Barry D. Kussman1,2, Amy E. Roberts3, and Wanda C. Miller‐Hance4,5 1 Department of Anesthesiology, Perioperative and Pain Medicine, Boston Children’s Hospital, Boston, MA, USA 2 Department of Anaesthesia, Harvard Medical School, Boston, MA, USA 3 Department of Cardiology and Division of Genetics, Department of Pediatrics, Boston Children’s Hospital and Harvard Medical School, Boston, MA, USA 4 Department of Anesthesiology, Perioperative and Pain Medicine, Arthur S. Keats Division of Pediatric Cardiovascular Anesthesiology, Baylor College of Medicine and Texas Children’s Hospital, Houston, TX, USA 5 Department of Pediatrics, Section of Cardiology; Baylor College of Medicine and Texas Children’s Hospital, Houston, TX, USA Congenital heart disease (CHD) is the most common birth defect, occurring in 0.8% of live births and accounting for almost one‐third of all major congenital anomalies [1, 2]. The etiology and mechanisms of congenital cardiac malformations are not yet fully understood, and much of our understanding is derived from vertebrate animal models and progress in genomics, proteomics, transgenesis, imaging, and integrative systems biology [3]. CHD is known to have genetic and non‐genetic causes, and the multifactorial inheritance of some lesions is probably due to the interactions between several genes, transcriptional programs, and modulating environmental factors, including known teratogens (such as alcohol, isotretinoin, excess vitamin A, thalidomide, anticonvulsants), infectious agents (such as rubella), or maternal diseases (such as diabetes mellitus, obesity, phenylketonuria) [4, 5]. Developmental mechanisms include genetic (somatic or germline variants, chromosomal aneuploidy, copy number variants, point mutations), epigenetic (transcriptional programs, chromatin remodeling factors, histone‐modifying proteins), and hemodynamic and contractile forces [4, 6–10]. Furthermore, the understanding of genes and developmental mechanisms producing CHD has provided an insight into adult cell lineages and has opened the door for using regenerative medicine and novel therapies to treat congenital and acquired heart diseases [11]. It must be stated that much of the knowledge and understanding we have regarding cardiovascular development was derived from research in non‐human species, which was performed with the hope that the same processes occur in humans. We have used an anatomical approach to describe the development of the heart and vascular system and to demonstrate the relationships between abnormal development and specific congenital cardiac anomalies. Although a segmental approach is used to describe embryology, cardiovascular development is a three‐dimensional, spatiotemporal process with many elements occurring simultaneously. Up until the 1990s, the conventional view of cardiac development was based on the segmental model, in which the primordia of all the future components of the heart were hypothesized to be present in the initial heart tube [12]. Many of the classic concepts have been challenged over the last two decades as advancements have been made in labeling and transgenic fate‐mapping techniques. Although the labeling studies performed by Stalsberg, de la Cruz, and Viragh from 1969 onward suggested that cells were added to the poles of the heart from the surrounding mesoderm [13–15], more recent research conducted by Buckingham, Markwald, and others have confirmed that cells are added to the heart after the initial stage of looping [16–19]. As discussed in detail in the following sections, it is now thought that the primitive heart tube contains little more than the precursors for the left ventricle, parts of the atria, and perhaps the atrioventricular (AV) canal, and that the precursor cells of the other cardiac components are added to both the venous and arterial poles from a second heart field outside the initial heart tube [12, 20]. As stated by Christoffels and Jensen, cardiac development is an iterative process of progenitor cell expansion, intercellular signaling, transcriptional responses, patterning, and differentiation into specialized cell types [20]. Elucidation of the genetic and transcriptional networks regulating cardiac development has provided new insights in that abnormal development not only results in CHD but also influences adult‐onset heart disease such as cardiomyopathies, conduction defects, and arrhythmias [21]. The human embryo has no heart or vascular system during the first 2 weeks of life. Instead, it relies on diffusion from the uteroplacental circulation. At the end of the second week, the embryo is a bilaminar disc made up of the epiblast and the hypoblast. In vertebrates, the cardiovascular system is the first organ system to develop and function; this begins during the third week of life when diffusion is no longer adequate to meet embryonic nutritional requirements and to remove metabolic waste. The embryo develops into a trilaminar disc by the process of gastrulation, thereby establishing all three germ layers – the ectoderm, mesoderm, and endoderm. Gastrulation involves cells in the epiblast migrating through the primitive streak caudal to the primitive node. These cells detach from the epiblast (invagination), and some displace the hypoblast to create the embryonic endoderm, others come to lie between the epiblast and endoderm to form the mesoderm, and the remaining epiblast cells form the ectoderm (Figure 6.1) [22]. The epiblast is therefore the source of the ectoderm, mesoderm, and endoderm. Cardiac progenitor cells are located in the epiblast just lateral to the primitive streak. After gastrulation, these progenitor cells in the lateral plate mesoderm migrate laterally and cranially to locate on either side of the midline under the head folds. The lateral plate mesoderm becomes split by the pericardial coelom into the somatic (dorsal) and splanchnic (ventral) layers (Figure 6.2). Cells in the somatic layer will form the pericardium and cardiac progenitor cells located in the splanchnic layer will form the bilateral cardiogenic or heart fields [24]. These cardiac progenitor fields express the transcription factor NKX2.5 throughout the left ventricle and atrial chambers, a member of the Homeobox‐containing genes that play critical roles in regulating tissue‐specific gene expression essential for tissue differentiation and for temporal and spatial patterns of development [25]. The heart fields merge in the midline, cranial to the stomatopharyngeal membrane, to form the cardiac crescent (Figure 6.3A,B). There are at least two distinct lineages of cells within the cardiac crescent: one population is referred to as the “first heart field” (FHF), and the other is referred to as the “second heart field” (SHF) [10]. The SHF is contiguous with and located dorsal and medial to the FHF. The FHF will give rise to the linear heart tube and eventually gives rise to the left ventricle (LV) and parts of the right and left atria [27]. Cells from the SHF will be added at the inflow (venous) and outflow (arterial) poles during cardiac looping, ultimately giving rise to the right ventricle (RV), parts of the atria, septum and outflow tract, and base of the aorta and pulmonary trunk. Cardiac cell lineage and specification are shown in Figure 6.4 [27]. Differentiation of the heart field cells into the myocardium is dependent on signals from the neural and dermal ectoderm and adjacent endoderm [28]. The proliferation and differentiation of SHF cells are delayed relative to those of the FHF cells. As discussed in the following sections, the embryonic cells that contribute to cardiac development arise not only from the heart field mesoderm but also from the cardiac neural crest [29] and the proepicardium [30]. Figure 6.1 Formation of three cell layers. Cross section through the cranial region of the streak at 15 days showing invagination of epiblast cells. The first cells to move inward displace the hypoblast to create the definitive endoderm. Once definitive endoderm is established, inwardly moving epiblast forms mesoderm. (Source: Sadler [22]. Reproduced with permission of Wolters Kluwer. The primitive linear heart tube is formed during the fourth week of development from the FHF cells with the folding of the embryo. Contrary to originally held beliefs, the cardiogenic fields do not fuse cranially to caudally in a zipper‐like fashion. Folding of the embryo and invagination of the foregut brings the lateral portions of the cardiac crescent together that then fuse at the midline from anterior (cranial) to posterior (caudal) to form the ventral part of the heart tube [20, 26] (Figure 6.2 and Figure 6.3C–G). The medial portions of the cardiac crescent will form the dorsal part of the heart tube, which is open to the ventral foregut endoderm and is suspended from the foregut by the dorsal mesocardium. The myocardium of the heart tube is called primitive or primary because, unlike adult working myocytes, the myocytes of the heart tube have few contractile elements, a poorly developed sarcoplasmic reticulum, a low density of gap junctions, and high automaticity [12]. The heart