Glyn D. Williams1, Chandra Ramamoorthy1, Anshuman Sharma2, and Manchula Navratnam1 1 Professor of Anesthesiology, Perioperative and Pain Medicine, Department of Anesthesiology, Stanford University School of Medicine, Lucile Packard Children’s Hospital, Stanford, CA, USA 2 Department of Anesthesiology, Perioperative and Pain Medicine, University of California, San Francisco, CA, USA Following the pioneering adult and infant heart transplants of the late 1960s [1, 2], cardiac transplantation has evolved into a successful, standard therapy for end‐stage pediatric heart disease. Advances in immunosuppressant therapy, pretransplant recipient optimization, donor organ procurement/preservation techniques, and post‐transplant recipient management have all contributed to improved pediatric outcomes despite increased case complexity [3]. The Scientific Registry of the International Society for Heart and Lung Transplantation (ISHLT) is the main source of international multicenter data and captures approximately 75% of worldwide thoracic transplant activity [3]. Currently, approximately 500–600 pediatric heart transplants are performed annually across the globe, with 210 pediatric centers reporting data to ISHLT [3] (Figure 32.1). Most of the pediatric data are reported from centers in Europe and North America. Centers performing >10 transplants/year perform the majority (nearly 50%) of transplants [4] (Figure 32.2). Diagnostic indications for pediatric heart transplantation were updated in 2007 [5] and summarized in recent ISHLT annual reports [4, 6]. As post‐transplant survival rates and outcomes have improved, the dilemma is no longer whether to transplant, but rather when to do it [7]. Premature transplantation results in exposure of the recipient to the hazards of transplantation and immunosuppression. Excessive delay may result in death without transplantation or the development of high‐risk comorbidities, such as renal and liver dysfunction, malnutrition, and elevated pulmonary vascular resistance (PVR). Optimal timing for listing will vary depending on the primary diagnostic indication and anticipated disease progression. Primary diagnostic indications for heart transplantation are categorized into four main groups, with dilated cardiomyopathy (DCM) and congenital heart disease (CHD) accounting for the majority of pediatric heart transplants (Table 32.1) [6]. Indications for heart transplantation vary with age; the majority of transplants in infants are for CHD, whereas DCM is the leading cause in children >1 year of age [4, 8] (Figure 32.3). Indications for heart transplant also vary with geography (Figure 32.4), such that the primary diagnosis in Europe and other countries is DCM. CHD is a more common indication in North America than in Europe, accounting for 43% of heart transplants in North America versus 22% in Europe. Diagnostic indications for heart transplant are important since they are associated with differences in post‐transplant morbidity and mortality. For example, DCM confers a post‐transplant survival advantage compared with CHD across all age groups [4]. Figure 32.1 Number of pediatric heart transplants performed per year – age distribution by year of transplant. Note that this figure includes only the heart transplants that are reported to the International Society for Heart and Lung Transplantation Transplant Registry. (Source: Rossano et al. [4]. Reproduced with permission of Elsevier.) Figure 32.2 Pediatric heart transplants: distribution of heart transplants by center volume. (Source: Rossano et al. [4]. Reproduced with permission of Elsevier.) Mechanical circulatory support as a bridge to transplant is being increasingly used in children for all diagnostic indications. Approximately 30% of patients are currently bridged to transplant with a ventricular assist device (VAD) and approximately 3–5% with extracorporeal membrane oxygenation (ECMO) [4]. Table 32.1 Primary diagnostic indications for pediatric heart transplants Source: Rossano et al. [6]. Reproduced with permission of Elsevier. HLHS, hypoplastic left heart syndrome. Almost 40% of children presenting with symptomatic DCM receive a heart transplant or die within 2 years of diagnosis [8]. Over 50% of children ≥5 years of age with DCM are bridged to transplant with a VAD [4]. DCMs have a diverse spectrum of etiologies and phenotypic manifestations (Table 32.2). Pediatric myocarditis, a secondary cause of DCM, has a 50–80% chance of resolution within 2 years of diagnosis even when patients present with acute, severe symptomatology [9, 10]. CHD as a primary indication for heart transplant includes a diverse group of conditions and accounts for 20–60% of pediatric heart transplants depending on age and geographical location (Figures 32.3 and 32.4). With improved outcomes and widespread adoption of the neonatal stage I palliation, the number of neonates and infants with single ventricle physiology undergoing heart transplant as a primary therapeutic modality has decreased significantly. However, there is an increasing number of patients presenting later in life with failing single ventricle palliation and this subgroup constitutes at least two‐thirds of patients with CHD presenting for heart transplant [11, 12]. Failing Fontan patients are a growing cohort presenting for pediatric heart transplant and are discussed in more detail later. Figure 32.3 Pediatric heart transplants: recipient diagnosis by age, 2010–2018. (Source: Rossano et al. [4]. Reproduced with permission of Elsevier.) Figure 32.4 Pediatric heart transplants: diagnosis distribution by geography. (Source: Rossano et al. [4]. Reproduced with permission of Elsevier.) “Other indications” are primarily due to hypertrophic cardiomyopathies (HCM) and restrictive cardiomyopathies (RCM). HCMs account for 5–6% of pediatric heart transplants and include several genotypes and etiologies including idiopathic, familial