55 The primary function of the kidneys is the excretion of metabolic waste products and the maintenance of the composition of body fluids through regulation of water, electrolyte, and acid-base excretion. Acute kidney injury (AKI) describes a sudden decrease in glomerular filtration rate (GFR), resulting in the retention of metabolic waste products and dysregulation of fluid, electrolyte, and acid-base homeostasis.1–3 AKI, however, does not represent a discrete disease. Rather, AKI is a heterogeneous spectrum that includes hemodynamic perturbations that disrupt normal renal perfusion and decrease GFR without causing overt parenchymal injury; partial or complete obstruction to urine flow; and acute parenchymal injury resulting in glomerular, interstitial, tubular, or vascular dysfunction. Although all forms of AKI can occur in those who are critically ill, the most common causes in this population include hemodynamically mediated prerenal dysfunction and acute tubular necrosis (ATN) arising as a result of ischemia-reperfusion injury, nephrotoxin exposure, or sepsis. The term acute kidney injury has largely replaced the older terminology of acute renal failure (ARF).3–6 Implicit in this older terminology was a focus on the most severe presentations of acute kidney dysfunction, generally characterized by overt organ failure. The term AKI attempts to broaden the focus to include less severe episodes that, although not resulting in overt organ failure, are associated with increased risks of morbidity and mortality, particularly in the critically ill. However, the use of the term injury may incorrectly connote the presence of parenchymal organ damage, which may be absent in a number of forms of AKI, particularly in hemodynamically mediated prerenal states and acute obstructive uropathy. In this chapter, the term AKI will be used to describe the entire spectrum of the syndrome, and the term ARF will be restricted to severe organ failure requiring renal replacement therapy. Often, the terms ATN and AKI have been used interchangeably; although ATN is the most common form of intrinsic AKI, particularly in critically ill patients, the terms are not synonymous, because ATN represents only one of the multiple forms of AKI. The cardinal manifestation of AKI is the retention of metabolic waste products, most notably creatinine and urea.1,2 Decreased urine output may also be a manifestation of AKI; however, the urine output in AKI can be highly variable, ranging from virtual anuria (<100 mL/day) to polyuria (>3 L/day).1 In addition, transient oliguria (urine volume <400 mL/day) may occur in the absence of decreased kidney function in patients with intravascular volume depletion as the physiologic response to volume depletion due to an increase in tubular salt in water reabsorption.7 In contrast, persistent oliguria despite adequate volume resuscitation is virtually always a manifestation of AKI.7 Although the presence of oliguria complicates management, increasing the risk of volume overload, hyperkalemia, and other electrolyte disturbances, and is associated with a greater mortality risk, therapeutic interventions to augment urine output have not been shown to improve outcomes. A wide array of operational definitions have been used to define AKI based primarily on relative or absolute changes in serum creatinine concentration.14 The first attempt at developing a consensus definition was undertaken by the Acute Dialysis Quality Initiative in 200215; the resultant RIFLE criteria consisted of three strata (“Risk,” “Injury,” and “Failure”) based on the magnitude of increase in serum creatinine and the duration of oliguria as well as two outcome stages (“Loss” of kidney function and “End-Stage” kidney disease) (Table 55.1). In the RIFLE criteria, the “Risk,” “Injury,” and “Failure” strata were defined based on an increase in serum creatinine developing over 7 days of 50%, 100%, or 200%, respectively, relative to baseline or oliguria, defined as a urine output of less than 0.5 mL/kg/hour for more than 6 hours (“Risk”) or 12 hours (“Injury”) or a urine output of less than 0.3 mL/kg/hour for more than 24 hours or anuria for more than 12 hours (“Failure”). These three strata were proposed as providing increasing specificity, although decreasing sensitivity, for the diagnosis of AKI. The outcome criteria were defined based on continued need for renal replacement therapy for more than 4 weeks (“Loss”) or more than 3 months (“Failure”). The RIFLE criteria were subsequently modified by the Acute Kidney Injury Network (AKIN) with the addition of an absolute increase in serum creatinine of more than 0.3 mg/dL to the definition of AKI, a decrease in the time frame for the increase in serum creatinine from 7 days to no more than 48 hours, and by dropping the two outcome criteria.4,5 More recently, the Kidney Disease Improving Global Outcomes (KDIGO) Clinical Practice Guideline for Acute Kidney Injury harmonized the RIFLE and AKIN definitions, maintaining the three stages, but specifying that the 0.3-mg/dL increase in serum creatinine was to have developed over no more than 48 hours but that the greater than 50% increase could occur over up to 7 days (see Table 55.1).3 Estimates of the incidence of AKI are highly dependent on both the precise definition employed and the characteristics of the patient population studied. Many of the epidemiologic studies that have been published either preceded the development of the standardized definitions just described16–24 or have utilized administrative data that do not correlate with these definitions.25–28 In addition, there are important differences in the distribution of the different types of AKI among patients who develop AKI prior to hospitalization, on general medical-surgical wards, or in the intensive care unit. As a result, published estimates of the incidence of AKI in hospitalized patients have ranged from as high as 44%, using a 0.3-mg/dL change in serum creatinine as the definition to less than 1% when the definition was based on an increase is serum creatinine of more than 2 mg/L, with most estimates of the incidence of AKI ranging from 3% to 7% of the overall hospital population and 10% to 35% of critically ill patients, and 5% to 6% of the ICU (intensive care unit) population having AKI severe enough to require renal replacement therapy.16–24 The incidence of AKI has increased substantially over the past several decades. In an analysis of data from the National Hospital Discharge Survey in the United States conducted by the Centers for Disease Control and Prevention, the incidence of AKI among hospitalized patients increased from 18 per 100,000 population in 1980 to 365 per 100,000 population in 2005 with similar trends present in analyses of data from