central nervous system (CNS) function and thus are well positioned to mediate multiple aspects of general anesthesia. Clinical concentrations of volatile anesthetics inhibit Na + channels in isolated rat nerve terminals and neurons. Although not as sensitive to volatile anesthetics as cys-loop ligand-gated ion channels, Na + channels are inhibited in vitro in a clinical concentration range. Moreover, reductions in neurotransmitter release from presynaptic terminals by volatile anesthetics involve inhibition of presynaptic action potentials through Na + channel blockade. The presynaptic effects of isoflurane are selective for inhibition of release of the excitatory transmitter glutamate over the inhibitor transmitter GABA, consistent with their neurodepressive actions. Intrathecal administration of the specific Na + channel blocker tetrodotoxin reduces MAC in rats, whereas the activator veratridine increases MAC, supporting a role for Na + channel block in immobility produced by isoflurane. Voltage-gated Ca 2+ channels, which are structurally homologous to Na + channels, have also been implicated as targets for volatile anesthetics, and for isoflurane-induced immobility in particular. These channels mediate Ca 2+ entry in excitable tissues and are critical to many anesthetic-sensitive functions such as synaptic transmission and cardiac contractility.
There is also evidence for K + channels as targets for inhaled anesthetics. The MAC of chloroform, desflurane, halothane, and sevoflurane is variably increased in a knockout of the TREK-1 K 2P K + channel. This variable effect on MAC is not paralleled by the effect of these anesthetics to increase channel opening in vitro, which argues against an exclusive causal connection between TREK-1 and anesthetic effects. N 2 O, xenon, and cylopropane also activate TREK-1. The MAC of halothane is increased significantly by knockout of the TASK-3 channel, but the MAC of isoflurane is not. Increased pH opens TASK K + channels, but decreases in arterial partial pressure of carbon dioxide (Pa co 2 ) (with associated increases in pH) do not decrease MAC. Reduced pH blocks TASK channels, but increases in Pa co 2 that decrease pH also decrease MAC. Finally, intravenous and intrathecal infusions of the KCNK-type K + channel activator riluzole decrease isoflurane MAC equipotently in rats, which suggests that although activation of K + channels might affect anesthetic requirement, the effect is primarily on higher centers rather than the spinal cord. The issue is complicated by the nonspecificity of riluzole, which blocks slowly inactivating Na + channels (important for maintaining repetitive firing, and thus likely MAC) even more potently than KCNK channels.
Blockade of receptors for glutamate, the principal excitatory neurotransmitter in the CNS, provides a plausible mechanism for depression of excitatory synaptic transmission and thereby CNS depression by inhaled anesthetics. NMDA-type and α-amino acid-3-hydroxy-5-methyl-4-isoxazole (AMPA)-type receptors are critical for all forms of synaptic function and plasticity, including learning, memory, and nociception among other roles. Blockade of NMDA receptors, as well as hyperpolarization-activated cyclic nucleotide-gated 1 (HCN1) channels, appears important to the anesthetic mechanisms of ketamine (see Chapter 10 ), and might contribute to inhaled anesthetic effects as well. Isoflurane and xenon inhibit NMDA and AMPA receptors in vitro due to competitive blockade of the glycine coagonist site. In addition to K 2P channels and nAChRs, NMDA receptors are among the most sensitive targets in vitro to N 2 O and xenon and are therefore important to their anesthetic actions, although this conclusion awaits confirmation in vivo. Thus N 2 O, xenon, and cyclopropane have a distinct pharmacologic profile with little or no effect on GABA A receptors but strong inhibition of NMDA and activation of the K 2P channel TREK-1.
Compared with the intravenous anesthetics (see Chapter 10 ), inhaled anesthetics are generally more promiscuous in their molecular targets, lacking the receptor-specific, and sometimes receptor subtype–specific, effects of certain intravenous anesthetics. This complicates their pharmacologic characterization, but might also provide considerable redundancy contributing to their marked efficacy across phyla and within species. Such redundancy might also explain the relatively minor effects on anesthetic potency in vivo of mutations that reduce sensitivity of specific molecular targets to inhaled anesthetics in vitro. It is likely that actions on multiple targets rather than a single specific target contribute to the immobilizing effect of volatile anesthetics.