tube has two caudolateral inlets (venous pole) and one craniomedial outlet (arterial pole) [26, 31]. The endocardium is also derived from the splanchnic mesoderm and develops simultaneously with the myocardium in the cardiac crescent. Adjacent endodermal cells release promoters and antagonists of bone morphogenic protein (BMP), fibroblast growth factor (FGF), and wingless‐type (WNT) families of growth factors for differentiation of the cardiac crescent cells into cardiomyocytes (29). T‐box transcription factors are key regulators of the fate of cardiomyocytes. Tbx5 and Tbx20 are activators of the chamber program of gene expression. Antagonists of working myocardium gene expression, TBX2 and TBX3, allow the inflow tract, AV canal, and outflow tract myocardium to develop. Tbx1 is expressed in outflow tract precursors and Tbx18 at the venous pole [26]. There are direct correlations between this specific programming and the variants that alter protein function and lead to CHD in humans. Pathogenic germline variants, for example in TBX1, have been reported in tetralogy of Fallot [32]. A distinct population of mesodermal cells in each heart field undergoes de novo vasculogenesis into two hollow endocardial tubes that join to form a single tube, which becomes enveloped by the cardiomyocytes. The endocardium becomes contiguous with the endothelium of the developing vasculature. The cardiac jelly, a thick acellular matrix secreted by the myocardium, lies between the myocardium and endocardium. The initial heart tube is therefore a tubular structure with an inner endocardial layer, a middle layer of cardiac jelly, and an outer myocardial layer. Figure 6.2 Diagrammatic representation of the formation of the heart, foregut pocket, and coelom in the chick embryo. AIP, anterior intestinal portal. (Source: Kirby [23]. Reproduced with permission of Oxford University Press.) Figure 6.3 Folding of the embryo and formation of the heart tube. (A) The embryo starts as a flat disc containing the three germ layers, the ectoderm (Ecto), mesoderm (Meso), and endoderm (Endo). (A–A‴) With the ongoing folding of the embryo, the embryonic gut that runs from the stomatopharyngeal membrane (SM) to the cloacal membrane (CM) is formed. The heart (HT) becomes positioned ventrally to the foregut (FG), caudally to the head, and cranially to the umbilical cord and transverse septum (TS). HN, Hensen’s node or primitive node. (B) Division of the heart‐forming field into the first heart field (1), which will give rise to the linear heart tube and the second heart field (2), which will remain in continuity with the first heart field during subsequent development and from which cardiomyocytes are added to the developing heart. In reality, the strict borders drawn here are gradual. PM, pharyngeal mesoderm. (C–G) Formation of the heart tube from a flat horseshoe‐shaped cardiac crescent to a tube. Folding of the embryo brings the lateral portions of the cardiac crescent (red line) together to form the ventral part of the heart tube, while the medial portions of the cardiac crescent (blue line) will form the dorsal part of the heart tube, which is suspended from the foregut by the dorsal mesocardium (DM). After the DM closes and the suspension from the foregut is lost, cells of the second heart field can only be added to the heart via the arterial and venous poles (AP and VP, respectively). CS, Carnegie stage (noted along right side of figure). (Source: Sylva et al. [26]. Reproduced with permission of John Wiley & Sons.) The schematic drawings in older embryology textbooks illustrating the presence of all the cardiac segments in the straight heart tube prior to looping are hypothetical constructions, as most of the segments are added to the primitive heart tube during the looping stages [33]. The primitive atria and ventricles begin to contract around day 22 as the heart tube loops to the right, with circulation through the embryo beginning around day 26. Flow in the valveless heart tube is unidirectional, and because the highest automaticity is found at the venous pole, traveling mechanical waves sweep blood from the venous to the arterial pole [34]. Clearly, failure of the heart tube to develop (acardia) leads to fetal demise, and as shown in the zebrafish, failure of the bilateral heart fields to fuse results in cardia bifida (two separate hearts in lateral positions) [35]. Figure 6.4 Early cardiac development. (A) Cardiac cell lineage and specification during development demonstrate the commitment of pluripotent cells toward mature cardiac cell types within the heart development. (B) Schematic of cardiac morphogenesis in humans. At the second week of gestation, the cardiogenic mesodermal cells migrate toward the anterior side of the embryo to form the FHF or cardiac crescent and SHF that are specified to form specific segments of the PHT, which is patterned along the anteroposterior axis to form the various regions and chambers of the looped and mature heart during weeks 3 and 4. The FHF gives rise to the beating PHT and will eventually give rise to the LV and parts of the right and left atria (RA and LA, respectively). The SHF, located behind the PHT and within the pharyngeal mesoderm by gestational week 3, will contribute to the formation of the RV, parts of the atria and outflow tract, and later to the base of the aorta and pulmonary artery. At gestational week 3, the cells at the venous pole contribute to the formation of the superior and inferior vena cavas (IVC, respectively). By gestational week 4, the cardiac neural crest cells migrate in from the dorsal neural tube, forming smooth muscle cells within the aortic and pulmonary arteries. In addition, the proepicardial organ formed by the proepicardial progenitor cell clusters later contributes to the formation of the epicardium. The four chambers form by the end of week 7. Wnt, Wingles integrated; FGF, fibroblast growth factor; BMP, bone morphogenetic proteins; FHF, first heart field; SHF, second heart field; OFT, outflow tract; PHT, primary heart tube; PM, pharyngeal mesoderm; VP, venous pole; CNCCs, cardiac neural crest cells; LV, left ventricle; RV, right ventricle; PEO, proepicardial organ; SVC, superior vena cava; IVC, inferior vena cava; EPC, epicardium; and PA, pulmonary artery. (Source: Tan [27] / S. Karger AG / CC BY 4.0) Looping is a crucial process in cardiac morphogenesis that brings the tubular configuration of the primitive circulation into the correct conformation for chamber specification, septation, and the creation of systemic and pulmonary pathways [33, 36]. The heart is the first organ not only to function but also to develop a bilateral asymmetric form. Cardiac asymmetry is attained during the process of looping. At first, the heart tube is straight in the ventral midline with paired venous limbs at its caudal end and a single arterial outlet connected to the aortic sac and pharyngeal arch arteries at its cranial end (Figure 6.5) [37, 38]. Looping begins with ventral bending (from dorsal to ventral) and the loss of the dorsal mesocardium, which suspends the heart tube from the foregut (ventral pharynx); these changes transform the straight tube into a curved tube (Figure 6.6). This is followed by a rotation around the craniocaudal axis to the right (dextro‐ or D‐looping), bringing the left side of the tube to a ventral (front) position, which is seen as a C‐shaped loop with the convexity to the right in a ventral, two‐dimensional view [33]. At this stage, the junction of the inflow and outflow limbs is at the deepest convexity. Externally, the junction corresponds to the bulboventricular groove, and internally it corresponds to the bulboventricular fold [39]. Subsequent looping produces a ventral, two‐dimensional, S‐shaped loop (Figure 6.7). The heart tube lengthens as cells are added in a continuous stream to the venous (inflow) and arterial (outflow) poles by the addition of myocardium (cardiomyocytes) and endocardium from the SHF in the adjacent pharyngeal mesoderm. The poles are the only entryway for new cells from the SHF [40]. The ventricular bend then shifts caudally from its cranial position above the atria, and the inflow region shifts cranially, thereby resulting in the convergence of the inflow and outflow poles. After looping, there is rotation (untwisting) of the outflow tract, which results in a leftward shift of the outflow tract. The complex process of cardiac looping is shown in schematic form in Figure 6.8. Wedging is the process in which the aorta will come to nestle between the mitral and tricuspid valves and behind the pulmonary trunk (Figure 6.9) [36]. When looping is complete, the named segments of the heart (which are extremely variable between authors) are the sinus venosus, common atrium, AV canal, presumptive left ventricle, presumptive right ventricle, conus arteriosus, and truncus arteriosus (frequently referred to as the conotruncus) [41]. Figure 6.5 Straight heart tube. Scanning electron micrograph of a chick embryo (HH‐stage 9), viewed from the front, showing the “straight heart tube” with paired venous limbs at its caudal end and an