the U.S. National Inpatient Sample and a 5% sample of hospitalized Medicare beneficiaries.28 These data need to be interpreted with some caution; however, administrative coding for AKI is incomplete, capturing only 20% to 30% of all episodes, and trends over time may be subject to bias from changes in administrative coding practices.29 However, similar trends were observed in an analysis of administrative and clinical data from Kaiser Permanente of Northern California, an integrated health care delivery system.30 Using laboratory creatinine data to confirm the diagnosis of AKI, the incidence of AKI not requiring renal replacement therapy increased from 323 to 522 cases per 100,000 person-years between 1996 and 2003; over the same time period, the incidence of AKI requiring renal replacement therapy increased from 19.5 to 29.5 cases per 100,000 person-years. Epidemiologic studies have consistently found that AKI is more prevalent in men and in African Americans and increases in incidence with increasing age.25,26 Increasing risk of AKI is also associated with severity of baseline chronic kidney disease (CKD). In the aforementioned study from Kaiser Permanente of Northern California, the risk of developing AKI requiring dialysis was approximately double in patients with a baseline estimated GFR between 45 and 60 mL/minute/1.73 m2 as compared to patients with an estimated GFR greater than 60 mL/minute/1.73 m2, with the risk increasing to more than 40-fold among patients with a premorbid estimated GFR less than 15 mL/minute/1.73 m2.31 Other identified independent risk factors for the development of AKI include diabetes mellitus, hypertension, and the presence of proteinuria.31,32 Although AKI is common in critically ill patients, robust epidemiologic data are lacking as the majority of published studies represent the experience at single centers or limited numbers of ICUs.19–23,33,34 In addition to varying criteria used to define AKI, the incidence of AKI is also highly dependent upon the characteristics of the ICU population. As shown in Figure 55.1, the incidence of AKI is lower in the cardiac care unit and in patients after coronary artery bypass grafting, being 5% to 10%,35,36 as compared to patients with severe trauma,37 burns,38 and following liver and allogeneic bone marrow transplant, in whom AKI occurs in 20% to 40%.39 The patterns of AKI in the ICU differ from those seen in the general hospital population (Table 55.2). Patients with ICU-associated AKI are younger, are more likely to be male, are more likely to have acute tubular necrosis as compared to prerenal AKI or obstructive disease, are more likely to have AKI in association with multisystem organ failure as opposed to isolated AKI, are more likely to require renal replacement therapy, and have markedly higher mortality rates.19 Sepsis is the most common predisposing condition for the development of AKI in critically ill patients, contributing to the development of AKI in as many as half of all cases.19,21,22,24 Other conditions associated with the development of AKI in ICU patients include major surgery, cardiogenic shock, hypovolemia, medication-associated toxicity, and advanced liver disease (Table 55.3).19,21,22,24 Two distinct patterns of ICU-associated AKI have been described: early-onset AKI is present on ICU admission or within the first 48 hours of ICU stay, and late-onset AKI develops after more than 48 hours of ICU care.23 Delayed-onset AKI is more likely to be due to postischemic or sepsis-associated AKI and is associated with higher mortality rates. Table 55.2 Comparison of Intensive Care Unit (ICU)– and General Hospital Ward–Associated Acute Kidney Injury From Liano F, Junco E, Pascual J, et al. The spectrum of acute renal failure in the intensive care unit compared with that seen in other settings. Kidney Int 1998;53(Suppl 66):S16-S24. Table 55.3 Factors Associated with Acute Kidney Injury in the Intensive Care Unit Setting AKI can be divided into three broad etiologic categories: prerenal AKI, intrinsic AKI, and postrenal (obstructive AKI). Prerenal states (Box 55.1) are characterized by effective hypoperfusion of the kidneys leading to a decrease in GFR in the absence of overt parenchymal damage to the kidneys. Postrenal or obstructive AKI is characterized by acute obstruction of the urinary tract, including ureters or bladder outlet (Box 55.2). In intrinsic AKI, acute injury to the renal parenchyma underlies the development of kidney dysfunction, as is seen in acute tubular necrosis (ATN), acute interstitial nephritis (AIN), and acute glomerulonephritis (AGN) (Box 55.3). Categorizing the forms of AKI in this fashion is useful for didactic purposes and is helpful in guiding the initial assessment of the patient with AKI, but substantial overlap may exist among these categories, particularly between prerenal and intrinsic causes of AKI. For example, renal hypoperfusion may cause a spectrum of renal dysfunction ranging from mild prerenal azotemia to overt ATN, depending upon its severity and duration. Even in patients with classic clinical presentations of volume depletion–associated prerenal AKI sensitive clinical markers of tubular injury may be elevated.40 Thus, precise categorization of the cause of AKI into one of these categories may not be possible, and overlap and transition among categories may occur. Prerenal azotemia caused by extracellular fluid volume loss or volume sequestration, reduced cardiac output, systemic vasodilation, intrarenal vasoconstriction, or increased renal venous pressure is the most common cause of AKI (see Box 55.1), contributing to the development 30% to 60% of all cases.17–19,24 The primary pathogenesis of prerenal azotemia is a decrease in effective glomerular perfusion. This form of AKI, if treated early, is usually readily reversible; however, if left untreated, renal ischemia with resultant ATN may result. In classic forms of prerenal azotemia, reduced renal perfusion pressure and afferent arteriolar constriction combine to lower glomerular capillary hydrostatic pressure and the formation of glomerular ultrafiltrate.1,41,42 Prerenal azotemia develops when the capacity of the usual physiologic responses to hypovolemia is exceeded and GFR falls. In response to hypovolemia there is a decrease in mean arterial pressure, triggering baroreceptors that ultimately lead to activation of the sympathetic nervous system, activation of the renin-angiotensin-aldosterone system (RAAS), and secretion of the antidiuretic hormone vasopressin. Activation of the renal sympathetic nerves constricts the afferent (preglomerular) arterioles and stimulates release of renin from the juxtaglomerular apparatus. Renin secretion is also directly stimulated in response to hypovolemia by changes