Cytokine
Action
IL-1β (beta)
Increased vascular permeability and apoptosis of epithelial cells
IL-8
Increased neutrophil aggregation and activation
TNF-α (alpha)
Induction of inflammation, cachexia, and increased transcription of cytokines
TGF-β (beta)
Increased transcription of pro-inflammatory cytokines and integrins
TXA2
Increased aggregation and activation of platelets
Thrombin
Increased vascular permeability, increased expression of cytokines, increased recruitment of inflammatory cells
The predominant form of IL-1 in humans is IL-1β. Interleukin-1β is released from alveolar macrophages in response to atelectasis and acts locally on endothelial and vascular epithelial cells to promote inflammation, increased vascular permeability, and apoptosis. IL-1β is considered to be the central mediator of inflammatory injury in ALI.
IL-8 is a neutrophil chemoattractant and activating agent that results in neutrophil degranulation, increased respiratory burst activity, and increased neutrophil aggregation by up regulation of adhesion molecules [5]. In response to IL-8 and cytokine induction, neutrophils readily cross into the pulmonary parenchyma during many types of inflammatory states including sepsis, ischemia/reperfusion injury, hemorrhage, and hypovolemic shock. The aggregation and activation of neutrophils within the lung parenchyma is an important contributor to the development of ALI. The necessity of neutrophil activation to the development of ALI has been demonstrated by experiments in which neutrophils are eliminated before injury occurs [6]. It is further demonstrated by the attenuation of the severity of ALI in neutropenic patients as compared to patients with normal leukocytes. Acute neutrophilic alveolitis is defined as an increased concentration of neutrophils in the alveolar fluid leading to inflammation and increased leakage of fluid between epithelial tight junctions [6]. Neutrophils also release proteases into the alveolar fluid that in normal wound healing function in cell growth, remodeling, and repair; however, in ALI excessive activity can result in cell death and tissue injury. One of these proteases, neutrophil elastase, degrades collagen and elastin resulting in the breakdown of the endothelial vascular barrier.
TNF-α is released from both macrophages and endothelial cells of the lung in response to bacterial endotoxin and IL-1. Its systemic effects include the induction of fever and up regulation of catabolic processes resulting in cachexia. The primary action of TNF-α in ALI is the regulation of immune cells and induction of inflammation through the increased transcription and release of other inflammatory mediators such as IL-1 and nuclear factor-kappa B (NF-κB [kappa beta]). NF-κB is a transcription factor that acts on enhancer/promoter regions that are located on genes known to produce cytokines as well as other immunoregulatory molecules [6].
TGF-β is generated in response to tissue injury. Of its three isoforms, it is TGF-β1 that is present in its inactive form in endothelial cells and contributes to the progression to fibrosis in ALI. The activation of TGF-β signaling pathways results in induction of many genes including those that contribute to fibrosis after lung injury, increased endothelial cell permeability, and decreased ion and fluid transport contributing to increased pulmonary edema [7, 8]. All cells in the body have receptors for TGF-β and activation of these receptors results in a signaling pathway that modulates the transcription of genes for pro-inflammatory factors such as TNF-α and IL-1 by a protein kinase-linked mechanism. Other functions of TGF-β include the increased transcription and expression of integrins that result in increased transmigration of fibroblasts, macrophages, and neutrophils into sites of inflammation [8].
Platelets play a key role in inflammation associated with ALI. Neutrophil activation results in the sequestration of platelets within the lungs. These activated platelets release thromboxane A2, which is a pro-inflammatory cytokine. Thromboxane A2 is a derivative of arachidonic acid through the action of cyclooxygenase that contributes to thrombosis of the microvasculature by increasing the activation and aggregation of platelets. Activated platelets also release prostaglandins, prostacyclins, and leukotrienes—all of which play a role in acute inflammation and increased vascular permeability.
Thrombin is not only a pro-coagulant factor but also acts to propagate lung injury in ALI by regulation of cellular contraction, thus increasing vascular permeability, increasing the expression of inflammatory mediators, and increasing chemotaxis and transendothelial migration of neutrophils and other inflammatory cells [9].
Non-cardiogenic Pulmonary Edema and Endothelial Layer Disruption
Development of pulmonary edema results from changes in oncotic pressure across the endothelial–epithelial membrane. An increase in the leakage of proteins into the interstitial and alveolar space results in fluid shifts and the accumulation of exudative edema fluid within the airways. The usual barrier function is maintained by a single layer of vascular endothelial cells that are joined together by proteins called adherens that form tight junctions between cells. This single cell layer forms the basis for gas exchange as well as regulates permeability to water and electrolytes. Inflammatory mediators cause dysfunction in this barrier by disrupting adherens.
