165 Adrenal Insufficiency
Historical Review
In the same decade, Hench and Kendall, two rheumatologists at the Mayo Clinic, found that in patients with different forms of rheumatism, the symptoms showed temporary remissions during pregnancy and inflammatory diseases like hepatitis. They speculated that this might be due to a general stimulation of the endocrine system and concluded that the use of cortisone might be beneficial in patients with acute rheumatoid arthritis. In September 1948, a female bedridden patient with severe and painful rheumatism that was resistant to all standard therapies at the time was the first documented case of cortisone treatment for inflammatory disease. After 3 days, the patient was able to stand up; 1 week later, she left the clinic without pain and on her own feet. Retrospectively, the speculations regarding pregnancy and hepatitis were obviously wrong, but the antiinflammatory character of cortisone was a key finding in pharmaceutical research. In contrast to Selye, who described cortisone as a crucial promoter of the physiologic stress response, the aforementioned finding that the adrenal gland cortex is the location for endogenous production of cortisone, an important inhibitor of stress and inflammation, has been confirmed. In 1950, Kendall, Hench, and Reichstein received the Nobel Prize in Medicine for their historical findings on the physiologic role of the adrenal gland.1–4
Anatomy of the Adrenal Gland
The adrenal cortex receives afferent and efferent innervation. Direct contact of nerve terminals with adrenocortical cells has been suggested, and chemoreceptors and baroreceptors present in the adrenal cortex infer efferent innervation. Diurnal variation in cortisol secretion and compensatory adrenal hypertrophy are influenced by adrenal innervation. Splanchnic nerve innervation has an effect in regulating adrenal steroid release. The adrenal medulla secretes the catecholamines, epinephrine and norepinephrine, that affect blood pressure, heart rate, sweating, and other activities also regulated by the sympathetic nervous system. The adrenal cortex is divided into three layers: (1) the zona glomerulosa, just under the capsule, (2) the zona fasciculata, the middle layer, and (3) the zona reticularis, the innermost net-like patterned area with reticular veins draining into medullary capillaries. The zona glomerulosa exclusively produces the mineralocorticoid, aldosterone; the zonae fasciculate and reticularis produce glucocorticoids and androgens.5
Physiology of the Hypothalamic-Pituitary-Adrenal Axis
The adrenal glands are part of a complex system that produces interacting hormones to maintain physiologic integrity, especially during the stress response.6,7 This system, the hypothalamic-pituitary-adrenal (HPA) axis, includes the hypothalamic region which produces corticotropin-releasing hormone (CRH), triggering the pituitary gland. The pituitary gland is composed of two major structures: the adenohypophysis (anterior pituitary) and neurohypophysis (posterior pituitary). The anterior pituitary is responsible for the secretion of corticotropin (adrenocorticotropic hormone [ACTH]), thyroid-stimulating hormone (TSH), growth hormone (GH), β-lipotropin, endorphins, prolactin, luteinizing hormone (LH), and follicle-stimulating hormone (FSH). The posterior pituitary secretes vasopressin (antidiuretic hormone [ADH]) and oxytocin. Corticotropin regulates the production of corticosteroids by the adrenal glands. Hypothalamic neurons receive input from many areas within the CNS; they integrate these inputs and initiate an output to the anterior pituitary via the median eminence. The median eminence secretes releasing hormones into a hypophyseal portal network of capillaries that connect the median eminence with the pituitary hormones.