arterial outlet at its cranial end, which is connected to the aortic sac. A, arterial outlet; AIP, anterior intestinal portal; LVL, left venous limb; RV, primordium of the trabeculated portion of the morphologic right ventricle; RVL, right venous limb. (Source: Männer [33]. Reproduced with permission of John Wiley & Sons.) Cardiac looping is associated with the breaking of the initial bilateral symmetry of the embryo and the establishment of the left‐right axis [36, 37]. The left‐right axis is established during gastrulation by nodal cilia at the anterior border of the primitive streak. The cilia sweep from right to left, resulting in a leftward flow of extracellular fluid which concentrates a putative secreted factor on the left side of the embryo. Binding of this factor to its receptors triggers asymmetrical gene expression at the node and expression of Nodal (a member of the TGFβ family), Southpaw and Lefty2 [25, 42]. The left‐sided expression of Nodal is crucial in establishing the left‐right axis. Animal models with absent Lefty1 demonstrate thoracic left isomerism and bilateral expression of Nodal, Lefty2, and Pitx2c, implying that Lefty1 restricts expression of Nodal and Lefty2 on the left side and the Nodal and/or Lefty2 signal development [32]. Pitx2c (a member of the homeobox gene transcription factor family) is a key regulator of cardiac left‐right patterning that acts downstream of Nodal. PITX2c expression on the left side persists long after Nodal expression [26]. Left ventricle chamber identity is associated with HAND1 expression and right ventricular chamber identity with HAND2 expression [43]. Left‐right signaling pathways determine not only the direction of looping and the topology of the future ventricular chambers but also the proper development of the atria, the arterial and venous poles, and the systemic vasculature. Abnormalities in left‐right patterning increase the incidence of cardiac malformations in both D‐ and L‐loop hearts; typical examples include mirror imagery, atrial isomerism, discordant connections, and heterotaxy [44]. The left‐right signaling cascade is hierarchical because abnormalities high in the cascade result in randomization of situs, whereas abnormalities lower down, which are associated with tissue‐specific signaling, produce discordant connections [26]. Normal cardiac looping to the right, referred to as dextro‐looping or D‐looping, positions the right ventricle towards the right side and leaves the heart lying predominantly in the left hemithorax with a leftward apex (levocardia) (Figure 6.10, left panel). Situs solitus refers to normal looping with the liver, stomach, and spleen in their normal positions. Rotation around the craniocaudal axis to the left results in a levo‐loop (L‐loop) heart (Figure 6.10, right panel), in this position the right ventricle is positioned toward the left and the left ventricle toward the right. Congenitally corrected transposition of the great arteries (CCTGA) arises when the heart (ventricle) loops to the left but the atria and outflow receive correct left‐right signals, resulting in both AV and ventriculoarterial (VA) discordance. CCTGA can also occur when the heart loops to the right with inversion of the atria and VA discordance. Situs inversus totalis refers to an L‐loop heart with mirror‐image reversal, which means the heart lies predominantly in the right hemithorax with a rightward apex (dextrocardia) and there is a mirror‐image reversal of the liver, stomach, and spleen. Mesocardia refers to a midline heart with the apex pointing inferiorly or anteriorly. Mesocardia and dextrocardia can be associated with a normal or abnormal arrangement of cardiac structures. Atrial isomerism refers to a bilaterally symmetrical pattern of the atrial appendages (i.e. bilateral leftness or rightness) and is usually associated with severe complex cardiac malformations. Abnormal PITX2c expression results in both atria developing right atrial appendages, establishing a role of PITX2c in the differentiation of atrial sidedness [45]. Mixed situs occurs when some organs, or components thereof, have normal situs and others have situs inversus. This usually results in complex heart defects known as heterotaxia or heterotaxy syndromes (discussed later in the chapter). Figure 6.6 Steps in looping of the heart tube. The heart tube forms ventral to the foregut and is open to it at first. Then the heart tube is suspended at its nascent inner curvature from the ventral foregut by the dorsal mesocardium which quickly disappears. Ventral bending occurs first, followed by rotation to the right which brings the left side of the tube to the front and the inner curvature to the left side. Subsequent looping to form the S‐shaped loop involves the addition of cells at the inflow and outflow poles. DM, dorsal mesocardium; FG, foregut. (Source: Kirby [23]. Reproduced with permission of Oxford University Press.) Figure 6.7 Looping morphogenesis. Scanning electron micrographs of embryonic chick hearts, viewed from the front, showing the main positional changes of the developing heart chambers of higher vertebrate embryos during cardiac looping. (A) “C”‐shaped loop at early stages (HH‐stage 12). (B) “S”‐shaped loop at advanced stages (HH‐stage 17/18) of cardiac looping. RV, right ventricle; LV, left ventricle. (Source: Männer [37]. Reproduced with permission of Elsevier.) Cardiac septation begins after looping of the heart tube. It consists of four contemporaneous processes that occur between 27 and 37 days of development, dividing the heart into four chambers and creating separate systemic and pulmonary circulations [22]. Septation is attributable to the muscular septa in the atrial and ventricular chambers, the AV endocardial cushions, the outflow tract endocardial cushions, and the dorsal mesenchymal protrusion. TBX2/3 and BMP2/4 are important repressors of chamber‐specific genes like ANF to allow for the development of the nonchamber myocardium [25]. Indeed, deletion of either Bmpr1a or Tbx2 leads to the development of additional myocardial connections [46, 47]. Atrial septation takes place in phases to maintain right‐to‐left atrial shunting and involves the septum primum, septum secundum, and AV canal (AVC) septum. The primary atrial septum (septum primum) grows as a muscular crescent from the craniodorsal wall of the atrium on the right of the pulmonary pit toward the AV endocardial cushions (Figure 6.11). The leading edge of the septum primum is covered by a mesenchymal cap produced by the endothelial‐to‐mesenchymal transformation (EMT) of the endocardium covering the septum [40]. EMT is a complex biologic process whereby endothelial cells lose their specific phenotype and evolve into cells with a mesenchymal phenotype that includes spindle‐shaped elongated cell morphology, loss of cell‐cell junctions and polarity, and acquisition of functions such as cellular motility, contractility, and invasiveness [48]. This mesenchymal cap is continuous with the protrusion of the dorsal mesocardium (dorsal mesenchymal protrusion) and the dorsal (superior) and ventral (inferior) AV endocardial cushions [26]. The communication between the leading edge of the septum primum and the AV cushions is known as the primary atrial foramen (ostium primum), which closes when the septum primum becomes contiguous with the fused dorsal and ventral AV endocardial cushions. Communication between the left and right atria is maintained by the detachment of the septum primum from the roof of the atrial cavity to produce a secondary atrial foramen (ostium secundum) [49]. The secondary atrial septum (septum secundum) is formed by the folding of the dorsal wall between the primary atrial septum and the left leaflet of the sinoatrial valve. The septum secundum does not fuse with the AV cushions but remains open as the foramen ovale. The cranial portion of the septum primum remains as a flap valve, which fuses with the edges of the foramen ovale after birth to form the fossa ovalis [40]. The muscular septum between the inferior rim of the fossa ovalis and the AV valves is called the AVC septum. Figure 6.8 Ventricular looping. Schematic drawing (frontal views) of the four components of normal ventricular looping: (1) ventral bending transforms a straight tube into a curved tube whose outer curvature is formed by its ventral wall; (2) torsion around its original cranio‐caudal axis transforms a curved tube into a helically wound loop which appears C‐shaped in frontal views; (3) caudal shift of the ventricular segment; (D) untwisting is characterized by ventral and leftward shift of the proximal outflow tract, ventral shift of the primitive right ventricle, and rightward shift of the atrioventricular canal. A, common atrium; LV, embryonic left ventricle; O, outflow tract; RV, embryonic right ventricle. (Source: Männer [33]. Reproduced with permission of John Wiley & Sons.) Figure 6.9 Steps in aortic wedging (A–E). The aortic side of the outflow tract nestles between the mitral and tricuspid valves as the outflow myocardium is remodeled. AVC, atrioventricular canal. (Source: Kirby [23]. Reproduced with