Drug Class Effects
Inhaled anesthetics are characterized by their diverse chemical classes, including the volatile ethers and alkanes, and the gases N 2 O and xenon. The molecular effects of inhaled anesthetics are also diverse in that their low potency leads to pleiotropic interactions with multiple molecular targets. This includes a large number of systemic (nonanesthetic) effects. It is instructive to consider the effects of the volatile ethers and alkanes, which exert predominantly GABAergic effects ( Fig. 11.6 ), separately from the gases ( Fig. 11.7 ), which exert predominantly NMDA blocking effects (see Fig. 11.3 ).


Nonanesthetic Effects
Bronchodilation
Volatile anesthetics relax airway smooth muscle through block of voltage-gated Ca 2+ channels, depletion of Ca 2+ stores in the sarcoplasmic reticulum, and possibly through potentiation of GABAergic mechanisms. Because the airways normally have low tone, this effect is evident almost exclusively in the setting of bronchospasm. Baseline pulmonary resistance and dynamic pulmonary compliance are unchanged by 1 or 2 MAC halothane, enflurane, isoflurane, or sevoflurane. However, in subjects challenged with intravenous histamine, volatile anesthetics attenuate the increase in pulmonary resistance. Unlike other volatile anesthetics, this effect is not observed with desflurane. Volatile anesthetics also have antiinflammatory effects on airway epithelial cells mediated by GABA A receptor potentiation.
Neuromuscular Effects
In contrast to intravenous anesthetics, which can either have excitatory myoclonic effects or produce weak myorelaxation (propofol), volatile anesthetics produce dose-dependent skeletal muscle relaxation and potentiate both depolarizing and nondepolarizing neuromuscular blockers. The mechanisms of skeletal muscle relaxation include decreasing the depolarizing current at the neuromuscular junction in response to acetylcholine owing to direct inhibition of nAChRs and enhancement of spinal cord glycine receptors. Potentiation of nondepolarizing neuromuscular blocker effects is likely due to potentiation of antagonist affinity at the nAChR. Residual volatile anesthetic can potentiate residual neuromuscular blockade, while elimination facilitates recovery from neuromuscular blockade.
Analgesia and Neuroprotection
Inhibition of NMDA receptors by N 2 O, xenon, and cyclopropane probably underlies their unique analgesic properties, and their propensity to produce excitement and euphoria, and therefore abuse as psychotomimetics. In addition, NMDA receptor blockers including xenon and N 2 O are both neuroprotective and neurotoxic in animal models (see the “Emerging Developments” section ).
Adverse Effects
The promiscuous pharmacologic actions of inhaled anesthetics result in numerous undesirable effects summarized in the following text by system.
Respiratory Depression
All halogenated ethers cause dose-dependent respiratory depression. Even subanesthetic concentrations blunt both the hypoxic and hypercarbic ventilatory responses, though in general hypoxic drive is more sensitive. The acute hypoxic response can remain suppressed for several hours after anesthesia owing to depressant effects of subanesthetic concentrations (0.1 MAC). These effects are multifactorial. The peripheral drive from hypoxia detected by the carotid bodies is blunted. There is a general depression of the central respiratory centers and arousal centers, and motor neuron and respiratory muscle function is suppressed, both leading to reduced respiratory drive and upper airway muscular tone and patency. Minute ventilation is reduced at anesthetic doses mainly through a reduction in tidal volume, while respiratory rate is maintained or increased. These effects are mediated primarily centrally through enhanced postsynaptic inhibitory GABAergic and depressed presynaptic and postsynaptic excitatory glutamatergic transmission.
Hypoxia is sensed by glomus type I cells of the carotid bodies by reduced opening of K + channels, although the specific type is difficult to determine and might be species dependent. Volatile anesthetic suppression of peripheral hypoxic respiratory drive might be mediated by reactive oxygen species (ROS), altering the redox state of K + channels that detect hypoxia. Halothane, with a relatively large degree of peripheral metabolism and associated production of ROS, dramatically suppresses the carotid body response to hypoxia. Desflurane, with minimal metabolism, minimally suppresses the carotid body reflex. Isoflurane, with an intermediate degree of metabolism, moderately inhibits the carotid bodies. Pretreatment with a mixture of antioxidants prevents suppression of the respiratory response by subanesthetic doses of halothane and isoflurane.