Calcium diffusion across the cell membrane and through disrupted tight junctions results in propagation of inflammatory signals across gap junctions and also functions in the activation of myosin light-chain kinase (MLCK). Increased expression of MLCK contributes to the rearrangement of actin and increased actin–myosin interactions that result in contraction of cells, increased permeability to proteins and fluids, and increased transmigration of neutrophils into the alveoli. MLCK knockout models result in decreased pulmonary edema and reduced nitric oxide, reactive oxygen species, and vascular permeability implicating an interaction between MLCK and paracrine signaling pathways in ALI [10].
Nitric oxide release from endothelial cells and neutrophils is also increased in response to inflammation. Nitric oxide is a potent vasodilator and at high concentrations is toxic to endothelial cells. Nitric oxide synthase expression is increased in response to pulmonary inflammation and sepsis [11].
Apoptosis
Endothelial cell dysfunction alone does not result in pulmonary edema, but a combination of endothelial dysfunction with disruption of the epithelial cell layer results in massive pulmonary edema characteristic of ARDS and life-threatening hypoxemia. The epithelial layer provides a substantial barrier to the accumulation of fluid within the airspace and also functions in the active clearance of fluid by the up regulation of Na/K-ATPase. In the acute inflammatory phase several factors favor apoptosis of epithelial cells including decreased surfactant, increased TGF-β, and increased activity of angiotensin-converting enzyme leading to increased production of angiotensin II.
Normally there is a balance between pro-apoptotic and anti-apoptotic factors within the lungs. Fas and fas ligand (FasL) are regulators of apoptosis in epithelial cells. Increases in the expression of Fas have been demonstrated in experimental models of lung inflammation secondary to exposure to endotoxin. Increases in the amount of FasL in the BAL fluid of patients with ALI are associated with a poor prognosis [12] and survivors of ALI have been demonstrated to have significantly lower levels of FasL in BAL fluid samples compared to non-survivors [13]. Caspase-3 is an executioner protein that is upregulated in Fas-mediated apoptosis [13]. Jo2 is a potentiator of Fas that favors epithelial cell apoptosis, neutrophilic inflammation, and increased permeability in experimental models of ALI [12].
The over-expression of Her 2, a tyrosine kinase receptor expressed in pulmonary epithelial cells, has also been shown to play an active role in epithelial cell barrier disruption. Activation of Her 2 through interactions with IL-1 by A disintegrin and metalloproteinase 17 (ADAM 17) and neuroregulin-1-dependent mechanism during inflammation results in barrier disintegration in an experimental model of ALI [14].
Injury to the epithelial barrier has three direct consequences: (1) it contributes to alveolar fluid accumulation through loss of barrier function; (2) the damage to type II pneumocytes leads to loss of adequate surfactant production; (3) and disorganized repair leads to fibrosis and scarring [8]. Loss of the epithelial layer through apoptosis results in edema, hemorrhage, and increase in proteinaceous fluid accumulation within the remaining alveoli.
Transfusion-Related Acute Lung Injury
Although the clinical presentation of TRALI is nearly identical to other forms of ALI there are a few key differences in the pathophysiology. Patient-related risk factors for TRALI include recent surgery, active infections, and hematologic malignancies. A two-hit theory of inflammatory response to transfusion has been proposed as a mechanism for TRALI [15]. The first event involves priming of neutrophils to induce ALI in response to the major histocompatability complex (MHC) class I antibody by bacterial endotoxin, sepsis, or even stress of surgery. The second hit develops in response to anti-leukocyte antibodies contained within the blood products with resultant activation of neutrophils and aggregation within the lung parenchyma in a manner similar to other forms of ALI. This theory is supported by the fact that donor-related risk factors include plasma from donors exposed to class I antibodies, including plasma from female donors who may have been exposed to antibodies during pregnancy. For this reason most blood banking centers restrict plasma donations to male donors and as a result, the incidence of TRALI has significantly decreased.
Diagnosis
The diagnosis of ALI is made based on the consensus criteria established by the American-European Consensus Committee shown in Table 8.2. Additionally, for TRALI, the patient must develop ALI within 6 hours of receiving blood products.