permission of Oxford University Press.) Early in development, the venous pole of the heart has two channels (the left and right horns of the sinus venosus), returning blood from the embryo (via the cardinal, vitelline, and umbilical veins) to the sinus venosus and common atrium. During the convergence phase of looping, the common atrium expands to the right and left, forming two lateral pouches that will become the right and left atrial appendages [33, 50]. Remodeling results in the sinoatrial junction shifting to the right side of the common atrium. The right horn of the sinus venosus and the sinoatrial junction become incorporated into the dorsal (back) wall of the right atrium (Figure 6.12). The sinoatrial junction has left and right craniocaudally oriented valves (venous valves) that meet cranially to form the septum spurium [50]. As the distal portion of the left superior cardinal vein regresses (and thereby absent left superior vena cava), the left horn of the sinus venosus becomes smaller and is incorporated into the developing AV groove to become the coronary sinus. The dorsal (back) wall of the left atrium is also formed by the incorporation of the pulmonary vein and its surrounding myocardium [39, 50]. Figure 6.10 Handedness of the cardiac loop. Scanning electron micrographs of embryonic chick hearts, viewed from the front, showing the so‐called D‐loop (dextral‐loop) (A) and L‐loop (levo‐loop) (B) configurations (“C‐shaped” loops; HH‐stage 12). (Source: Männer [37]. Reproduced with permission of Elsevier.) Figure 6.11 Schematic drawing illustrating septation of the atria and primary foramen. (A) Chamber‐forming heart with the atrioventricular canal (AVC) and outflow tract (OFT) cushions and ridges. The two arrows going down through the AVC into the ventricle represent blood flow during diastole. The two arrows pointing toward the OFT represent blood flow during systole. Note that the primary foramen (PF) is the crossroad of the blood running from the right atrium (RA) to the right ventricle (RV), and the blood running from the left ventricle (LV) to the OFT. (B–F) Sagittal sections at the level of the dotted line in (A). (B) The ventral and dorsal endocardial cushions (1 and 2, respectively) are growing toward each other. (C) The primary atrial foramen (PAF, ostium primum) is closing due to ingrowing of the primary atrial septum (PS, septum primum), with its mesenchymal cap (MC), the dorsal mesenchymal protrusion (DMP), and the endocardial cushions. (C, D) In the PS, small holes appear and merge to form the secondary foramen (SF, ostium secundum). (D–F) The secondary septum (SS, septum secundum) grows to the right side of the PS covering the SF and the rest of the PS, and leaving at the right surface of the atrial septum only the oval fossa (OF) uncovered. A, atrium; LA, left atrium; V, ventricle; 3 and 4, septal and parietal outflow tract ridges, respectively. (Source: Sylva et al. [26]. Reproduced with permission of John Wiley & Sons.) The most common cause of an atrial‐level shunt is a secundum atrial septal defect (ASD), which is located within the region of the fossa ovalis. It is usually due to a deficiency of the septum primum but, in rare cases, it can be caused by the deficiency of the septum secundum [51]. Failure of the septum primum and septum secundum to fuse completely during infancy results in a patent foramen ovale (PFO). As long as the left atrial pressure exceeds the right atrial pressure and the flap valve is large enough to cover the boundaries of the fossa ovalis, the foramen remains functionally closed (probe patent foramen). An ostium primum defect results from the failure of the septum primum to fuse with the endocardial cushions. The defect is outside the confines of the fossa ovalis and extends from the inferior limbus of the fossa ovalis to the crest of the interventricular septum. An ostium primum defect is part of the family of AVC defects, also referred to as AV septal and endocardial cushion defects and may occur in isolation or in association with other abnormalities of the AV junction. A sinus venosus defect occurs in the area derived from the embryologic sinus venosus (posterior aspect of the right atrium) so is not strictly a defect in the atrial septum. It is an interatrial communication caused by a deficiency of the common wall between the superior vena cava (SVC) and the right‐sided pulmonary veins [52, 53]. The most common location for this defect is between the right upper pulmonary vein and the cardiac end of the SVC type, although in rare cases the right lower and/or middle pulmonary veins and more caudal atrial wall are involved (inferior vena cava [IVC] type) [51]. A coronary sinus defect occurs because of unroofing of the normal course of a persistent left anterior cardinal/superior caval vein into the left horn of the sinus venous, i.e. the tissue between the coronary sinus and the left atrium is either partially or completely absent (unroofed) resulting in communication between the right and left atria via the coronary sinus orifice [53]. The left‐to‐right shunt causes enlargement of the coronary sinus orifice. Figure 6.12 Formation of the venous pole. (A–F) Cross‐sections of three‐dimensional (3D) reconstructions of human hearts (gray, myocardium; blue, systemic veins; orange, pulmonary veins; yellow, cardiac mesenchyme). (A, B) Developing right and left atrium (RA, LA); the RA is connected to both the right superior (RSCV) and inferior caval vein (ICV) as well as the left superior caval vein (LSCV), via the coronary sinus (CS). At this stage, the entire systemic venous pole is connected to the right atrium through one orifice, flanked by the left and right venous valves (LVV and RVV). The primary septum (PS) is growing to the right of the opening of the pulmonary vein (PV). (C, D) The RSCV and CS are still connected to the atrium via a single orifice, which has now been muscularized. The secondary foramen (SF) appears in the primary septum. (E, F) All veins connect separately to the atrium via myocardialized orifices. The primary atrial foramen (PAF) is closed, and the secondary septum (SS) is growing between the PS and the LVV, which is already partially fused to the secondary septum. The RVV can now be separated into a part that flanks the CS and a part that flanks the RSCV, the future Thebesian and Eustachian valves, respectively. OF, oval foramen; CS, Carnegie stage (noted on the left margin of figures). (Source: Sylva et al. [26]. Reproduced with permission of John Wiley & Sons.) A common atrium is caused by the absence of the septum primum, septum secundum, and atrial portion of the AVC septum and is usually associated with the heterotaxy syndrome [51]. After looping of the heart tube occurs, differentiation and re‐initiation of cell division by the primary myocardium of the outer curvature cause the ventricles to expand caudally in a pouch‐like fashion on either side of the bulboventricular groove (Figure 6.13). De Boer and colleagues [54] have shown that growth of the trabeculae occurs by cellular proliferation at the bases, causing the ventricles to expand or “balloon outward” (ballooning model of chamber formation). The disappearance of the cardiac jelly results in the formation of trabeculations on the luminal side, producing a spongy‐type myocardium. Compact myocardium forms later when epicardially derived fibroblasts infiltrate the epicardial side, at which time proliferation in the trabeculations ceases. Figure 6.13 Formation of the cardiac chambers. (A–D) Developmental series of mouse embryos. Whole‐mount RNA in situ hybridization for the embryonic chamber marker atrial natriuretic factor (ANF) is used as a marker for differentiation into chamber myocardium. (E–G) and (I–L) Schematic drawings of these chamber‐forming hearts. Gray, primary myocardium; blue, chamber‐forming myocardium; arrows in K indicate the expansion of the chambers eventually leading to the adult configuration, with the ventricles positioned ventro‐caudally to the atria. (H) Electron micrograph of a CS14 human heart, demonstrating the similarity with the mouse E11.5 heart and the schematic shown in (L). For didactic purposes, in the schematic in (L), the outflow tract is hinged toward the right side, in vivo it is positioned ventrally to the heart, as depicted in (D) and (H). (A, E, I) The heart tube (HT) consists solely of the primary myocardium from the venous (VP) to the arterial pole (AP). (B, F, J) The first chamber to start ballooning is the embryonic ventricle (V) at the outer curvature of the heart. (C, G, K) The heart tube has started to loop and acquire an S shape. An embryonic left and right ventricle are now visible. The atria (A) also start to balloon towards the left and right side. The myocardium of the outflow tract (OFT), inner curvature (IC), and atrioventricular canal (AVC) remain as the primary myocardium. RA, right atrium; LV, left ventricle; CS, equivalent Carnegie stage noted in the left margin of figures. (Source: Sylva et al. [26]. Reproduced with permission of John Wiley & Sons.) The muscular portion of the interventricular septum is