Cardiovascular Depression
Volatile anesthetics universally produce concentration-dependent myocardial depression, especially halothane. This is due primarily to altered Ca 2+ entry and sarcoplasmic reticulum Ca 2+ handling. The most significant cardiodepressant effect of halothane is direct myocardial depression. The negative inotropy is compounded by decreases in systemic vascular resistance (SVR) by enflurane, isoflurane, desflurane, and sevoflurane to further reduce blood pressure. In contrast, N 2 O and especially xenon have minimal direct cardiovascular effects ( Table 11.3 ). Xenon does not affect the major cation currents in isolated cardiomyocytes, which probably explains the minimal cardiovascular depression. Reduced SVR is most prominent with isoflurane, leading to suggestions that it could promote a steal phenomenon in patients with coronary artery disease. However, there is no convincing clinical evidence that isoflurane is riskier than other inhaled anesthetics in patients with coronary artery disease as long as perfusion pressure is maintained. Rapid increases in inhaled concentrations of isoflurane and especially desflurane can lead to increases in heart rate and blood pressure secondary to sympathetic activation as the result of an irritant effect. Sevoflurane has minimal effects on heart rate.
Cardiac Output | System Vascular Resistance | Mean Arterial Pressure | Heart Rate | |
---|---|---|---|---|
Halothane | ↓ | ↔ | ↓ | ↓↓ |
Enflurane | ↓↓ | ↓ | ↓↓ | ↑ |
Isoflurane | ↓ | ↓ | ↓↓ | ↑ |
Desflurane | ↔ | ↓ | ↓ | ↑ |
Sevoflurane | ↔ | ↓ | ↓ | ↔ |
Nitrous oxide | ↓ | ↑ | ↔ | ↑ |
Xenon | ↔ | ↔ | ↔ | ↓ |
Cardiac Dysrhythmias
Isoflurane, desflurane, and sevoflurane all prolong the electrocardiographic QT interval, potentially increasing the risk of torsades de pointes polymorphic ventricular tachycardia. There is some controversy as to whether halothane presents a similar risk. Prolongation of the QT interval is likely due to inhibition of the delayed rectifier K + current through human ether-à-go-go–related gene (hERG) channels that normally contribute to cardiomyocyte repolarization (see Chapter 27 ). Halothane and cyclopropane are well known for myocardial sensitization to catecholamine-induced arrhythmias. This has been proposed to result from disruption of muscarinic regulation of myocardial adenylyl cyclase. These arrhythmias are suppressed by calcium channel blockers and β-adrenergic blockers. The ether anesthetics, xenon, and N 2 O have minimal proarrhythmic effects.
Neurophysiologic Effects
Volatile anesthetics decrease the cerebral metabolic rate of oxygen consumption (CMRO 2 ; see Chapter 9 ). Because of flow-metabolism coupling this should result in reduced cerebral blood flow (CBF) and intracranial pressure. However, these effects are offset by the direct vasodilation effect of volatile anesthetics on cerebral vasculature, particularly at higher doses. N 2 O increases CBF with a mild increase in CMRO 2 . Coadministration of propofol, barbiturates, or opioids counteracts these vasodilatory effects.
Volatile anesthetics lead to characteristic changes in the electroencephalogram (EEG; see Chapter 8 ). During quiet wakefulness, α and β activity predominate. As anesthetic concentration increases, the EEG shifts to larger-amplitude, lower-frequency activity in the θ and δ range. As concentration increases further, the EEG shows burst suppression, alternating periods of isoelectric activity, and bursts of activity. By the time concentration reaches 1.5 to 2 MAC, the EEG becomes isoelectric. These characteristic shifts in the EEG power spectrum have been exploited to create multiple measures of depth of anesthesia, including the bispectral index (BIS), spectral entropy, and spectral edge. Unfortunately, none has proven to be a compelling monitor for awareness, in that the BIS or Anesthetic Gas to Reduce Explicit Recall (BAG-RECALL) trial failed to demonstrate superiority for awareness prevention when following BIS compared to a protocol to maintain a minimum end-tidal volatile anesthetic concentration. Ketamine and N 2 O do not reduce the BIS or suppress midlatency auditory evoked potentials, whereas xenon does. Thus anesthetic depth monitors are inaccurate when ketamine or N 2 O is used.