Table 8.2
Diagnostic criteria for ARDS
American-European consensus committee diagnostic criteria for ALI and ARDS |
---|
PaO2/FiO2 <300 (ALI) or <200 (ARDS) |
Absence of cardiogenic pulmonary edema |
Diffuse bilateral patchy infiltrates on chest radiograph |
Experimentally, researchers have utilized positron emission tomography (PET) in the diagnosis of ALI. PET scanning has been shown to be useful in delineation of several of the pathophysiologic characteristics of ALI and can be used to elucidate the heterogeneity of diseased lung parenchyma. Currently there are three areas of pathophysiologic interest that have been elucidated using PET scanning: the aggregation of activated neutrophils within the diseased lung parenchyma, fluid movement and vascular permeability, and ventilation and perfusion. Because the metabolic activity of neutrophils can be measured using fluorodeoxyglucose (18F) [16], PET scanning can be used to localize aggregates of activated neutrophils within the lung parenchyma in patients with ALI. Fluid movement and vascular permeability can be measured using radiolabeled transferrin. Finally, ventilation and perfusion can be measured using radiolabeled nitrogen (inspired and intravenous, respectively) [17]. Although it is of significant interest to investigational researchers, PET scanning is not currently utilized clinically.
Management
When managing ALI, it is important to have a systematic approach with the goal of improving oxygenation and pulmonary compliance while diminishing morbidity and ultimately improving survivability. The management of ALI in surgical patients begins with the prevention of the causative factors that contribute to its development, most notably atelectasis and systemic inflammatory response syndrome (SIRS).
Basic Principles
As noted previously, atelectasis is a common problem in surgical patients due to the compounding effects of preoperative pulmonary conditions and postoperative pain. Thus adequate management of postoperative pain is of paramount importance, as is maintaining adequate lung volumes for oxygenation but without inducing barotrauma. Appropriate pain control includes early identification of patients who may benefit from epidural analgesia, appropriate usage of patient-controlled analgesia, and early transition to oral analgesia to provide steady-state pain control. Other conditions that can limit chest wall excursion and exacerbate atelectasis include abdominal compartment syndrome, rib fractures, and burns to the thorax. Appropriate intervention would include early decompressive laparotomy, rib fracture plating, and escharotomy as indicated. Prevention of atelectasis in surgical patients can also be accomplished through appropriate training in incentive spirometry (IS) and early involvement of the respiratory therapist for patients determined to be at high risk based on the type of surgery and preoperative pulmonary comorbidities. Respiratory therapists can provide additional recruitment maneuvers to awake, spontaneously breathing patients that result in improvement in the functional residual capacity (FRC) with improved oxygenation and ventilation. These maneuvers serve as an adjunct to IS or as a replacement in patients unable to adequately perform IS due to cognitive or physical limitations. Intermittent positive pressure breathing (IPPB) with systems including the Acapella valve (Smith Medical, St. Paul, MN) and EZPAP (Smith Medical, St. Paul, MN) makes IPPB easier for patients to perform and tolerate. These devices each contains an inspiratory valve and expiratory resistance dial and results in hyperinflation of the lungs, thus increasing lung capacity. Respiratory therapists can also provide chest physiotherapy with the goals of improving respiratory efficiency, expansion of the lungs, strengthening of the respiratory muscles, and elimination of secretions.
Trauma and sepsis are the most common causes of SIRS in the acute care surgery patient. Early goal-directed therapy for sepsis is paramount in preventing ARDS. Risk factors for the development of ALI in patients with sepsis due to infectious extra-pulmonary processes are delayed goal-directed resuscitation and delayed antibiotic treatment. Appropriate identification of the etiology of sepsis and therapy tailored to provide adequate source control is of utmost importance. Identification of patients presenting with clinical signs and symptoms of systemic inflammation and appropriate initiation of treatment at the earliest time point has been associated with improved outcomes. The definition of SIRS is outlined in Table 8.3. The treatment of SIRS is directed at the inciting cause as well as appropriate supportive care that may include broad-spectrum antibiotics, appropriate fluid resuscitation, and close cardiac monitoring as indicated whether by invasive means (pulmonary artery catheterization) or noninvasive means (cardiac and inferior vena cava ultrasound, impedance…), as well as early, appropriate source control.
Table 8.3
Systemic inflammatory response syndrome (SIRS)
Diagnosis of the systemic inflammatory response syndrome |
---|
Any two of the following: |
Temperature <36 °C or >38 °C |
Heart rate >90 bpm Respiratory rate >20 bpm WBC <4,000/mm3 or <12,000/mm3 |
Or >10% bands |
The prevention of MOF is the main indicator of survivability in ARDS. Numerous studies have concluded that death in ARDS is directly attributable to MOF rather than refractory hypoxemia, and that improvement in oxygenation does not necessarily predict a better outcome. A large international study determined that disease severity and MOF were the strongest independent predictors of death from ARDS [18].