The HPA axis is stimulated not only by physical or psychic stress but also by peptides like ADH and cytokines. Thus, the HPA axis plays an important role during infections and immunologic disorders.8,9 By interaction with the renin-angiotensin-aldosterone system (RAAS) regulating fluid and salt balance, synthesis of androgens (e.g., dehydroepiandrosterone) with possible impact on immunomodulation, and the sympathoadrenergic system, the HPA axis is probably the most important organ of stress response. Stimulation of the immune system by infections induces the release of proinflammatory cytokines like tumor necrosis factor alpha (TNF-α), interleukin (IL)-1β, or IL-6. Following a cascade, these cytokines stimulate both the hypothalamus and the anterior pituitary gland, which finally leads to the release of glucocorticoids. IL-6 is also able to induce steroid release directly from the adrenal gland. The adequate increase of glucocorticoid levels during inflammation is a crucial factor for appropriate stress response. In acute infections, this release maintains metabolic and energy integrity. If the process is chronic, the HPA axis develops an adaptation which induces typical clinical manifestations such as hypercatabolic states, hyperglycemia, and suppression of androgens and growth and thyroid hormones. These changes, however, may increase the risk for secondary infections. Increased cortisol levels suppress higher regulatory levels of the HPA axis in terms of a negative feedback loop. Hence, after major surgery or during sepsis and septic shock, high cortisol and low ACTH levels are detectable.10,11 Even the infusion of dexamethasone or CRH is not able to suppress increased cortisol levels in these patients.12,13 This phenomenon leads to the question of how cortisol release is induced. Several investigations demonstrated that adrenal cortisol synthesis in critically ill patients is not regulated by ACTH, but by paracrine pathways via endothelin, atrial natriuretic peptide (ANP), or cytokines like IL-6.14–16 IL-6 directly induces the adrenal cortex to release cortisol, which in chronic courses, can worsen the prognosis.17
Cellular Response to Adrenocortical Hormones and Related Drugs
Cortisol, the major free circulating adrenocortical hormone, is a hydrophobic hormone; being a steroid, it circulates bound to protein. Complexed cortisol-binding globulin (CBG, or transcortin) accounts for about 95% of circulating cortisol, but only the free form is biologically active. Its plasma half-life is 60 to 120 minutes. Cortisol is metabolized by hydroxylation in the liver, and the metabolites are excreted in urine. Steroid hormones enter the cytoplasm of cells where they combine with a receptor protein. Metabolic, immunologic, and hemodynamic responses to adrenocortical steroid hormones are regulated in a very complex manner that includes transactivation, transrepression, posttranscriptional/translational regulation, and nongenomic effects. The immediate nongenomic effects of steroid hormones were primarily attributed to mineralocorticoids (aldosterone). Rapid activation of the sodium-proton exchanger, increase of intracellular Ca++, and activation of second messenger pathways were described.18,19 A randomized trial in patients during cardiac catheterization revealed that within minutes after aldosterone injection, cardiac index and arterial pressure increased significantly for 10 minutes and returned to baseline afterwards.20 Interestingly, the genomic effects of aldosterone seemed to be mediated by binding to glucocorticoid (GC) receptors and not to mineralocorticoid receptors.21 There is evidence that GC, like cortisol, also modulates immune functions by rapid nongenomic effects via nonspecific interactions with cellular membranes and specific binding to membrane-bound GC receptors (GR).22 Nonspecific membrane effects have been demonstrated for inhibition of sodium and calcium cycling across plasma membranes by impairing Na+/K+-ATPase and Ca++-ATPase. Moreover, the rapid activation of lipocortin-1 and inhibition of arachidonic acid release after GC was independent from GR translocation. Finally, high-sensitivity immunofluorescence staining revealed membrane-bound GR on circulating B lymphocytes and monocytes.22