formed by apposition and merger of the medial walls of the expanding ventricles, with cells added mainly from the adjacent left ventricular free wall [22]. The septum represents the least changed part of the lumen of the embryonic heart and the superior portion expresses a transcriptional repressor, TBX3 [55]. It is initially crescent‐shaped, extending from the ventral (inferior) AV cushion posteriorly to the dorsal (superior) AV cushion anteriorly. The space between the free rim of the muscular septum and the fused AV cushions is the primary interventricular foramen. Although the primary interventricular foramen allows for communication between the primitive left and right ventricles, initially blood from the primitive atrium can only reach the primitive right ventricle via the interventricular foramen (Figure 6.11). It is only after the AV junction develops that the right atrium will communicate directly with the right ventricle. Closure of the interventricular foramen occurs by fusion of three tissue components: the dorsal (superior) and ventral (inferior) AV endocardial cushions, the muscular interventricular septum, and the endocardial cushions of the outflow tract (conal cushions). The septal conal cushion extends deep into the future right ventricle to merge with the dorsal AV cushion and the rightmost edge of the developing muscular septum [56]. The site where these three structures fuse constitutes the membranous part of the ventricular septum. The ventricular septum is fully closed at an estimated gestational age of 9 1/7 weeks [57]. A ventricular septal defect (VSD) is an opening or hole in the interventricular septum [58]. It may be found in isolation or may co‐exist with other congenital cardiovascular anomalies. Because the ventricular septum is composed of three components (muscular, AVC endocardial cushions, and outflow tract [conal] cushions), defects at the ventricular level may result from a deficiency of one or more of these structures and/or from their malalignment. The classification of VSDs can be confusing because of different nomenclatures used by various authors [59]. The most common VSDs are those that occur in the muscular portion of the interventricular septum [2]. This type of VSD is surrounded by a rim made completely of muscle. They are often multiple and may be located anywhere in the muscular septum (apical, anterior, mid, or posterior). The membranous septum is a small, translucent structure. It is adjacent to the anteroseptal commissure of the tricuspid valve when viewed from the right side and is located below the non‐coronary leaflet of the aortic valve when viewed from the left. Small defects may spontaneously close after birth due to the presence of the surrounding fibrous tissue (also referred to as aneurysmal tissue). Defects that extend beyond the boundaries of the membranous septum are called para‐ or perimembranous defects. Membranous VSDs are closely associated with the bundle of His, increasing the risk of causing conduction disturbances and complete heart block during the repair. A conoventricular or subaortic defect is situated between the conal and muscular portions of the ventricular septum and is also close to the bundle of His. This defect is often related to malalignment of the muscular and conal portions of the ventricular septum. A subpulmonary, conal, supracristal, or doubly committed subarterial defect is located in the outflow tract (conal septum) just below the pulmonary valve. Fibrous continuity between the aortic and pulmonary valves is frequently present. The aortic valve (right cusp or non‐coronary cusp) may prolapse into the defect, which may result in aortic regurgitation. An inlet or AVC‐type VSD occurs when the inferior endocardial cushion does not fuse with the muscular component of the ventricular septum. The defect is located in the inlet portion of the right ventricle beneath the tricuspid valve and may be perimembranous or muscular [59]. The origin of the AV node and the course of the AV bundle (His) differ with respect to the normal heart, increasing the risk of injury during surgical repair. A common or single ventricle, defined as the presence of two AV valves with one ventricular chamber or a large dominant ventricle with a diminutive or hypoplastic opposing ventricle may result from various mechanisms. The pathology may be due to the arrest of or a defect in interventricular septation or from poor alignment of the common AV valve with the ventricles [60]. A wide spectrum of functional single ventricles is recognized. These ventricles include double‐inlet single ventricle (usually left), defects resulting from atresia or absence of either the right or left AV valves, such as tricuspid atresia, mitral atresia (part of hypoplastic left heart syndrome [HLHS]), those with an atretic semilunar valve (HLHS, pulmonary atresia with intact ventricular septum), unbalanced common AVC defects, and some forms of double‐outlet right ventricle. The cardiac jelly at the AV junction (and outflow tract) forms into mounds by the myocardial synthesis of the extracellular matrix. The endocardial cushions arise from the cells of the endocardium overlying these swellings that invade the cardiac jelly and undergo EMT into fibroblast‐like mesenchymal cells [61, 62]. AV canal and outflow tract myocardium produce BMPs that signal through the cardiac jelly to the endocardium. As a result, TGF‐beta and Notch signals regulate the EMT of the cushions [63]. Prior to valve formation, the endocardial cushions and underlying myocardium prevent the backflow of blood. Four cushions develop in the AVC: the inferior (ventral) and superior (dorsal) cushions located centrally and two smaller cushions on the lateral walls (left and right). Central fusion of the inferior and superior cushions forms the AV septum, thereby separating the AVC into the left (mitral) and right (tricuspid) orifices [64]. The atrial portion of the AV septum extends from the level of the valve annuli to fuse with the primary atrial septum, whereas the ventricular portion of the AV septum extends from the level of the valve annuli to form the inlet portion of the interventricular septum. The AVC expands to the right, as does the right ventricle, to allow for direct communication between the presumptive right atrium and the presumptive right ventricle (Figure 6.11). The septal leaflet of the tricuspid valve and the anterior leaflet of the mitral valve are derived from the fused inferior and superior endocardial cushions, whereas the mural leaflets are derived from the lateral cushions [65]. Mature AV valves and the subvalvular apparatus are formed by cavitation and remodeling of the cushions and excavation of the underlying ventricular myocardium to form leaflets and chordae tendinae (Figure 6.14) [65, 66]. Late in development, the primitive leaflets undergo lengthening and delamination with the disappearance of the myocardial layer. After the endocardial cushions have fused, the fibroblast‐like cells of the cushions are replaced by myocardial cells in a process called myocardialization. Fusion of the nonmyocardial mesenchymal cushions and myocardialization create part of the atrial septum, ventricular septum, and conal septum, as well as the substrate for the crux of the heart. Figure 6.14 Development of the atrioventricular (AV) and outflow tract (OFT) valves and their contributing tissues. (A–H) Sections through mouse hearts in a plane comparable to the schematic heart in panel (S). (B, D, F, H) The lineage contributions of the epicardium is displayed at different developmental stages. The epicardial lineage marker WT1 was used; epicardial lineage is depicted in red, myocardium in green. (I–P) Schematic drawings of valve development in both the atrioventricular canal and outflow tract. Red, epicardium (Ep); gray, primary myocardium; and yellow, endocardial cushion tissue (EC). Note that in the outflow tract the cells are primarily neural crest‐derived and not endocardial‐derived as in the AV canal. (P) The contribution of the different cushions and ridges to the eventual valves (panel T) is depicted. (Q, R) Display the proepicardium (PE) in a 3‐day‐old chick embryo. The PE is attached to the heart tube (HT) and spreads out to form the epicardium. Ao, aorta; DAVC, dorsal AV cushion; IR, intercalated ridge; LA, left atrium; LC, lateral cushion; LV, left ventricle; MC, mesenchymal cap; PR, parietal ridge; PT, pulmonary trunk; RA, right atrium; RV, right ventricle; S, septum; SR, septal ridge; VAVC, ventral AV cushion. Equivalent Carnegie stage (CS) is noted in the left margin of the figures. (Source: Sylva et al. [26]. Reproduced with permission of John Wiley & Sons.) The endocardial cushions of the AVC contribute to the formation of the atrial septum, the ventricular septum, and the mitral and tricuspid valves. They form between the atrium and ventricle in a vascular endothelial growth factor‐dependent manner. Variants in vascular endothelial growth factor (VEGF) are implicated as a modifier gene in valvuloseptal CHD [67]. Endocardial cushion‐derived structures can have varying degrees of abnormalities