in intrarenal hemodynamics. Secretion of renin activates a cascade in which angiotensinogen is cleaved to form angiotensin I, which is then cleaved by angiotensin-converting enzyme released by local endothelium to form angiotensin II. Angiotensin II stimulates both afferent and efferent (postglomerular) arteriolar vasoconstriction; however, the effect on the afferent vessel is opposed by vasodilatory prostaglandins, kallikrein, kinins, and nitric oxide.43,44 The net effect is to maintain the tone of the afferent arteriole while constricting the efferent vessel, returning intraglomerular pressure and glomerular ultrafiltration toward normal. In addition, angiotensin II stimulates proximal tubular sodium reabsorption and, through its action as an aldosterone secretagogue, increases plasma aldosterone levels and stimulates distal tubular sodium reabsorption. Increased vasopressin levels stimulate water and urea reabsorption in the collecting duct. The net effect of modest hypovolemia is maintenance of the GFR at near normal levels accompanied by production of concentrated urine with low sodium content and a decrease in the fractional excretion of urea. When effective volume depletion exceeds the normal physiologic compensatory mechanisms, the GFR falls and overt prerenal AKI ensues. Multiple medications can exacerbate the development of prerenal AKI. The prerenal form of AKI complicating diuretic use is caused by extracellular volume depletion. With nonsteroidal anti-inflammatory drugs (NSAIDs), including both nonselective and cyclooxygenase 2 (COX-2)–selective agents, the inhibition of cyclooxygenase leads to depletion of renal vasodilatory prostaglandins that normally counteract the afferent arteriolar constricting effect of angiotensin II and increased renal adrenergic tone.45–47 In situations in which vasoconstrictor mechanisms are activated, such as volume depletion, heart failure, sepsis, cirrhosis, and nephrotic syndrome, and in patients with chronic kidney disease, the use of NSAIDs may result in severe afferent vasoconstriction, severely reducing glomerular capillary filtration pressure and causing AKI. If promptly discontinued, NSAID-induced AKI can be reversible; however, if NSAIDs continue to be administered ATN may ensue. Angiotensin-converting enzyme inhibitors (ACEIs), angiotensin receptor blockers (ARBs), and direct renin inhibitors act by inhibiting the RAAS. Inhibition of angiotensin II production by direct renin inhibitors and ACEIs or blockade of its action by ARBs prevents angiotensin II–mediated constriction of the efferent arteriole and diminishes glomerular capillary filtration pressure. Although this effect is desirable in preventing hyperfiltration injury in patients with CKD, the use of these agents may contribute to the development of AKI in the setting of renal hypoperfusion, when maintenance of glomerular filtration is dependent upon activation of renin and angiotensin II.48 This is particularly likely to happen when therapy is initiated or escalated and volume depletion or hypotension supervenes in patients with renal artery stenosis, CKD, heart failure, or liver disease. The prerenal azotemia that may develop in these settings is usually reversible if the offending agent is promptly discontinued; however, these agents have also been associated with an increased risk of ATN.49 Although the majority of forms of prerenal AKI are mediated by decreased renal perfusion, altered intrarenal hemodynamics may result in decreased GFR despite increased organ perfusion. Early in sepsis, renal perfusion may be increased; however, GFR may fall as the result of decreased efferent arteriolar tone, resulting in decreased glomerular capillary filtration pressure.50 Increases in renal venous pressure may also contribute to the development of prerenal AKI. As renal venous pressure increases, renal perfusion pressure falls; in addition, renal parenchymal pressure increases as venous pressure rises, narrowing the pressure gradient driving glomerular filtration.51,52 Two settings in which renal venous congestion results in a prerenal state are the abdominal compartment syndrome and heart failure. Abdominal compartment syndrome (ACS) typically develops in critically ill patients, most commonly in the setting of trauma with abdominal hemorrhage, abdominal surgery, massive fluid resuscitation, liver transplantation, and gastrointestinal conditions including peritonitis and pancreatitis. ACS is defined by an intra-abdominal pressure of 20 mm Hg or higher associated with dysfunction of one or more organ systems.53 However, intra-abdominal pressures lower than 20 mm Hg may be associated with ACS, while values higher than this threshold do not universally lead to the ACS.54–57 Oliguria, which can lead to anuria, often develops and, as is true for other forms of AKI associated with impaired renal perfusion, urine sodium concentration is commonly reduced. Renal venous congestion associated with modest increases in intra-abdominal pressure has also been shown to contribute to the acute renal dysfunction accompanying acute decompensated heart failure.58,59 AKI resulting from obstruction usually accounts for fewer than 5% of ICU-associated AKI.19 Urinary tract obstruction may occur at any level of the renal collecting system, beginning at the renal pelvis and ending at the urethra (see Box 55.2). Obstruction above the level of the bladder is referred to as upper tract obstruction and may result from either intrinsic (intraluminal) or extrinsic disease. The development of AKI from upper tract obstruction requires the presence of bilateral obstruction or unilateral obstruction in the setting of a single functioning kidney or dysfunction of the contralateral kidney. Common causes for upper tract obstruction include luminal obstruction from ureteric calculi, blood clots, urothelial tumors, sloughed renal papillae or ureteral strictures, and external compression from retroperitoneal and pelvic tumors, adenopathy, hematomas, or retroperitoneal fibrosis. More commonly, obstruction occurs at the level of the bladder neck or urethra, as may occur with benign prostatic hypertrophy or prostate cancer, bladder tumors, and bladder stones. Neurogenic bladder dysfunction associated with diabetes mellitus, autonomic neuropathy, and spinal cord disease may also contribute to the development of obstructive disease. Treatment with narcotics and agents with anticholinergic action may exacerbate subclinical voiding dysfunction and precipitate acute urinary retention in the setting of acute illness. Patients with obstructive disease may present with anuria if obstruction is complete, with normal or even increased urine volume in the setting of partial obstruction, or with fluctuating urine output with