The multiple mechanisms by which GCs modulate cellular responses include mainly genomic pathways.23–25 Nongenomic effects are thought to account for immediate immune effects of high doses of GC, whereas membrane-bound receptors probably mediate low-dose GC effects. The classic model is that GCs bind to the cytoplasmatic ligand-regulated GC receptor alpha (GRα), which is an inactive multiprotein complex consisting of two heat shock proteins (hsp90) acting as molecular chaperones and other proteins (Figure 165-1). Upon GC binding to GRα, conformational change causes dissociation of hsp90, with subsequent nuclear translocation of GRα homodimers, binding of GRα to GC response elements (GRE) of DNA, and transcription of responsive genes (transactivation) such as lipocortin-1 and β2-adrenoreceptors. Alternatively, GRα may bind to negative GRE (nGRE) and repress transcription of genes (transrepression) such as pro-opiomelanocortin (POMC). More importantly, transrepression without direct binding of GRα to GRE by protein-protein interactions of GRα with transcription factors, nuclear factor kappa B (NF-κB) and AP-1, has been recognized as a key step by which GC suppress inflammation,26 inhibiting synthesis of TNF-α, IL-1β, IL-2, IL-6, IL-8, inducible nitric oxide synthase (iNOS), cyclooxygenase (COX)-2, cell adhesion molecules, and growth factors, and promoting apoptosis.27 In addition, NF-κB repression may be mediated by GC-induced up-regulation of the cytoplasmatic NF-κB inhibitor, IκBα (see Figure 165-1) which prevents translocation of NF-κB.28 Clinical investigations provide support for the presence of endogenous GC inadequacy in the control of inflammation and peripheral GC resistance.29 With GC treatment, the intracellular relations between the NF-κB and GRα signaling pathways change from an initial NF-κB-driven and GRα-resistant state to a GRα-sensitive one. However, data are conflicting and probably do not explain early (<2 hours) suppressive effects of GC but may account for the longer-term dampening effect of GC on inflammatory processes.23
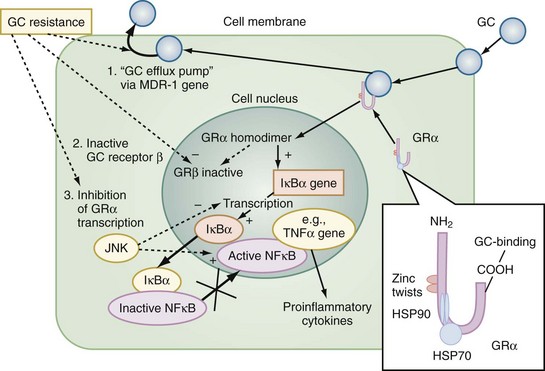
Figure 165-1 Cellular mechanisms of glucocorticoid effects (right) and glucocorticoid resistance (left).
Besides transcriptional regulation, posttranscriptional, translational, or posttranslational processes have been described for GC-induced modulation of COX-2, TNF-α, GM-CSF, IL-1β, IL-6, IL-8, and interferon gamma (IFN-γ).23 Furthermore, GCs act at multiple levels to regulate iNOS expression by decreased iNOS gene transcription and mRNA stability; reduced translation and increased degradation of the iNOS protein by the cysteine protease, calpain30; limitation of the availability of the NOS cofactor, tetrahydrobiopterin; reduced transmembranous transport and de novo synthesis of the NOS substrate, L-arginine; and lipocortin-1-induced inhibition of iNOS.31,32 Together, these complex mechanisms result in the considerable effect of GC to inhibit inflammation and to stabilize hemodynamics. Finally, GC receptors have been found in nearly every nucleated cell in the body, and since each cell type has its own expression of GC effect, it follows that GCs have many effects in the body, which is equally true of endogenously produced GC hormones or exogenously administered GC medications. Both increase hepatic production of glucose and glycogen and decrease peripheral use of glucose. Steroids also affect fat and protein metabolism. They increase lipolysis both directly and indirectly by elevating free fatty acid levels in the plasma and enhancing any tendency to ketosis. GCs further stimulate peripheral protein metabolism, using the amino acid products as gluconeogenic precursors.
Definitions of Adrenal Insufficiency
Adrenal glands may stop functioning when the HPA axis fails to produce sufficient amounts of the appropriate hormones. Primary adrenal insufficiency is defined by the inability of the adrenal gland to produce steroid hormones even when the stimulus by the pituitary gland via corticotropin is adequate or increased. Primary adrenal insufficiency affects 4 to 6 out of 100,000 people. The disease can strike at any age, with a peak between 30 and 50 years, and affects males and females about equally. In 70%, the cause is a primary destruction of the adrenal glands by an autoimmune reaction (“classical” Addison’s disease or autoimmune adrenalitis), with about 40% of patients having a history of associated endocrinopathies. Most adult patients have antibodies against the steroidogenic enzyme, 21-hydroxylase,33 but their role in the pathogenesis of autoimmune adrenalitis is uncertain. In the other 30%, the adrenal glands are destroyed by cancer, amyloidosis, antiphospholipid syndrome, adrenomyeloneuropathy, acquired immunodeficiency syndrome (AIDS), infections (e.g., tuberculosis, cytomegaly, fungi), or other identifiable diseases (Box 165-1). In these cases, the typical morphologic changes of the adrenal cortex are atrophy, inflammation, and/or necrosis. In primary adrenal insufficiency, the whole adrenal cortex is involved, resulting in a deficiency of GCs, mineralocorticoids, and adrenal androgenes.34,35