producing a spectrum of lesions called endocardial cushion defects (as previously noted, they are also referred to as AVC or AV septal defects). Failure (incomplete or complete) of the endocardial cushions to fuse creates a defect in the AV septum. A partial (incomplete) AVC defect results from incomplete fusion of the superior endocardial cushion with the septum primum and consists of an ostium primum ASD with distinct left and right valve annuli, and frequently there is a mitral valve cleft with varying degrees of regurgitation [68]. A transitional AVC defect is typically an ostium primum defect with a very small to moderate (restrictive) inlet VSD, which is often occluded by AV valve tissue. Although there are two separate orifices, the valves are abnormal [68]. Lack of fusion of the superior and inferior endocardial cushions leads to a complete AVC (CAVC) defect comprising an ostium primum ASD, a non‐restrictive VSD just below the AV valve (inlet VSD), and a common AV valve annulus that bridges both the left and right sides of the heart, i.e. the valve leaflets are shared between the left and right ventricles and are not adherent to the crest of the ventricular septum. In the normal heart, the septal leaflet of the tricuspid valve inserts lower (more apical) than the anterior leaflet of the mitral valve and the AV septum is that portion between the hinge points of the tricuspid and mitral valves. With AVC defects, the mitral valve (left AV valve) insertion is at the same level as the tricuspid valve (right AV valve). This displacement of the common hinge plane of the mitral valve towards the apex reduces the inlet‐to‐apex distance and increases the apex‐to‐outlet ventricular dimension, resulting in a “gooseneck” deformity of the left ventricular outflow tract with the potential to cause left ventricular outflow tract obstruction [69]. A balanced CAVC defect has appropriately sized left and right ventricles and the common AV valve is distributed equally over the ventricular masses. In an unbalanced CAVC defect, one ventricle is hypoplastic with the other ventricle receiving most of the AV valve tissue and ventricular blood flow because of straddling (insertion of chordae in the opposite ventricle) or override (insertion of chordae to the crest of the septum or the appropriate ventricle). AVC defects may be isolated or may appear as one element of a more complex lesion or syndrome. Approximately 40% of patients with trisomy 21 (Down syndrome) have AVC defects [66]. Ebstein anomaly is the most important cause of congenital tricuspid regurgitation and is thought to be due to abnormal delamination of the inlet zone of the right ventricle [70]. The septal and posterior leaflets do not attach to the annulus but are displaced downward toward the right ventricular apex. The portion of the right ventricle that extends from the true tricuspid annuls to the level where the septal and posterior leaflets attach is thin due to partial absence of myocardium and is termed the “atrialized” portion, whereas the trabecular and outlet portions make up the functional right ventricle. The anterior leaflet is large (“sail‐like”) and dysplastic and can obstruct the right ventricular outflow tract. Ebstein anomaly can be associated with abnormalities in left ventricular morphology, such as ventricular non‐compaction and increased fibrosis of the wall and septum. Most patients with this anomaly have a PFO, and approximately 50% have an ASD [71]. Downward displacement of the leaflets is associated with discontinuity of the central fibrous body, thus producing a substrate for accessory AV connections and ventricular pre‐excitation [70]. Tricuspid atresia is characterized by agenesis of the tricuspid valve and right ventricular hypoplasia. The region normally occupied by the tricuspid valve may be replaced by an atretic membrane, muscular tissue, or fibrofatty tissue, and an ASD is necessary for survival. With normally related great arteries, blood passes to the lungs through a VSD into a small right ventricle and pulmonary artery, whereas with transposition of the great arteries, blood reaches the lungs directly from the left ventricle. Isolated tricuspid stenosis is exceedingly rare. Mitral valve stenosis is most commonly associated with other left‐sided obstructive lesions, HLHS being at the most severe end of the spectrum and coarctation of the aorta, aortic arch hypoplasia, and subvalvular or valvular aortic stenosis in Shone’s complex occurring with varying severity [72]. Congenital mitral regurgitation is more common than mitral stenosis and is usually associated with AVC defects. Cardiac neural crest cells are a subpopulation of the cranial neural crest and are crucial for normal cardiovascular development [29]. Cardiac neural crest cells contribute to the formation and remodeling of the aortic (pharyngeal) arch arteries, outflow tract septation, semilunar valvulogenesis, cap of the interventricular septum, parasympathetic innervation of the heart (neurons and supporting cells of cardiac ganglia), and cardiac conduction system [73, 74]. The outflow tract connects the primitive ventricle to the aortic arch arteries and is traditionally divided into three sections: the conus (proximal), the truncus (middle), and the aortic sac (distal) [75]. Septation of the outflow tract begins with the formation of a shelf of mesenchyme in the dorsal wall of the aortic sac in the area between the fourth and sixth pairs of aortic arch arteries [76]. This shelf, called the aorticopulmonary septum, elongates toward the truncus, thereby dividing the lumen of the aortic sac into the future intrapericardial portions of the aorta and pulmonary trunk. Cardiac neural crest cells delaminate from the cranial portion of the neural tube and migrate into pharyngeal arches 3, 4, and 6, and a subset of these cells migrates into the outflow tract (Figure 6.15). The outflow endocardial cushions are ridges of mesenchyme, formed by EMT of the underlying endocardium, that spiral into the outflow tract. Mesenchymal cells from the pharynx, followed by neural crest cells, invade the truncal cushions to form two centrally placed columns, or prongs, in the shape of an upside‐down “U” (Figure 6.16) [78]. These prongs will fuse with the aorticopulmonary septum distally and with the conal cushions proximally. Rotation of the developing pulmonary valve (see later in the chapter) is associated with spiraling of the aorticopulmonary septum and prongs, so that the developing aorta will connect with the rightward and cranial component of the aortic sac and the developing pulmonary trunk will connect with the leftward and caudal component of the aortic sac [79]. Figure 6.15 Cardiac neural crest. Migration of the cardiac neural crest to the circumpharyngeal ridge, caudal pharyngeal arches (3–6), and outflow tract depicted schematically. S1, S2, S3, S4, somites 1–4. Oto, otic vesicle; Ao, aorta; P, pulmonary artery. (Source: Kirby [23]. Reproduced with permission of Oxford University Press.) The convergence of the inflow and outflow poles during looping combined with the rotation and wedging processes brings the atria and outflow vessels into concordance with the AV valves and ventricles [75, 78]. In the conus, myocardial cells invade the cushions (myocardialization), which then bulge further into the lumen and subsequently meet and fuse in the midline. These conal cushions fuse most proximally with the AV cushion tissue and the crest of the muscular ventricular septum. Muscularization of the central conal cushion tissue and development of the adjacent aortic and pulmonary sinuses produce the subpulmonary infundibulum and a plane of space between the infundibulum and aortic root [79]. The developing pulmonary valve is initially posterior to and left of the developing aortic valve. Morphogenetic movement of the pulmonary valve (posterior to anterior, left of the aortic valve) brings it to its final position anterior and lateral to the aortic valve (Figure 6.9) [80]. This movement of the pulmonary valve is thought to be caused by the development of the subpulmonary conus (infundibulum), which also causes the pulmonary valve to be in a superior position relative to the aortic valve. Early in development, both the subpulmonary conus and the subaortic conus are situated above the right ventricle. Following torsion and a leftward shift of the outflow tract, resorption and shortening of the subaortic conus cause the aortic valve to sink inferiorly and posteriorly so that it comes to lie directly over the left ventricle and in fibrous continuity with the mitral valve (Figure 6.9) [81]. The conversion from the primitive, single in‐series circulation to the double in‐series circulation takes place during the fifth week of development. Following outflow tract septation, the semilunar valves are formed as the mesenchyme in the outflow cushions is remodeled into the tri‐leaflet aortic and pulmonary valves. Initially, three pairs of ridges, consisting of mesenchymal tissue covered by the endocardium, protrude into the lumen on each side of the divided truncus [78]. Valve sinuses are formed