periods of anuria alternating with rapid passage of urine as the pressure in the collecting system rises and overcomes the obstructing disease. In the acute phase of obstruction, intratubular pressure rises, resulting in a reduction in glomerular filtration pressure. As the obstruction persists, renal blood flow diminishes, intratubular pressures fall toward normal, but GFR remains severely depressed. Relief of the obstruction with placement of a bladder catheter for lower tract obstruction or ureteral stents or percutaneous nephrostomy tubes for upper tract obstruction usually results in prompt return of GFR if the duration of obstruction has not been excessive.60 The causes of intrinsic AKI can be categorized based on the anatomic compartments of the kidney that are involved (see Box 55.3). Using this approach, intrinsic AKI is commonly divided into tubular, interstitial, glomerular, and vascular processes. In addition, in a variety of settings the primary pathophysiology consists of intratubular deposition of crystals or protein. These latter syndromes could be considered as a subset of acute tubular disease, but given the striking difference in pathogenesis, these disorders will be discussed separately in this chapter. It is also important to recognize that although this approach provides a construct for understanding the multitude of causes of intrinsic AKI, there are substantial overlaps among categories. Thus, small vessel vasculitides with predominant glomerular involvement could be classified either with acute glomerulonephritis or as a renal vascular disorder. Similarly, interstitial inflammation may play a substantial role in the pathogenesis of ATN, blurring the distinction with acute interstitial nephritis (AIN). The most common intrinsic cause of AKI is ATN, accounting for 85% to 90% of intrinsic ICU-associated AKI.19,24 The causes of ATN can be broken down into three major categories: ischemia-reperfusion injury, nephrotoxins, and sepsis (see Box 55.3). There is, however, significant overlap between septic and ischemic ATN; many, although not all, cases of sepsis-associated ATN develop in the setting of septic shock, and sepsis-associated ATN has frequently been classified as a subcategory of ischemic ATN.61 Data suggest, however, that sepsis-associated ATN has unique features and may develop in the absence of overt renal ischemia and should be considered as a separate etiologic category.62–64 Nephrotoxic ATN can result from toxicity from the endogenous heme pigments myoglobin and hemoglobin or may be caused by exogenous toxins, most commonly medications such as aminoglycosides, amphotericin B, and cisplatin. In many patients, multiple etiologic factors are present and the ATN must be characterized as multifactorial. A description of the pathophysiologic mechanisms thought to underlie ischemic, septic, and nephrotoxic causes of ATN are provided in the online supplement at www.expertconsult.com. Although the characteristic histologic lesion in ATN is epithelial cell injury with tubular cell necrosis or apoptosis, the pathophysiology of ATN also includes endothelial cell damage and activation of inflammatory pathways. Although all segments of the nephron may be affected, proximal tubular epithelial cells at the corticomedullary junction and outer stripe of the medulla are most susceptible to ischemic injury.65 More proximal tubular segments are involved in toxic nephropathy as a result of their transport and endocytotic pathways that lead to increased cellular uptake of the toxin. Ischemic injury results in depletion of cellular adenosine triphosphate (ATP) in the tubular epithelial cells leading to disruption of the cytoskeleton.66 Maintenance of the cytoskeleton is critical to the functional integrity of the tubular epithelium; its loss leads to disruption of the luminal (apical) brush border membrane and of the adhesion proteins that are critical for maintaining attachment to the basement membrane and for the integrity of the tight junctions between adjacent cells. With their loss, transport proteins such as Na+/K+-ATPase that are normally restricted to the apical or basolateral domains can migrate and normal vectorial transport of sodium and other solutes from tubular lumen to blood is disrupted.67 This may account, in part, for the elevated urine sodium and fractional excretion of sodium often seen in patients with ATN. The loss of attachment to the basement membrane leads to the sloughing of cells into the tubular lumen. Severe ATP depletion may lead to cell necrosis, but less severe injury activates apoptotic pathways, which appear to be the predominant mechanism of cell death in ATN.68 In addition, ATP depletion leads to a rise in intracellular calcium, which serves as a further mediator of cell damage. In addition to epithelial injury, endothelial damage and microvascular dysfunction play a central role in the pathogenesis of ischemic and septic ATN. Endothelial damage results in increased expression of adhesion molecules, leukocyte and platelet adhesion, activation of coagulation pathways, vascular congestion, and obstruction resulting in diminished blood flow through the renal microvasculature.69 The endothelial and epithelial cell injury also leads to recruitment of leukocytes and activation of inflammatory pathways.70,71 The combination of endothelial damage and inflammation may amplify the tubular injury, even after the inciting insult has resolved.69 The reduction in GFR in ATN is the result of several factors.1 Early in ischemic ATN, the combined influence of a decrease in renal perfusion pressure and afferent arteriolar constriction initiates the reduction in glomerular filtration. Later, endothelial damage and microvascular obstruction contribute to the persistence of decreased glomerular filtration. Sloughed debris from necrotic and apoptotic tubular epithelial cells and nonadherent viable cells form obstructing intratubular casts, increasing intratubular hydrostatic pressure and further decreasing glomerular filtration. When these casts pass into the urine they are the “muddy brown” granular casts that are characteristic of ATN. Finally, “back-leak” of filtrate across the denuded tubular basement membrane contributes to the loss of effective GFR. The clinical course of AKI can be conceptualized as consisting of four phases.72 The initial inciting injury is followed by an extension phase during which the endothelial and inflammatory processes result in extension of the tubular injury despite resolution of the triggering insult. The extension phase is followed by a maintenance phase of variable duration during which the GFR remains very low. During this phase, tubular epithelial cells are believed to undergo a process of dedifferentiation and