Secondary adrenal insufficiency is characterized by adrenal hypofunction due to the lack of pituitary ACTH or hypothalamic CRH. Diseases of the anterior pituitary that can cause secondary adrenal insufficiency include neoplasms (e.g., craniopharyngiomas, adenomas), infarction (e.g., Sheehan’s syndrome, trauma), granulomatous disease (e.g., tuberculosis, sarcoidosis), hypophysectomy, and infection.36 Causes also include hypothalamic dysfunction, such as after irradiation or surgical interventions (see Box 165-1). Because aldosterone secretion is more dependent on angiotensin II than on ACTH, aldosterone deficiency is not a problem in secondary adrenal insufficiency. Selective aldosterone deficiency can occur as a result of depressed renin secretion and angiotensin II formation.34 Rare patients have an isolated deficiency of CRH,37 and lymphocytic hypophysitis with subsequent adrenal insufficiency was described in women.38 These disorders may lead to an isolated ACTH deficiency.34
The so-called tertiary adrenal insufficiency, which is often summarized together with secondary forms, commonly occurs after withdrawal of exogenous GCs. Many of these patients do well during normal activities but are unable to mount an appropriate GC response to stress. This effect depends on the dose and duration of treatment and varies greatly from person to person. It should be anticipated in any patient who has been receiving more than 30 mg of hydrocortisone per day (or 7.5 mg of prednisolone or 0.75 mg of dexamethasone per day) for more than 3 weeks.35 If supraphysiologic doses of GCs have been administered to a patient for more than 1 to 2 weeks, the drug should be tapered to allow for adrenal gland recovery. It may take 6 to 12 months for the adrenal glands to recover fully after prolonged use of exogenous GCs.39 Since ACTH is not a major determinant of mineralocorticoid production, the basic deficit in adrenal insufficiency is that of deficient GC production. It is important that neither the dose of applied glucocorticoids, nor the time of treatment, nor the basal plasma level of cortisol allow sufficient assessment of the function of the HPA axis. Some drugs have also been described to induce adrenal insufficiency, either by directly affecting adrenocortical steroid release (e.g., fluconazole, etomidate)40,41 or by enhanced hepatic metabolism of cortisol (e.g., rifampicin, phenytoin).35
Relative Adrenal Insufficiency
The aforementioned forms of adrenal insufficiency which lead to an absolute deficiency of steroids are rare in critically ill patients (0%-3%).42 They are mostly characterized by morphologic changes of the HPA axis. To reflect the notion that subnormal adrenal corticosteroid production during acute severe illness can also occur without obvious structural defects in the HPA axis, deficiency syndromes due to a dysregulation have been termed functional adrenal insufficiency.43 Functional adrenal insufficiency can develop during the course of an illness and is usually transient.35 Decreased levels of GCs are registered much more often; these levels might be sufficient in normal subjects but are too low for stress situations, owing to higher need, and are associated with a worse outcome.44 This led to the concept of relative adrenal insufficiency (RAI). The major cause for RAI is inadequate synthesis of cortisol due to cellular dysfunction. Hence, in contrast to absolute adrenal insufficiency, the morphologic changes in RAI may be minor, sometimes characterized by cellular hyperplasia within the adrenal cortex. This is often combined with peripheral GC resistance of the target cells, which is caused by inflammatory events and aggravates the clinical course, although the absolute cortisol serum levels might be normal.45 In septic shock, RAI may be due to impaired pituitary corticotropin release, attenuated adrenal response to corticotropin, and reduced cortisol synthesis (Figure 165-2).35,46,47 In addition, cortisol transport capacity to effect sites may be reduced, and response to cortisol may be impaired at the tissue level by cytokines modulating GC receptor affinity to cortisol and/or GC response elements.48,49 In clinical trials, it was demonstrated that prolonged treatment of systemic inflammation in patients with severe acute respiratory distress syndrome (ARDS) with methylprednisolone can improve the decreased GC response by increasing the GC receptor affinity and reducing the NF-κB-mediated DNA binding and transcription of proinflammatory cytokines.29 Thus, if RAI can be identified, treatment with supplemental corticosteroids may be of benefit.35 Prevalence of RAI in the critically ill varied from 0% to 77% with different definitions, cutoff values, study populations, and adrenal function tests34,35,46,50,51 and may be as high as 50% to 75 % in severe septic shock.52
Evaluation of Adrenal Insufficiency