inborn errors of metabolism, and malformation syndromes such Noonan’s and Beckwith–Wiedemann syndromes. [5]. HCM is a leading cause of sudden cardiac death in adolescents and young adults. A recent independently validated model for risk of sudden cardiac death in pediatric HCM identified a positive associated with nonsustained ventricular tachycardia and unexplained syncope and a nonlinear association with age at diagnosis, interventricular septal thickness z score, left ventricular (LV) posterior wall diameter z score, and left atrial diameter z score [13]. RCMs result in diastolic dysfunction usually with normal ventricular size and function and biatrial enlargement. One‐third of patients have a mixed phenotype of RCM and HCM [14]. RCMs are associated with myocardial infiltrative processes, such as amyloidosis, hemochromatosis, glycogen storage disease, mucopolysaccharidosis, sarcoidosis, and endomyocardial fibrosis. Syncope and ischemia indicate a poor prognosis, and elevated PVR is often present. Due to limited medical and surgical treatment options and the risk of irreversible pulmonary hypertension, thromboembolic disease, and sudden death many centers favor an approach of early listing for heart transplant [5]. Retransplantation accounts for a small percentage (5–7%) of pediatric heart transplants and is more common in North America (6%) than Europe (2%) [4, 15]. Coronary allograft vasculopathy (CAV), the most common indication for retransplantation, is discussed in more detail later. Other indications include acute or chronic rejection and primary graft failure (Table 32.3). Data indicate that retransplantation for early primary graft failure has a worse prognosis than for late graft failure or CAV [16–18]. The best outcomes are associated with those retransplanted for CAV [15, 18]. Children with graft failure due to acute rejection with hemodynamic compromise, especially <6 months post‐transplant, are regarded as inappropriate candidates for retransplantation [18]. The majority (65%) of pediatric retransplants occur at least 3 years after the primary transplant [4, 15]. Retransplantation has a similar 1‐year survival to primary transplants but is associated with decreased long‐term survival and increased transplant‐related morbidities such as renal dysfunction, late rejection, and CAV [4, 15, 18]. Table 32.2 Causes of dilated cardiomyopathy Source: Lipshultz et al. [8]. Reproduced with permission of Wolters Kluwer Health, Inc. DCM, dilated cardiomyopathy; GSD, glycogen storage disease. Table 32.3 Indications for cardiac retransplantation Source: Mahle et al. [17]. Reproduced with permission of Elsevier. Infants listed for heart transplant have the longest waitlist time and a higher waitlist mortality when compared with older children. Infant waitlist mortality in the United States is 25% compared with 15% for children and adolescents [19, 20]. An increasing number of centers are adopting ABO‐incompatible (ABO‐I) heart transplants as a standard practice to reduce waitlist mortality in this vulnerable population. Historically donor and recipients are matched for ABO compatibility. ABO‐I heart transplants have been avoided because of the risk of hyperacute rejection that can occur when recipient isohemagglutinins (serum anti‐A or anti‐B antibodies) bind to the corresponding blood group antigens on donor organ vascular endothelium. Organ allocation across blood group barriers increases the donor organ pool available for heart transplantation. Since blood group O‐type recipients have both anti‐A and anti‐B antibodies (isohemagglutinins), only an O‐type donor is compatible and hence they have the longest waitlist time. Isohemagglutinins are not present at birth, and serum antibody titers remain low until 12–14 months of age [21]. Young infants also have an immature complement system. Therefore, the mechanisms leading to hyperacute rejection in ABO‐I heart transplant are absent during infancy. This discovery was pioneered in Toronto in 1996 when the first ABO‐I heart transplant was performed [22]. With increasing multicenter experience and data, current United Network for Organ Sharing (UNOS) guidelines recommend ABO‐I heart transplant in children under 1 year of age with any isohemagglutinin titer and in 1–2 years with titers less than 1 : 16. In 2016, UNOS also changed its allocation criteria so that patients listed as ABO‐I have equal organ priority to ABO‐compatible (ABO‐C) heart transplants. This has led to an increased number of patients listed and receiving ABO‐I heart transplants. Various registry and multicenter data have shown comparable survival and outcomes for ABO‐I heart transplants compared with ABO‐C heart transplants in infants <1 year and no difference in rates of rejection 1‐year post‐transplant [23]. There is consistent data that ABO‐I heart transplants decrease waitlist time, especially in patients with blood group O [23, 24]. A detailed assessment of patients is required to determine their suitability for heart transplantation (Box 32.1). Factors that must be considered include severe central nervous system, liver or kidney dysfunction, pulmonary infarction, pulmonary hypertension, morbid obesity, and some infections, malignancies, or chromosomal abnormalities. Prediction of mortality after transplantation was more accurate when multiple high‐risk criteria were included in the assessment of potential recipients [25]. Transplant centers usually have formal meetings to present a structured multidisciplinary evaluation of recipients prior to accepting for heart transplant listing. At Stanford Children’s Hospital, a cardiac anesthesiologist is present at these meetings. Evaluation revolves around four key areas [5]: Assessment of cardiopulmonary function usually includes cardiac catheterization and magnetic resonance imaging (MRI) or computed tomography (CT) to delineate the vascular connections, particularly