proliferation, repopulating the tubular basement membrane. Finally there is a recovery phase, in which the tubular epithelial cells redifferentiate and reestablish a normal tubular epithelium and GFR recovers. To the extent that repair to the tubular epithelium is complete, kidney function can recover to near normal levels; however, to the extent that fibrotic pathways are activated and there is rarefaction of the capillary bed, recovery of kidney function will be incomplete.73–75 ATN also exhibits distant organ effects. In experimental models, renal ischemia reperfusion injury is associated with alterations in cardiac, pulmonary, hepatic, and brain function.76–78 It is postulated that these distant organ effects are mediated by systemic inflammatory changes, activation of proapoptotic pathways, increases in leukocyte trafficking, and dysregulated channel expression and that these distant effects may contribute to nonrenal organ dysfunction in patients with ATN. The precise mechanisms by which sepsis causes ATN have not been elucidated. In septic shock, renal hypoperfusion likely predominates.61 In less severe sepsis the pathophysiology is thought to include a combination of intrarenal hemodynamic changes; endothelial dysfunction; infiltration of the renal interstitium by lymphocytes, neutrophils, and mononuclear cells; and activation of both pro- and anti-inflammatory mechanisms.62 The precise role of individual mediators in the pro- and anti-inflammatory pathways, including tumor necrosis factor-α interleukin (IL) 1, IL-6, and IL-10, is uncertain. The pathophysiologic process by which nephrotoxins induce renal damage is incompletely understood and most likely differs from agent to agent.46,79 Several of the more commonly encountered nephrotoxins in the ICU setting are aminoglycoside antibiotics, amphotericin B, and iodinated radiocontrast agents. Aminoglycosides generally induce mild (mean rise in serum creatinine of 1 to 3 mg/dL), nonoliguric ATN in 5% to 20% of patients receiving a 7- to 10-day course of therapy.80,81 However, even brief exposure in the setting of impaired renal perfusion can induce some renal damage. Aminoglycosides are avidly taken up by the proximal tubular epithelial brush border via a megalin-dependent mechanism. Once within the cell they are transported to the endoplasmic reticulum and then enter the cytosol. Once in the cytosol, the aminoglycoside disperses to various intracellular organelles, such as mitochondria, where it mediates organelle-specific toxicity.82 Risk factors for aminoglycoside nephrotoxicity include the use of high doses or prolonged courses of therapy, volume depletion, diabetes mellitus, advanced age, baseline CKD and the presence of hypovolemia, renal ischemia, or concomitant administration of other nephrotoxins.81,83 Radiocontrast agents are relatively nontoxic in healthy individuals with an incidence of contrast nephropathy of less than 1%.84,85 Risk factors for the development of contrast-induced AKI include preexistent kidney disease, diabetes mellitus, effective intravascular volume depletion, concomitant therapy with NSAIDs, and exposure to high doses and repeated doses of the contrast agent. The precise mechanism of toxicity is not understood. Administration of radiocontrast agents induces marked renal vasoconstriction, mediated in part by endothelin. The osmotic load and altered flow through the microvasculature due to the high viscosity of the contrast media induces medullary hypoxia. The hypoxia as well as direct effects of the contrast agents induces the generation of reactive oxygen species that may cause membrane and DNA damage. In addition, direct cytotoxicity to renal epithelial cells can be demonstrated. The risk of contrast nephropathy can be mitigated by the use of relatively less toxic low- and isosmolar agents, as compared to the older and more nephrotoxic high-osmolar agents and by administration of intravenous fluids to correct volume depletion and maintain high urine flow rates. Myoglobin and hemoglobin are endogenous heme-containing proteins that are associated with the development of ATN.86,87 Myoglobinuric AKI is much more common than hemoglobinuric disease. Myoglobin is released during muscle injury and is freely filtered by the glomerulus. In contrast, hemoglobin released during intravascular hemolysis is normally bound by haptoglobin; hemoglobinuria will only develop when hemolysis is severe enough to overwhelm the binding capacity of haptoglobin, such as may occur with an ABO-incompatible blood transfusion. Renal injury in pigment nephropathy is due to a combination of factors including volume depletion, particularly in rhabdomyolysis, in which there is sequestration of fluid in the injured muscle, renal vasoconstriction, direct heme-pigment mediated cytotoxicity, and intraluminal cast formation. Despite the toxicity of the heme pigments, AKI is uncommon in the absence of volume depletion, acidosis, and possibly mild ischemia. Prevention of nephropathy in patients with rhabdomyolysis or hemolysis focuses on maintaining adequate extracellular fluid volume and renal perfusion. AIN constitutes approximately 5% to 10% of intrinsic AKI in the ICU setting and is defined by the presence of lymphocytic infiltration of the kidney (see Box 55.3). AIN is most commonly related to medication use but may also be associated with a wide variety of infections, autoimmune disorders, and malignancy.88 The most common offending medications include penicillin, cephalosporin, sulfonamide, and quinolone antibiotics; proton pump inhibitors; phenytoin; allopurinol; and NSAIDs. The classic presentation of medication-associated AIN includes fever, rash, and eosinophilia; however, this complete triad is present in fewer than one third of patients and is unusual in NSAID-associated AIN. Characteristic urinary findings include hematuria and pyuria and white blood cell casts in the absence of infection. Eosinophiluria may also be present but is neither a sensitive nor a specific finding. Although the diagnosis may be made based on clinical setting, history of medication use, and urinary findings, definitive diagnosis usually requires kidney biopsy. Acute vascular syndromes are divided into large vessel (renal artery and renal vein) and small vessel disease. Large vessel diseases include thrombosis, thromboembolism and dissection of the renal arteries, and renal vein thrombosis.89,90 Acute arterial or venous occlusion may present with renal infarction. With unilateral or subtotal disease, the presentation usually consists of pain and hematuria. Lactate dehydrogenase levels will be elevated and the diagnosis made by contrast-enhanced computed tomography (CT) or nuclear scintigraphy. As with obstructive disease, AKI is the presenting