In clinical practice, assessment of adrenal function is difficult, especially in critically ill patients, since the diurnal rhythm is lost. Values indicating normal adrenocortical function are listed in Box 165-2. Normally, serum cortisol concentrations in the morning (8 AM) of less than 3 µg/dL (80 nmol/L) are strongly suggestive of absolute adrenal insufficiency,53 while values below 10 µg/dL (275 nmol/L) make the diagnosis likely. Basal urinary cortisol and 17-hydroxycorticosteroid excretion is low in patients with severe adrenal insufficiency but may be low-normal in patients with partial adrenal insufficiency. Generally, baseline urinary measurements are not recommended for the diagnosis of adrenal insufficiency. To differentiate between primary, secondary, and tertiary adrenal insufficiency in cases of low cortisol, it is recommended to measure plasma ACTH concentrations simultaneously. Inappropriately low serum cortisol concentrations in association with increased ACTH concentrations are suggestive of primary adrenal insufficiency, whereas the combination of low cortisol and ACTH concentrations indicates secondary or tertiary disease. This, however, should be confirmed by stimulation of the adrenal gland with exogenous ACTH. In secondary or tertiary adrenal insufficiency, the adrenal glands release cortisol, whereas in primary adrenal insufficiency, the adrenal glands are partially or completely destroyed and do not respond to ACTH.
ACTH stimulation tests usually consist of administering 250 µg (40 International Units) of ACTH (so-called high-dose ACTH stimulation test). For long-term stimulation tests, which are preferred for differentiating between secondary and tertiary adrenal insufficiency, 250 µg of ACTH are infused either over 8 hours or over 2 days.54 Serum cortisol and 24-hour urinary cortisol and 17-hydroxycorticosteroid (17-OHCS) concentrations are determined before and after the infusion. This test may be helpful in distinguishing primary from secondary/tertiary adrenal insufficiency. In primary adrenal insufficiency, there is no or a minimal response of plasma or urinary cortisol and urinary 17-OHCS. Increases of these values in the 2 to 3 days of the test are indicative of a secondary/tertiary cause of adrenal insufficiency. In normal subjects, the 24-hour urinary 17-OHCS excretion increases 3- to 5-fold above baseline. Serum cortisol concentrations reach 20 µg/dL (550 nmol/L) at 30 to 60 min and exceed 25 µg/dL (690 nmol/L) at 6 to 8 hours post initiation of the infusion. Today this is not very often used, because clinical manifestations of adrenal insufficiency combined with basal cortisol levels, short-term ACTH stimulation tests, and CRH tests (see later) usually provide sufficient information.
A short-term stimulation test with 250 µg ACTH, mostly used for patients who are not critically ill, determines basal serum cortisol levels and the induced-response concentration 30 and 60 minutes after intravenous (IV) administration of ACTH. The advantage of the high-dose test is that pharmacologic plasma ACTH concentrations can be achieved by either IV or intramuscular injection.55 This way of application, however, may be too high to identify mild cases of secondary adrenal insufficiency or chronic deficiencies.56 Furthermore, it should not be used when acute secondary adrenal insufficiency (e.g., Sheehan’s syndrome) is presumed, since it takes several days for the adrenal cortex to atrophy, and it will still be capable of responding to ACTH stimulation normally. In these cases, a low-dose ACTH test or an insulin-induced hypoglycemia may be required to confirm the diagnosis.57,58 A rise in serum cortisol concentration after 30 or 60 minutes to a peak of 18 to 20 µg/dL (500 to 550 nmol/L) or more is considered a normal response to a high-dose ACTH stimulation test and excludes the diagnosis of primary adrenal insufficiency and almost all cases of secondary adrenal insufficiency except those of recent onset.59–61
To further differentiate between secondary and tertiary adrenal insufficiency, laboratory investigations may be augmented by a CRH stimulation test. In both conditions, cortisol levels are low at baseline and remain low after CRH. In patients with secondary adrenal insufficiency, there is little or no ACTH response, whereas in patients with tertiary disease, there is an exaggerated and prolonged response of ACTH to CRH stimulation which is not followed by an appropriate cortisol response.62,63 Formerly, the HPA axis was also tested by a stimulated hypoglycemia test. After administering 0.1 units of insulin per kilogram bodyweight, inducing a hypoglycemic state of less than 40 mg/dL serum glucose, an intact HPA axis induces a serum cortisol concentration of more than 20 µg/dL. Nowadays, this procedure is considered obsolete because of the high risk of hypoglycemia.