In patients presenting with mild hypoxemia and risk factors for ALI, appropriate administration of noninvasive ventilatory support is indicated. This can include continuous positive airway pressure (CPAP) and bilevel positive airway pressure (BiPAP) as appropriate. However careful monitoring of patients undergoing these interventions is important as rapidly progressive and treatment-refractory hypoxemia is most often imminent. These interventions may also be contraindicated in patients with recent upper gastrointestinal anastomoses if nasogastric decompression cannot be adequately maintained.
Standard Mechanical Ventilation Using a Lung Protective Strategy
Paramount to the treatment of ALI and ARDS is support with mechanical ventilation. Although invasive mechanical ventilation is often necessary for adequate oxygenation in cases of ALI and ARDS, it can exacerbate pulmonary parenchymal injury and complicate the care of patients. Shear forces associated with high-lung-volume ventilation cause damage to alveolar epithelial cell membranes leading to disruption and necrosis. Spillage of intracellular contents into the extracellular milieu potentiates the influx of inflammatory cells and cytokines in the pulmonary parenchyma. This repeated over-distention of the alveolar units and shearing injury due to volutrauma and barotrauma is known as ventilator-induced lung injury (VILI), which can exacerbate both ALI and MOF by increasing the release and dissemination of inflammatory mediators within the body and may increase translocation of bacteria from the lungs into the systemic circulation. The prevention of VILI has become the cornerstone of what is known as lung-protective ventilation for patients with ALI (Table 8.4). Lung-protective ventilation as described by the ARDS Net lung protective strategy [19] is defined by low tidal volumes, limited inspiratory plateau and peak pressures, and adequate PEEP to prevent cyclic opening and closing of lung units, thus ameliorating lung stretch and resultant shear injury. PEEP and FiO2 should be adjusted to keep the PaO2 between 50 and 80 mmHg. The fraction of inspired oxygen should ultimately be kept below 60% to prevent absorption atelectasis and oxygen toxicity with resultant free radical injury.
Table 8.4
ARDS network lung-protective ventilation
ARDS network lung-protective ventilation criteria |
---|
Tidal volume 6 ml/kg |
FiO2 <60 mmHg |
PEEP at an adequate level to keep PaO2 between 50 and 80 mmHg |
Inspiratory plateau pressures <30 mmHg |
Low Tidal Volume
The reduction in tidal volumes for patients on mechanical ventilation in order to prevent complications from ARDS was initially resisted by many clinicians. This was due to concern for patient comfort (compensatory tachypnea), as well as the resultant hypercapnia and respiratory acidosis. The ARDS Net trial definitively demonstrated the morbidity- and mortality-reducing benefits of a low-tidal-volume ventilatory strategy. This was a multicenter randomized controlled trial examining the outcomes of 6 ml/kg tidal volumes versus 12 ml/kg tidal volumes. Overall, patients treated with the lower tidal volume strategy had decreased mortality, increased ventilator free days, and a decreased rate of MOF than their cohorts treated with the higher tidal volume. The reduction in tidal volume also seems to be associated with improvement in the function of the sodium/potassium adenosine triphosphate (Na/K ATPase) transporter [20]. This results in improved Na and water transport across the epithelial–endothelial cell junction and out of the pulmonary parenchyma, resulting in a reduction in pulmonary edema.
Limited Inspiratory Peak and Plateau Pressures
Mechanical ventilation can be based on either volume or pressure controlled parameters. For both strategies, the maintenance of plateau pressures below lung injury thresholds is important for the prevention of barotrauma. The upper limit of inspiratory plateau pressures should be maintained below 30 mmHg. Complications of barotrauma include migration of air into the extra-pulmonary space leading to pneumothorax, pnuemomediastinum, pneumoperitoneum, subcutaneous emphysema, and air embolism [21].