manifestation only when there is bilateral involvement or unilateral disease with an absent or nonfunctional contralateral kidney. Multiple conditions can affect the renal microvasculature and present with AKI, including the systemic vasculitides (discussed earlier) that present with primarily glomerular involvement. Other diseases with predominant renal microvascular involvement leading to AKI include malignant hypertension, scleroderma renal crisis, toxemia of pregnancy, and the microangiopathic hemolytic diseases, hemolytic-uremic syndrome, and thrombotic thrombocytopenic purpura. Atheroembolic disease represents another important disease of the renal microvasculature that may present as acute or subacute kidney disease.91,92 Atheroembolic disease is characterized by the systemic showering of atheromatous debris to the distal branches of the arterial tree. Although atheroembolism may occur spontaneously, it develops most commonly after cardiac or aortic angiography or surgery, or in association with systemic anticoagulation or thrombolytic therapy. Involvement is generally systemic with common manifestations including cutaneous livedo reticularis and digital ischemia and gastrointestinal, hepatic, and nervous system involvement. Because the kidneys receive approximately 20% of the cardiac output, renal involvement is common, ranging from renal infarction to AKI to a subacute and stuttering decline in kidney function. AKI may also occur as the result of crystalline or proteinaceous obstruction of the renal tubules. In acute tumor lysis syndrome, AKI often results from intratubular deposition of uric acid.93,94 Following ethylene glycol ingestion and in certain inborn errors of metabolism, the major mechanism for development of AKI is intratubular deposition of calcium oxalate. A number of medications have also been associated with acute crystalline nephropathy, including acyclovir, methotrexate, indinavir, triamterene, and sulfadiazine.95,96 In multiple myeloma, tubular obstruction by monoclonal light chains (Bence-Jones protein) is the cause of acute myeloma kidney disease.97,98 Urea is the major end product of nitrogen metabolism. Blood urea concentration, commonly assayed as blood urea nitrogen (BUN), is dependent upon the balance between hepatic synthesis and renal excretion. Increased urea generation resulting in elevations in the BUN in the absence of a marked decrease in GFR may result from high dietary protein intake, amino acid loading during parenteral nutrition, and the endogenous protein load from gastrointestinal hemorrhage as well as in hypercatabolic states associated with fever, sepsis, and glucocorticoid administration or inhibition of protein synthesis as may be seen with tetracycline antibiotics. Hypercatabolic states may also result in increases in BUN during AKI that exceed the increase of 10 to 20 mg/dL/day that usually is typically associated with absence of glomerular filtration.99 Normally, filtered urea is partially reabsorbed along the length of the nephron, with increased reabsorption in states of low urine flow. In volume depletion, severe heart failure, and obstructive uropathy, increased tubular reabsorption of urea often results in increases in the BUN that are disproportionate to the fall in GFR. This variability in the synthesis of urea and the non-GFR-related factors that influence its urinary excretion render the BUN a less reliable marker of GFR than the serum creatinine concentration. However, the level of BUN generally correlates with symptoms of renal failure, with uremic manifestations usually absent until the BUN is greater than 100 mg/dL. Creatinine is derived from the nonenzymatic hydrolysis of creatine, which is usually released at a constant rate from skeletal muscle and is excreted primarily by filtration at the glomerulus. In patients with normal kidney function, less than 10% of creatinine excretion occurs by tubular secretion, although this percentage increases in CKD. There is essentially no tubular reabsorption of creatinine. This relationship allows creatinine to serve as a reliable endogenous marker of glomerular filtration when the creatinine concentration is in steady state. Because much of the interindividual variability in creatinine production can be accounted for based on demographic and clinical variables including age, gender, race, and weight, it is possible to reliably estimate creatinine clearance or GFR from the serum creatinine concentration.8–10,12 The confidence intervals around these estimates are wide, however, and these estimates should not be interpreted as precise measures of kidney function. In the absence of glomerular filtration, serum creatinine typically increases by 1 to 2 mg/dL/day.100 This increase is influenced by numerous factors including the magnitude of decrement in GFR, the rate of creatinine production, and changes in the volume of distribution.100 For example, creatinine production may be reduced in sepsis,101 and increased in the setting of skeletal muscle injury (rhabdomyolysis). Aggressive volume resuscitation may also mask any increase in serum creatinine through dilution.102 Thus, although a sudden increase in serum creatinine concentration is the most common parameter triggering recognition of AKI, the use of estimating equations for GFR are not reliable in critically ill patients with AKI. There are several other factors that may also impair the reliability of serum creatinine as a marker of kidney function in critical illness. Some medications, most notably trimethoprim and cimetidine, block tubular secretion of creatinine, leading to an increase in serum creatinine concentration in the absence of decreased kidney function.103 This effect is generally minimal in patients with normal kidney function, but the increase in serum creatinine may exceed 30% in patients with underlying CKD. Reported creatinine concentrations may also be increased as a result of chemical interference with some assay methods by ketone bodies or by medications, such as cefoxitin.103 One final drawback to the use of creatinine as a marker of kidney function in patients with AKI is the inverse relationship between serum creatinine concentrations and GFR. Thus, significant reductions in GFR may occur prior to the serum creatinine concentration being recognized as increasing. Given the drawbacks to urea and creatinine as markers of kidney function, other readily available markers of GFR have been sought. The use of exogenous filtration markers such as inulin, iothalamate, or iohexol is cumbersome and not practical for the recognition of abrupt changes in kidney function. Cystatin C has been proposed as a more sensitive endogenous marker of GFR, including in ICU patients.104–106 Cystatin C is a cysteine protease