In critically ill patients, primary causes of absolute or relative adrenal insufficiencies are multiple and often undetectable if no specific hypothesis exists. Volume-resistant septic shock or any other form of life-threatening hypotension with increasing need for catecholamines should give reason to evaluate adrenal function. Formerly, a serum cortisol value less than 20 µg/dL was suggestive for the diagnosis. Meanwhile, it is acknowledged that several factors complicate investigations of the HPA axis in patients with critical illness. A short-term ACTH stimulation test may be performed in critically ill patients suspected of having adrenal insufficiency. However, in most patients, RAI will be present, especially in patients with severe sepsis and septic shock. A clear definition of RAI is still missing, and the pathophysiology is rather complex, which makes it difficult to define clear cutoffs for both basal serum cortisol concentrations and incremental increases after short-term ACTH stimulation tests. Proposed cutoff points may depend on different methods used to measure cortisol, with variations when compared to high-performance liquid chromatography (HPLC) as the reference method.64 In addition, considering free cortisol or increase in free cortisol in response to ACTH could increase accuracy of adrenocortical function tests.48 Furthermore, extrapolating the diagnosis from reference values obtained from healthy people or patients with hypothalamic-pituitary-adrenal disorders may be misleading, since normal or high-normal cortisol concentrations in septic shock may indicate inadequate adrenal response to stress. In a large series of patients, receiver operating characteristic curve (ROC) analysis reached highest sensitivity (68%) and specificity (65%) for a reference value of less than 9 µg/dL (incremental increase) to detect nonresponders.52 Basal cortisol of 34 µg/dL and incremental increase of 9 µg/dL after stimulation were the best cutoff points to discriminate between survivors and nonsurvivors. The higher the basal plasma cortisol and the weaker the cortisol response to corticotropin, the higher was the risk of death. Some investigators have questioned the discriminative power of the incremental increase of cortisol after stimulation in patients with high basal cortisol values, as increases may reflect adrenal reserve more than adrenal function. Hence, RAI was defined based on the hemodynamic response when a randomly measured cortisol was less than 25 µg/dL.46