Sevoflurane, isoflurane, and enflurane are notable for producing epileptiform EEG activity at high concentrations. This effect is particularly pronounced with hyperventilation and has been detected during pediatric inhalational induction with sevoflurane. This activity is of unclear clinical significance, but it raises concerns of possible neurotoxicity.
Immunomodulatory Effects
Postoperative immunocompromise in surgical patients is primarily related to the neuroendocrine stress response to surgery through activation of the autonomic nervous system and the hypothalamic-pituitary-adrenal axis. Monocytes, macrophages, and T cells all have β 2 -adrenoreceptors and glucocorticoid receptors through which the perioperative surge in catecholamines, adrenocorticotropic hormone, and cortisol can directly suppress function. This immunomodulatory effect has the potential for significant impact on postoperative outcome, particularly for cancer surgery, because pain and surgery increase tumor metastasis in cancer surgery when comparing groups that received systemic or epidural analgesia in addition to general anesthesia during surgery. This argues that the immune effects of anesthesia are significantly smaller than the effects of surgery, but volatile anesthetics also have immunomodulatory effects.
Data concerning the immunomodulatory effects of volatile anesthetics are somewhat contradictory, but the majority of studies show that they have immunosuppressive properties (see Chapter 38 ). Volatile anesthetics generally inhibit inflammatory cytokines, either directly or through stimulation of helper T-cell cytokines such as interleukin (IL)-4 and IL-10 that normally function to limit the inflammatory response. Studies have shown decreased lymphocyte proliferation, inhibition of neutrophil function, and suppression of alveolar monocyte responses to cytokines. Additionally, volatile anesthetics reversibly inhibit voltage-gated Ca 2+ channels, decrease intracellular Ca 2+ concentration, and reduce expression of inducible nitric oxide synthase (iNOS) in lymphocytes, while iNOS expression is increased in alveolar macrophages exposed to isoflurane.
Volatile anesthetics inhibit human neutrophil bacterial killing in vitro by reducing production of ROS. The resulting decrease in ROS suppresses the initial inflammatory response by directly limiting neutrophil-mediated local tissue injury, which itself is responsible for upregulation of endothelial adhesion molecules that recruit more immune cells and launch the systemic immune response. Whereas inhibition of neutrophil ROS production could compromise the immune response, neutrophil ROS release is also a significant contributor to the initial tissue injury that is responsible for reperfusion injury.
Hepatotoxicity
Anesthetic-induced hepatitis was first recognized in the 19th century as a specific risk of chloroform but not ether. Hepatotoxicity has been traced to biotransformation of volatile agents by cytochrome P450 enzymes, producing trifluoroacetylated protein antigens (see Chapter 7 ). Halothane-induced hepatitis is most common and is classified into two types. Type I occurs commonly (estimates range from 5% to 30% of patients) and results in asymptomatic elevation of serum transaminases 1 to 2 weeks after exposure. No prior exposure is required, and the mechanism of injury is unclear. Leading explanations include a direct toxic effect of halothane itself or an indirect effect as halothane metabolism produces free radicals that interact with cellular components, leading to an autocatalytic peroxidative chain reaction.
Type II halothane hepatitis is much less common (1 in 35,000 halothane anesthetics). An immune-mediated response directed against hepatocytes leads to fulminant hepatitis with high mortality. In most cases there is prior exposure to halothane. Delayed-onset jaundice or postoperative fever is also common. The presentation can be delayed up to 1 month following anesthesia. Cytochrome P450 2E1 (CYP 2E1) oxidation of halothane produces trifluoroacetyl chloride, which is highly reactive and covalently binds to hepatic proteins, producing trifluoroacetylated protein neoantigens that initiate the immune response responsible for the hepatitis. The trifluoracetylated sites act as haptens to establish an antibody reaction, and serum from patients with type II halothane hepatitis contains antibodies that react with acyl halides bound to liver proteins. Patients also develop autoantibodies against CYP 2E1, but the same autoantibodies have been found in unaffected pediatric anesthesiologists who were routinely exposed to halothane during inhalational inductions, suggesting that these CYP 2E1 antibodies do not have a pathogenic role.