inhibitor that is released into the bloodstream at a constant rate from all nucleated cells. It is readily filtered at the glomerulus and reabsorbed and catabolized by renal proximal tubular epithelial cells such that virtually no cystatin C appears in the urine. The interindividual variability in cystatin C production appears to be less than that for creatinine. Thus, in steady-state situations cystatin C may be a more reliable marker of GFR.107 In addition, the serum half-life of cystatin C is shorter than that of creatinine, making it a more sensitive marker for acute changes in GFR.104–106 However, cystatin C assays are not currently readily available in the acute setting, and the optimal place for cystatin C in the detection of AKI in at-risk critically ill patients remains to be determined. A number of markers of tubular injury have been proposed as novel diagnostic tests for the early diagnosis of AKI. These markers include kidney injury molecule-1 (KIM-1),108,109 neutrophil gelatinase-associated lipocalin (NGAL),110–116 interleukin 18 (IL-18),114–118 liver fatty acid binding protein (L-FABP)40,119 and α- and π-glutathione-S-transferase (GST),120,121 among others. Even though these markers have shown promise, their role in the clinical care of patients at risk for AKI remains uncertain. Decreased urine output represents a second major reason for recognition of AKI. Sustained oliguria, which is usually defined as a urine output of less than 400 to 500 mL/day, or a sustained urine output of less than 20 mL/hour, in the absence of overt volume depletion almost always indicates the presence of AKI.7 Although oliguria is often considered to be a cardinal feature of AKI, most cases of AKI in the critically ill are nonoliguric.122 Thus, although sustained oliguria should suggest prompt the evaluation for AKI, the presence of a well-maintained urine output should not be construed to represent the presence of adequate kidney function. Anuria (the absence of urine output) always demands prompt attention. True anuria is most often caused by complete urinary obstruction but may also be seen with vascular catastrophes with bilateral renal infarction and less commonly with severe rapidly progressive glomerulonephritis. Rarely, severe ATN may result in a short period of complete anuria. The first step in evaluating the patient with AKI is to determine whether it is primarily prerenal or postrenal in origin or is due to intrinsic renal disease. Although this division is useful for conceptualizing and categorizing the causes of AKI, it is important to recognize that many patients may not fall neatly into a single category. For example, prerenal azotemia and ischemic ATN fall on a continuum; a process that may start out as pure prerenal azotemia may evolve over time into ischemic ATN. This blurring between these broad categories is further evidenced by the fact that markers of tubular epithelial cell injury are elevated in patients with classic presentations of prerenal azotemia.40 Moreover, most cases of AKI encountered in ICU patients have more than a single cause,* with the single most common condition predisposing to AKI in the critically ill patient being sepsis and with the majority of patients having AKI in the setting of multiple organ failure.24,61,125 The evaluation of the patient should begin with a careful review of the history and physical examination with particular attention to hemodynamic status, episodes of hypotension and infection, and the record of medication administration. Assessment of voiding function and postvoiding residual bladder volume should be performed to exclude bladder outlet obstruction. This step should then be followed by microscopic examination of the urine and evaluation of urine electrolytes. Renal imaging may be necessary to evaluate for upper tract obstruction or to evaluate for patency of the renal vasculature. In a minority of patients, a renal biopsy will be required for definitive diagnosis. The initial step in attempting to identify the cause of AKI should be a thorough history, physical examination, and review of the medical record. The overall clinical setting, recent events in the patient’s illness, use of medications, and possible toxic exposures should be noted, with particular attention to events during the 1- to 2-day interval prior to the onset of AKI. A history of vomiting, blood loss, diarrhea, diuretic use, burns, or symptoms compatible with decompensation of heart failure or of liver disease suggests potential prerenal azotemia. A history of prostatism with intermittency, hesitancy, or decrease in the force of the urinary stream; history of urologic, gynecologic, or other pelvic or retroperitoneal malignancy; flank or suprapubic pain; or hematuria or pyuria may suggest obstructive (postrenal) disease. The history of a systemic disorder, fever, rash, vascular disease, or musculoskeletal complaints is compatible with a renovascular, glomerular, or interstitial disorder. Review of the medical record should focus on indices of volume status including intake and output records, serial weights, and serial measurements of blood pressure to help assess for risk factors for intravascular volume depletion and prerenal azotemia. Delineation of the pattern of urine output is often helpful. Finally, a careful review of medication records to assess for exposure to potential nephrotoxins is critical.46,79 The physical examination should focus on assessing for evidence of intravascular volume depletion, such as orthostatic changes in pulse and blood pressure, dry mucous membranes, decreased skin turgor, longitudinal tongue furrows, and absence of sweat in the axilla and inguinal regions.126 A fall in systolic blood pressure of more than 20 mm Hg and in diastolic blood pressure of more than 10 mm Hg accompanied by an increase in heart rate of more than 30 beats per minute is suggestive of intravascular volume depletion. However, these findings are neither sensitive nor specific. Orthostatic changes in blood pressure may be absent in some patients despite significant intravascular volume depletion and may be observed in the absence of volume depletion in patients with autonomic dysfunction and in the elderly. Examination for neck vein distention, pulmonary rales, ventricular gallops, and pedal edema may indicate the presence of heart failure. Occasionally, a chest radiograph, cardiac function testing (i.e., an echocardiogram or nuclear gated blood pool scan), or assessment of plasma brain natriuretic peptide (BNP) or N-terminal pro-BNP (NT-proBNP) levels may assist in the diagnosis of heart failure. Accurate assessment of extracellular fluid volume status and cardiac function may be difficult on clinical grounds, particularly in immobilized, mechanically