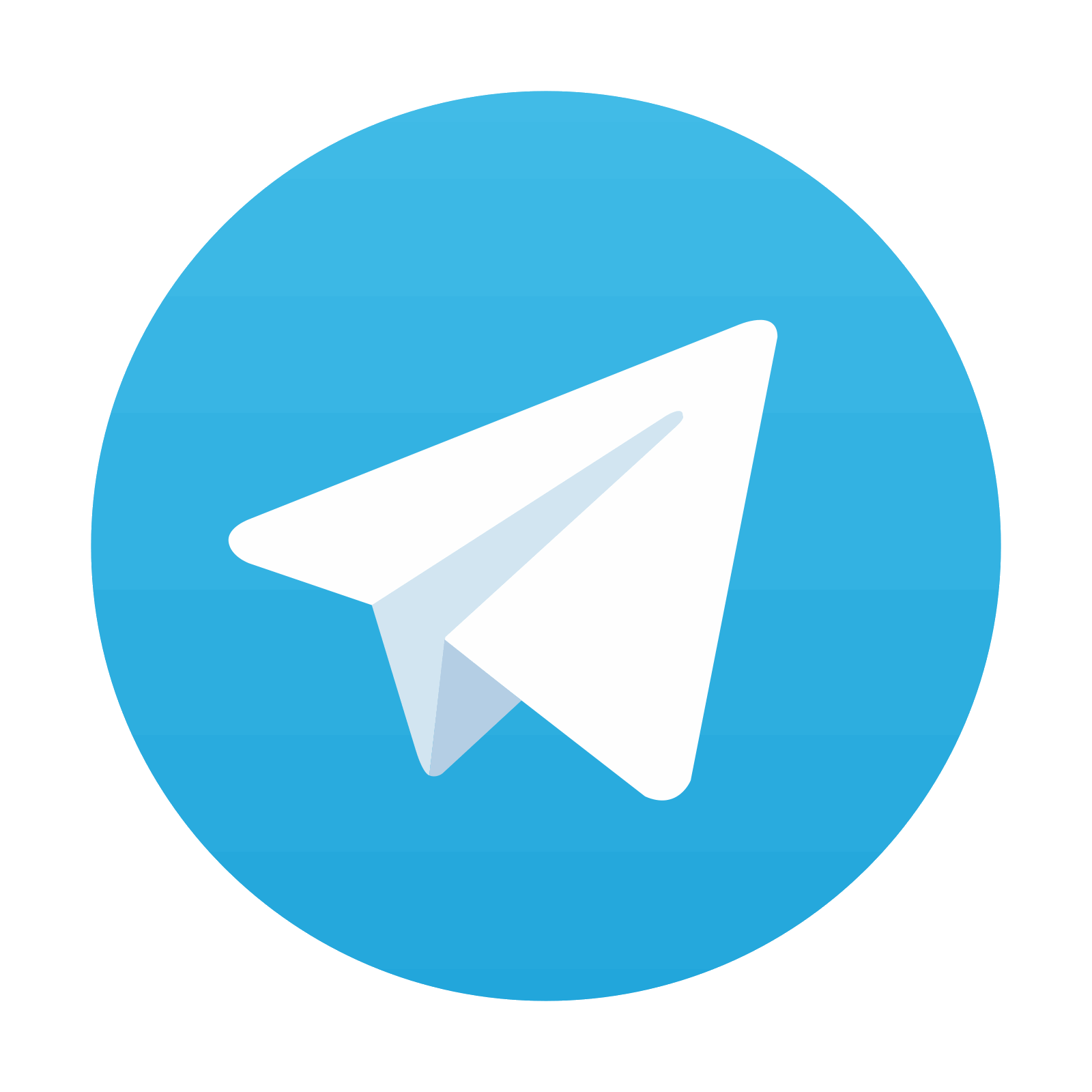
Stay updated, free articles. Join our Telegram channel
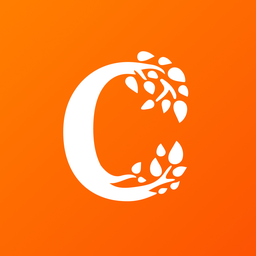
Full access? Get Clinical Tree
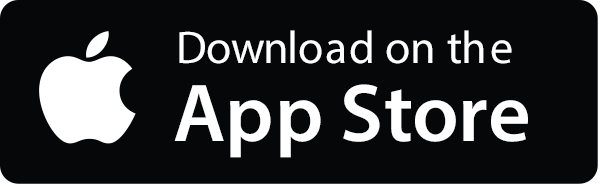
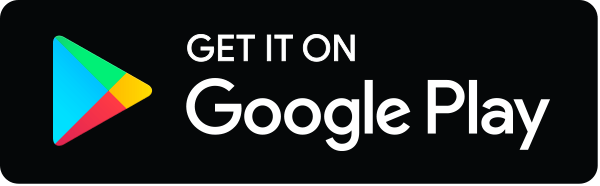