Nephrotoxicity
There are two potential pathways by which volatile anesthetics contribute to nephrotoxicity, either by metabolism leading to inorganic fluoride production or via degradation products, as described earlier for sevoflurane. Fluoride production has a well-documented history of inducing nephrotoxicity, whereas the other degradation product pathways lack reported clinical significance in humans.
Methoxyflurane was withdrawn after reports of severe nephrotoxicity owing to production of inorganic fluoride. A high-output renal failure unresponsive to vasopressin develops in association with serum fluoride concentrations in excess of 50 µM. Sevoflurane is the modern anesthetic with the highest associated serum fluoride concentrations, with some reports of serum levels exceeding 50 µM after as little as 2 MAC hours of exposure, although nephrotoxicity from sevoflurane exposure has not been reported and controlled studies have failed to detect an impact of sevoflurane anesthesia on renal function. This is likely secondary to the metabolism of methoxyflurane by cytochrome P450 enzymes 2E1, 2A6, and 3A4 within the kidney, producing fluoride locally within the nephrons themselves. In contrast, there is less renal metabolism of sevoflurane. Sevoflurane metabolism leads to formation of compound A, a nephrotoxic vinyl halide (see Chapters 4 and 7 ). However, the dose-dependent nephrotoxicity of compound A has been demonstrated only in rats and multiple human studies have not shown evidence of renal impairment.
Malignant Hyperthermia
Malignant hyperthermia is an uncommon inherited genetic disorder of skeletal muscle that can produce a potentially lethal hypermetabolic state after certain triggers (see Chapter 7 ), which include all the volatile anesthetics, but not N 2 O or xenon. A trigger leads to excessive release of Ca 2+ from intracellular stores, which leads to muscle rigidity and rhabdomyolysis. The associated hypermetabolism increases body temperature, CO 2 production, and O 2 consumption. If left untreated, malignant hyperthermia has an estimated mortality rate of 80%. Mortality is reduced to near 10% by early dantrolene treatment to terminate the hypermetabolic reaction by blocking intracellular Ca 2+ release and supportive care to manage the sequelae of rhabdomyolysis. A molecular lesion underlying malignant hyperthermia was first traced to a single point mutation in the ryanodine receptor RyR1 in pigs, but it is now known that a variety of mutations, most commonly in RyR1 but also in the α 1 subunit of the dihydropyridine receptor (Cav2.3) and rarely in calsequestrin or sarcoplasmic endoreticular calcium ATPase (SERCA), can lead to this pharmacogenetic reaction (discussed further in Chapter 7 ).
Postoperative Nausea and Vomiting
All inhaled anesthetics are emetogenic, including the volatile anesthetics, N 2 O and xenon; there are no significant differences between the modern volatile ether inhaled agents in their propensity for inducing postoperative nausea and vomiting (PONV). General anesthesia increases the risk of PONV significantly relative to regional anesthesia techniques (see Chapter 34 ). The incidence of PONV following general inhalational anesthesia involving the use of two triggering agents (opioids and volatile anesthetics) is 25% to 30%. Substituting propofol, which is not emetogenic (but rather is antiemetic) for the volatile anesthetic reduces the relative risk of PONV by 19%, and using nitrogen as a carrier gas rather than N 2 O reduces the relative risk by 12%.
Metabolic Effects
N 2 O can produce megaloblastic bone marrow changes and neurologic complications owing to vitamin B 12 deficiency (see Chapter 7 ). The cobalt ion in vitamin B 12 is oxidized, which inhibits the activity of methionine synthase, which is responsible for conversion of homocysteine to methionine, thymidine, and tetrahydrofolate. Accumulation of plasma homocysteine could in theory increase the risk of perioperative myocardial events, but its clinical significance is unlikely.