ventilated ICU patients, and invasive monitoring of central venous pressure, or less commonly, pulmonary artery and pulmonary capillary occlusion pressure, and assessment of cardiac output may be helpful. Assessment for ascites and abdominal distention may assist in the diagnosis of AKI associated with liver disease or the abdominal compartment syndrome. Abdominal palpation to detect flank, suprapubic or central abdominal masses may be helpful in assessing the presence of obstructive uropathy or for an abdominal aortic aneurysm with possible renovascular compromise. A bladder scan or placement of a bladder catheter to assess postvoid bladder volume in patients who do not have an indwelling bladder catheter should be performed to help exclude bladder outlet obstruction as a cause of obstructive uropathy. A rectal and pelvic examination may also be helpful in assessing for possible causes of obstructive uropathy. Careful examination of the skin may detect rashes compatible with a drug-induced eruption, which may suggest drug-induced acute interstitial nephritis; palpable purpura, suggesting a microangiopathic hemolytic anemia such as hemolytic-uremic syndrome or thrombotic thrombocytopenic purpura or a systemic vasculitis, such as cryoglobulinemia; and livedo reticularis or digital ischemia, suggesting atheroembolic disease. Assessment of urine composition and examination of the urine sediment can assist in determining the cause of AKI (Table 55.4). The electrolyte composition of the urine may be helpful in differentiating between prerenal azotemia and ATN.127–130 In prerenal azotemia, renal tubular function is intact and the tubules avidly reabsorb sodium in an effort to restore extracellular fluid volume and renal perfusion to normal. Thus, in prerenal azotemia, urine sodium concentrations are usually less than 20 mmol/L and the fractional excretion of sodium [calculated as (UNa/PNa) ÷ (UCr/PCr), where UNa is the urine sodium concentration, PNa is the plasma or serum sodium concentration, UCr is the urine creatinine concentration, and PCr is the plasma or serum creatinine concentration] is less than 1%. In contrast, in ATN, the damaged renal tubular cells fail to reabsorb sodium normally, perhaps because of loss of cellular polarity, with a resultant urine sodium concentration greater than 40 mmol/L and a fractional excretion of sodium above 2%. It needs to be recognized, however, that these urinary electrolyte findings are not absolute. Thus, increased fractional excretion of sodium may occur in prerenal states when diuretics have been administered, in patients with underlying CKD who may take several days after the onset of volume depletion to maximize sodium conservation, in the presence of glycosuria or other osmotic diuresis, or in the presence of bicarbonaturia. A fractional excretion of sodium of less than 1% may be present in multiple settings other than prerenal azotemia, including in the absence of any kidney disease and in many forms of glomerulonephritis, in microangiopathic hemolytic anemia, and in some forms of ATN, including radiocontrast-associated AKI, myoglobinuric ATN, early sepsis-associated ATN, and nonoliguric ATN.131,132 The fractional excretion of urea [calculated as (Uurea/Purea) ÷ (UCr/PCr), where Uurea is the urine urea nitrogen concentration, Purea is the blood urea nitrogen concentration, UCr is the urine creatinine concentration, and PCr is the plasma or serum creatinine concentration] has been proposed as an alternative index of tubular function for the diagnosis of prerenal azotemia in patients who have received diuretic therapy.133–136 The normal fractional excretion of urea is greater than 60%; in reversible prerenal states, values typically fall to less than 35%. Urinary chemical indices are not of value in establishing the diagnosis of obstructive uropathy. Table 55.4 Urinalysis Findings in Acute Kidney Injury (AKI) Urine microscopy is also often very helpful in determining the cause of AKI.137–141 A normal urine sediment suggests the presence of either a prerenal or postrenal cause of AKI, although obstructive uropathy may be associated with hematuria, pyuria, or crystalluria. In the presence of proteinuria a urinary sediment containing abundant cells or casts suggests an intrinsic cause of AKI. Specifically, the presence of many renal tubular epithelial cells, epithelial cell casts, or pigmented (muddy brown) granular casts suggests the diagnosis of ATN and has been associated with increased risk for greater severity of disease.141,142 The presence of white blood cells and white blood cell casts suggests AIN. Although eosinophiluria may be seen in association with interstitial nephritis, it is also associated with atheroembolic disease and with urinary tract infections, and the specificity and sensitivity of this finding are limited.143
Acute Kidney Injury
Definition
Epidemiology
Factor
Frequency (%)
Multiorgan failure
30-75
Sepsis
30-50
Drugs/medications
20-40
Postoperative state
15-35
Cardiogenic shock
15-30
Hypovolemia
15-30
Liver failure
5-10
Pathogenesis
Prerenal Acute Kidney Injury
Postrenal Acute Kidney Injury
Intrinsic Acute Kidney Injury
Acute Tubular Necrosis
Acute Interstitial Nephritis
Acute Vascular Syndromes
Intratubular Obstruction
Clinical Manifestations
Retention of Filtration Markers
Urea
Creatinine
Cystatin C
Markers of Tubular Injury
Oligoanuria
Diagnostic Approach
General Aspects
History, Physical Examination, and Record Review
Urinalysis and Urine Indices
Etiologic Disorder
Urine Chemistry
Urine Sediment
Prerenal
UNa <20 mmol/L
FENa <1%
FEurea <35%
Normal or nearly normal (hyaline casts and rare granular casts)
Acute tubular necrosis
UNa >40 mmol/L
FENa >2%
FEurea >60%
Renal tubular epithelial cells, epithelial cell casts, coarse pigmented (muddy brown) casts
Acute interstitial nephritis
Variable; UNa may be >40 mmol/L, FENa may be >2%
Red blood cells, white blood cells, white blood cell casts, eosinophils
Acute glomerulonephritis
Variable; UNa may be <20 mmol/L, FENa may be <1%
Red blood cells (dysmorphic), red blood cell casts
Acute vascular disease
Variable; UNa may be <20 mmol/L, FENa may be <1%
Red blood cells, red blood cell casts in HUS/TTP, eosinophils in atheroembolic disease
Crystal-associated AKI
Variable
Crystalluria
Uric acid crystals in tumor lysis syndrome
Calcium oxalate crystals in ethylene glycol ingestion
Drug crystals (acyclovir, methotrexate, indinavir, triamterene, sulfadiazine)
Obstructive
Variable; early UNa may be <20 mmol/L, FENa may be <1%; late UNa may be >40 mmol/L, FENa may be >2%
Normal or red blood cells, white blood cells and crystals
Stay updated, free articles. Join our Telegram channel
Full access? Get Clinical Tree
Acute Kidney Injury
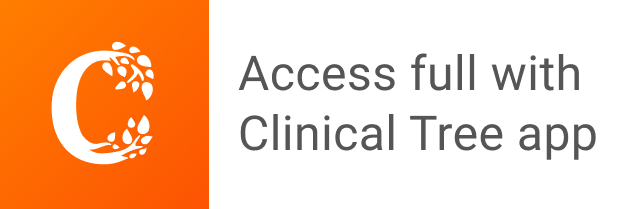