by excavation on the arterial (distal) face of the cusps. Thereafter, the leaflets are remodeled by mesenchymal apoptosis, blood flow, and shear stress to produce the delicate fibrous tissue seen in mature valves. Cells from the endocardium are the primary source of semilunar and AV valve fibroblasts, but fibroblasts derived from the epicardium also contribute to valve formation [76]. Cardiac neural crest cells are found at the tip of the leaflets and contribute to remodeling and maturation [74]. The role of cardiac neural crest in coronary vessel formation remains controversial. Figure 6.16 Schematic representation of the developing heart. Simplified model of a heart prior to ventricular septation. The roof of the atrium (A, light brown) is closed; therefore, atrial septation is not shown here. The anterior walls of the left ventricle (LV) and right ventricle (RV) are opened to reveal the inside. The interventricular foramen (primary foramen) is present. The inlet septum (IS) is connected to the inferior AV cushion (iAVC). The epicardium (olive green) covers most of the surface of the heart, but the venous proepicardial organ (vPEO; between liver and venous pole) and the arterial PEO (aPEO) surrounding the arterial pole are still present. The second heart field‐derived part of the RV is shown in yellow, the endocardial cushions in light blue, neural crest cells in lavender, and the gut (G), including the liver, in green. AVC, atrioventricular canal; M, mitral ostium; OFT, outflow tract; PV, pulmonary vein; sAVC, superior AV cushion; T, tricuspid ostium; VCI, inferior cardinal vein. (Source: Gittenberger‐de Groot et al. [77]. Reproduced with permission of Elsevier.) The loss or dysfunction of cardiac neural crest cells produces a wide spectrum of conotruncal and aortic arch abnormalities that are associated with syndromes linking defects in the heart with craniofacial and glandular (thyroid, parathyroid, and thymus) defects [74]. These include the 22q11 deletion syndromes, such as DiGeorge and velocardiofacial syndromes, CHARGE syndrome, fetal alcohol syndrome, retinoic acid embryopathy, Alagille syndrome, and Noonan and LEOPARD syndromes [75]. The association between thymus, thyroid and parathyroid abnormalities in these syndromes is explained by the fact that the neural crest cells contribute to the connective tissue of these three organs. Additionally, abnormalities of the SHF also produce outflow tract defects [78]. Persistent truncus arteriosus results from a failure in outflow tract septation (conotruncus and aorticopulmonary septum) combined with a virtual absence of the subpulmonary infundibulum and, thus, a VSD [69, 82]. Typically, the aortic and pulmonary valves are fused to form a single semilunar truncal valve. The origin of the pulmonary arteries from the truncal root varies and is dependent on the degree of the septation deficiency [83]. Incomplete outflow tract septation results in an aortopulmonary window. Aortoventricular tunnels are thought to result from abnormal excavation and maturation of the outflow cushions during the formation of the semilunar valves [79]. Unequal septation can result in either the aorta or pulmonary artery being hypoplastic relative to the other. Tetralogy of Fallot is thought to result from underdevelopment of the subpulmonary infundibulum (conus) [84]. The smaller infundibulum causes anterior deviation of the conal septum and malalignment of the conal septum with the muscular ventricular septum, producing right ventricular outflow tract obstruction and a subaortic VSD [85]. The pulmonary valve is usually abnormal, and the reduced blood flow contributes, to some degree, to pulmonary artery hypoplasia. Some patients have tetralogy of Fallot with pulmonary valve atresia (TOF/PA) and varying degrees of hypoplasia of the main and branch pulmonary arteries. Theories that have been proposed to explain TGA include resorption of the subpulmonary conus, causing the pulmonary valve to move inferiorly and posteriorly so that it comes to lie above the left ventricle and in fibrous continuity with the mitral and tricuspid valves [80], and failure of the aorticopulmonary septum to spiral [86]. Dextro‐ or D‐TGA refers to TGA with normal looping of the heart to the right, whereas L‐TGA refers to looping of the heart to the left. In D‐TGA, there is AV concordance and VA discordance. Congenitally or physiologically corrected TGA is characterized by TGA and AV discordance (i.e. double discordance). In the more frequent form, referred to as L‐transposition, there is levocardia with situs solitus of the atria and L‐looping of the heart. The other form is a mirror image of the above with dextrocardia, inversion of the atria (situs inversus), and D‐looping of the heart [87]. Persistence of both the subpulmonary and subaortic coni results in a double outlet right ventricle, whereas a deficiency of both results in a double outlet left ventricle (very rare); in either case, there is almost always a VSD. Fused or absent pulmonary commissures produce pulmonary valve stenosis, in which the valve is typically mobile and dome‐shaped with a small and sometimes eccentric orifice [88]. Critical pulmonary stenosis is a severe form of pulmonary stenosis in the neonate that is considered a ductal‐dependent lesion because a patent ductus arteriosus (PDA) is essential for pulmonary blood flow. Obstruction of the left ventricular outflow tract may occur at the valvular, subvalvular, or supravalvular levels. The most common aortic valve anomaly is a bicuspid aortic valve [2]. This malformation is also thought to be the most common cardiac defect (occurring in 1–2% of the population). It may be an incidental finding or may result in a clinical presentation in childhood or adult life, and it can occur in isolation or in association with left‐sided obstructive lesions [89]. There are several variants, the most frequent being characterized by commissural fusion (most commonly between the coronary cusps), creating a functionally bicuspid structure, and the far less frequent “true” bicuspid valve. Critical aortic valve stenosis in the neonate is a ductal‐dependent lesion in that a PDA is necessary for systemic perfusion. The valve is usually unicommisural or bicommisural and incompletely differentiated with thickened, redundant, and rolled cusps and leaflets consisting of immature loose connective tissue (myxomatous) [90]. Dysplastic valves, rather than commissural fusion, obstruct the orifice. Severe aortic stenosis or aortic atresia can be part of a spectrum of left‐sided obstructive lesions associated with hypoplasia of the left ventricle, ascending aorta and/or arch, mitral valve stenosis or atresia, and endocardial fibroelastosis of the left ventricle (i.e. HLHS) [64]. Subvalvular aortic stenosis has a number of embryologic etiologies. Malalignment of the conal septum with the muscular interventricular septum results in posterior projection of the conal septum into the left ventricular outflow tract and an associated VSD. This pathology may be seen within the context of an interrupted aortic arch. Familial hypertrophic cardiomyopathy is an autosomal dominant developmental abnormality most commonly due to mutations in the contractile protein (sarcomere) genes, but it may also be due to mutations in non‐sarcomeric genes that are associated with mitochondrial defects, potassium channel abnormalities, and abnormalities of protein kinase A [91]. A discrete fibromuscular ridge or membrane below the aortic valve can also cause subaortic stenosis. Congenital supravalvular aortic stenosis (SVAS) is caused by the deletion of or a loss‐of‐function mutation in the elastin gene on chromosome 7q11.23. In the majority of cases, SVAS occurs in association with Williams–Beuren syndrome, which is frequently referred to as Williams syndrome (WS) [92, 93]. In other cases, SVAS is inherited as an autosomal dominant disorder or results from sporadic mutations and occurs without mental retardation, elfin facies, distinctive behavioral traits, and neonatal hypocalcemia associated with WS [94]. During the early stages of development, the smooth muscle cells from patients with WS and non‐syndromic SVAS express only about 15 and 50%, respectively, of the normal levels of the elastin‐precursor tropoelastin [95]. The resultant arterial media has a reduced elastin content, pathologic alignment of the elastin fibers, an increased collagen content, and an increased number of hypertrophied smooth muscle cells. The entire ascending aorta may be narrowed (aortopathy) in about 30% of patients, and up to 80% of patients will have peripheral pulmonary artery stenosis [96]. Heritability and genetic etiologies of non‐syndromic left‐sided obstructive lesions are highly heritable. First‐degree relatives of an individual with a left‐sided obstructive lesion have a 20% chance of having CHD (most often a bicuspid aortic valve) [97]. The genetic causes of left‐sided obstructive lesions overlap due to genetic heterogeneity (Table 6.1, Figure 6.17) and help explain the phenotypic variability within families with the same genetic variant [98–100].