Routine use of the low ACTH stimulation test in critically ill patients cannot be recommended at present, although the low-dose test is preferred in patients with secondary or tertiary adrenal insufficiency.65 After stimulation with 250 µg ACTH, circulating corticotropin concentrations are 40 to 200 pg/mL during stress but may be as high as 60,000 pg/mL.35 Stimulation of the adrenal gland with low doses of ACTH (1 µg) was shown to increase sensitivity and specificity to detect adrenal insufficiency in patients with hypothalamic-pituitary-adrenal disorders who respond normally to traditional high-dose stimulation.35,66–69 The test is performed by measuring serum cortisol concentrations immediately before and 30 minutes after IV injection of ACTH in a dose of 1 µg (160 mIU) per 1.73 m2 body surface.34 This dose stimulates maximal adrenocortical secretion up to 30 minutes post injection, and in normal subjects results in a peak plasma ACTH concentration about twice that of insulin-induced hypoglycemia.70 A value of 18 µg/dL (500 nmol/L) or more at any time during the test is indicative of normal adrenal function. The advantage of this test is that it can detect partial adrenal insufficiency that may be missed by the standard high-dose test.57,58
Using the 1-µg ACTH stimulation test to more precisely uncover patients with RAI in septic shock has been proposed, but the 1-µg stimulation test has not been well validated in critically ill patients and patients with septic shock.34,35 In addition, studies evaluating low-dose and high-dose ACTH stimulation tests in septic shock may have been flawed by methodological problems. At present, using the 1-µg ACTH stimulation test cannot be recommended routinely until further data from well-designed randomized studies in septic shock patients are available. The current recommendation is to use a three-level therapeutic guide for evaluating RAI in critically ill patients, especially those with septic shock. Patients with a random basal cortisol below 15 µg/dL will likely profit from low-dose corticosteroid therapy, whereas corticosteroid replacement is unlikely to be helpful when basal cortisol is above 34 µg/dL. When a random basal cortisol value is between 15 and 34 µg/dL, adrenocortical stimulation with 250 µg ACTH should discriminate responders (incremental increase ≥ 9 µg/dL) from nonresponders (<9 µg/dL). However, it has been pointed out that no cutoff values will be entirely reliable.35
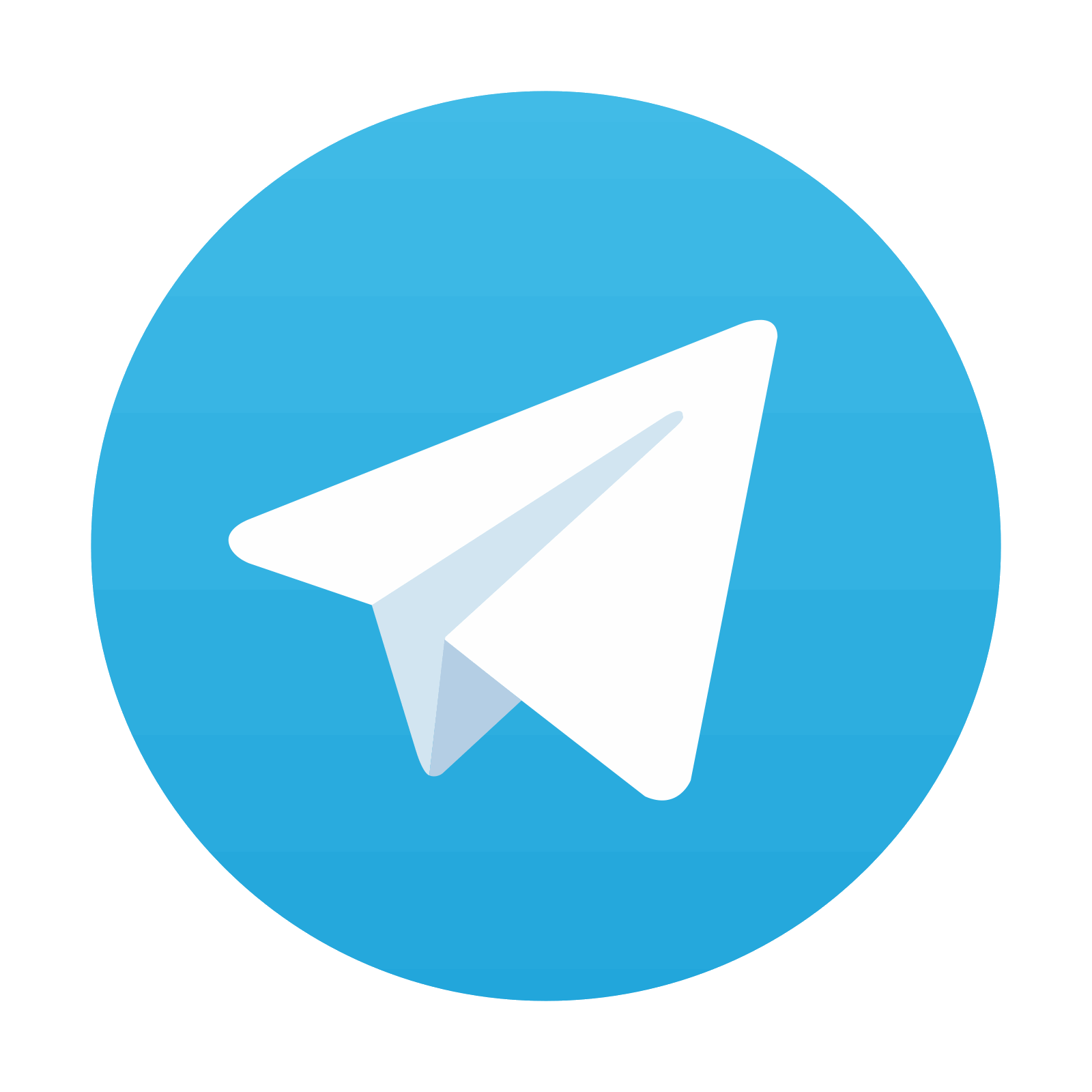