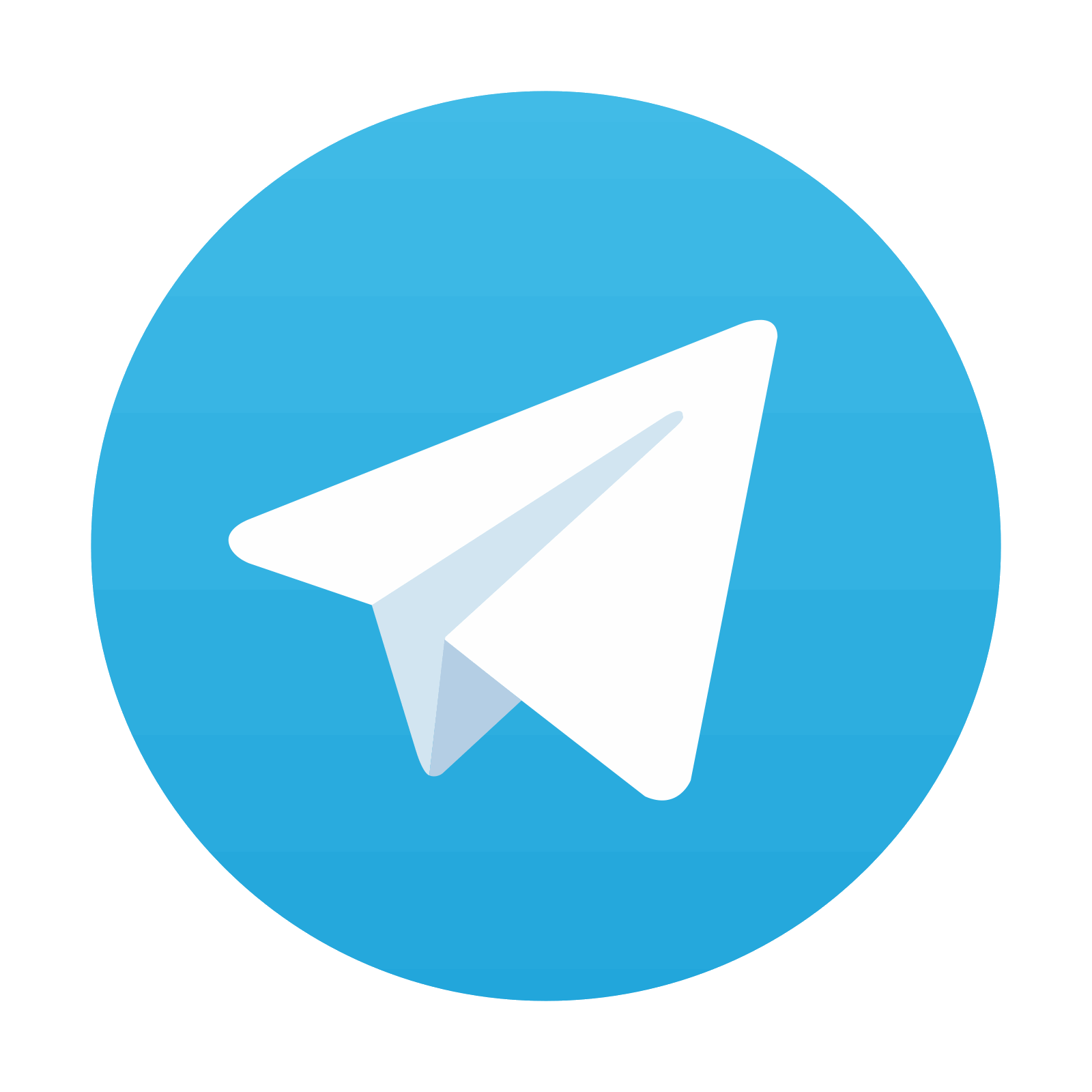
Stay updated, free articles. Join our Telegram channel
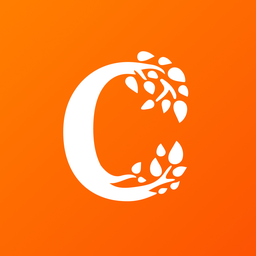
Full access? Get Clinical Tree
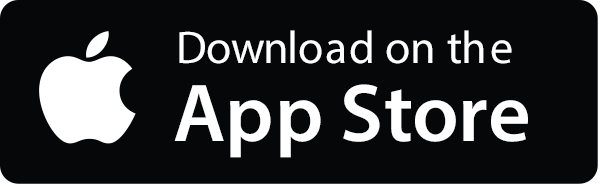
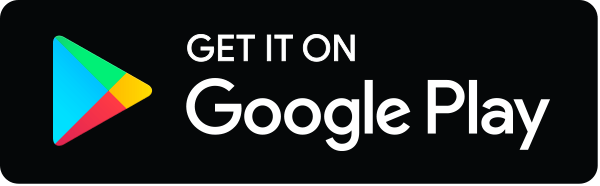