CHAPTER 6
Development of the Cardiovascular System
Introduction
Contemporary concepts of cardiac development
Cardiovascular development: normal and abnormal
Cardiogenic fields
Formation of the heart tube
Abnormalities of heart tube formation
Cardiac looping
Abnormalities of cardiac looping
Cardiac septation
Atrial septation
Defects in atrial septation
Ventricular septation
Defects in ventricular septation
Atrioventricular canal septation and AV valve development
Defects of the AVC
Outflow tract septation and semilunar valve development
Abnormalities of outflow tract septation
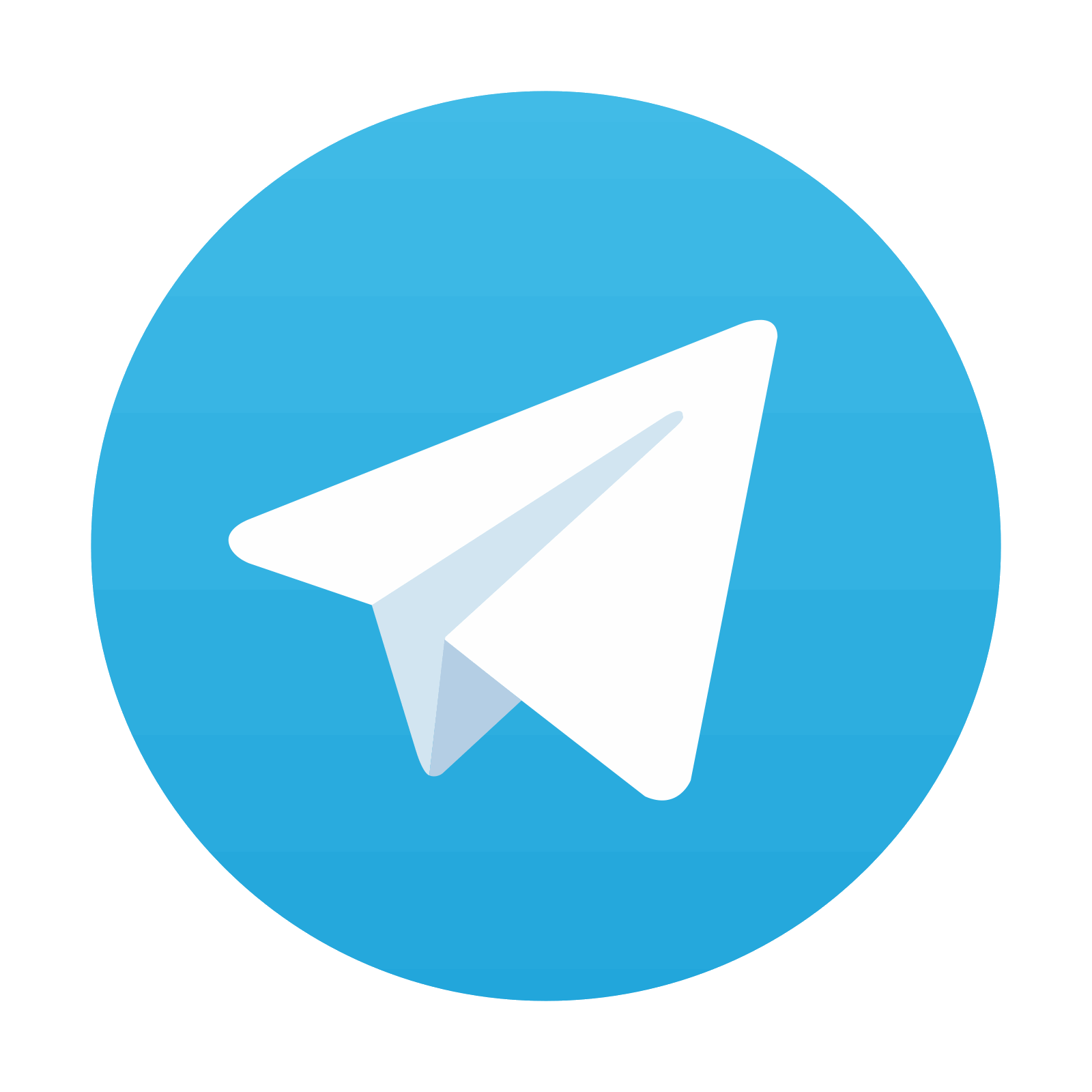
Stay updated, free articles. Join our Telegram channel
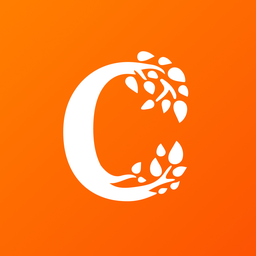
Full access? Get Clinical Tree
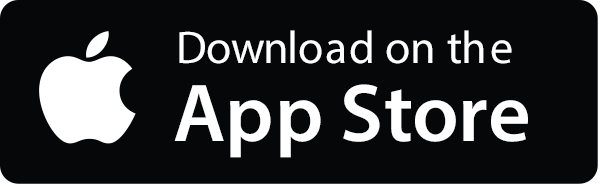
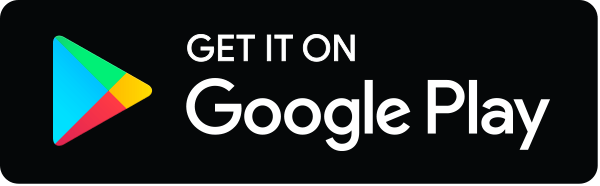