in patients previously palliated for complex heart disease. Abnormal cardiovascular anatomy influences surgical technique during harvesting and transplantation. For example, a patient with palliated hypoplastic left heart syndrome and residual arch obstruction may require extra donor arch material during donor harvesting, or a Fontan patient with branch proximal pulmonary artery (PA) stenosis may need PA reconstruction during transplantation. Identifying anomalous systemic or pulmonary venous return, PA malformations, and accessory collateral pulmonary blood flow is also important for the surgical procedure [5]. Quantifying PVR prior to listing is important because PVR > 6 Wood Units/m2 or a transpulmonary gradient >15 mmHg is associated with acute right heart failure and increased mortality [26, 27]. The upper limit of PVR associated with successful cardiac transplantation has not been established in children. All patients with pulmonary hypertension have PVR measured at baseline conditions and during administration of oxygen, nitric oxide (NO), and/or other pulmonary vasodilator therapy. When the response is marginal, repeat values after 1–2 weeks of inotropic support, afterload reduction, and pulmonary vasodilation may demonstrate improvement. Experienced institutions may accept children with PVR as high as 8–12 Wood Units/m2 if it is reactive. Patients whose PVR does not respond to therapy may be the candidates for heart–lung transplantation (HLT) or lung transplantation. Children with RCM are particularly prone to marked elevation of PVR, which may contribute to the poor prognosis in these patients and make cardiac transplantation problematic. Nitric oxide appears to be a good agent to demonstrate reversibility of PVR in these patients [26]. PVR also improves after left ventricular assist device (LVAD) implant, especially for patients with DCM and left atrial hypertension [28]. Endomyocardial biopsy may be required prior to listing for dilated cardiomyopathies to identify acute myocarditis and myocardial infiltrates. A diagnosis of acute myocarditis is important in determining suitability for transplant listing since there is chance of resolution as mentioned earlier [9, 10]. Pretransplant end‐organ dysfunction and chronic noncardiac disease have an impact on post‐transplant morbidity and mortality. Assessing the degree of pretransplant renal dysfunction is important because reduced estimated glomerular filtration rate (eGFR) and pretransplant renal dialysis are associated with increased 1‐, 5‐, and 10‐year mortality [4, 29]. Some centers will treat moderate‐severe renal dysfunction with a combined heart kidney transplant. Assessing liver function is also important especially in failing Fontan patients. Elevated serum bilirubin is a continuous risk factor for 1‐year mortality post‐transplant [12, 29]. There is an increasing trend of treating irreversible liver disease with a combined heart–liver transplant (CHLT) and is discussed in more detail later. Assessing the degree of chronic lung disease especially in older children with CHD is important and will include pulmonology assessment and pulmonary function tests. Evaluation of patients with cardiomyopathy should include a metabolic workup to detect potential etiologies such as mitochondrial disorders and genetic studies. Transplant teams are also intensifying attention on the pretransplant nutritional status of the recipient since this may have a significant impact on postoperative hemostasis, wound healing, and recovery. Infectious disease and immune system evaluation are essential. The child’s immunization status should be updated if necessary. Tests are performed for latent infections, such as cytomegalovirus or Epstein–Barr virus, that may become clinically significant during immunosuppression. Donor matching is based on ABO typing. The candidate’s blood is also screened for antibodies against sera of random blood donors and, if reactive, a serum crossmatch with the donor is performed. There is an increased risk for graft dysfunction, acute cellular and antibody‐mediated allograft rejection, and chronic rejection/CAV after heart transplantation in patients sensitized to HLAs. The number of heart transplant candidates allosensitized to HLA antigens has increased in recent years because of exposure to blood products, homograft material used in surgical palliation of CHD, use of ventricular assist and mechanical support devices, and patients requiring retransplantation. Determinations of panel‐reactive antibody (PRA) are done to delineate a patient’s potential for sensitization to donor HLA antigens. Patients with a reaction to >10% of antigens (either class I or II) are generally considered to be allosensitized. However, this is allosensitivity to a “random” donor; having a positive crossmatch with the actual donor (HLA antibody toward donor alloantigens) at the time of transplant has been clearly demonstrated to increase the risk for poor outcome after transplant. Donor‐directed HLA antibodies are associated with rejection‐related mortality from cellular and antibody‐mediated acute rejection and CAV. Patients with PRA >10% pretransplant and a positive crossmatch are at high risk for graft loss from hyperacute rejection and early acute cellular rejection (ACR), which in turn increase the risk of CAV. Allosensitized patients may be excluded from heart transplant, restricted to certain donors, or experience prolonged waiting times. Some transplant programs require a prospective negative donor‐specific crossmatch for patients with PRA screens >10%. However, this approach is problematic because of the donor shortage and some patients have antibodies to many HLAs. Therefore, perioperative management protocols for HLA‐sensitized children have been developed and include pretreatment of sensitized patients (using treatments such as intravenous immune globulin, cyclophosphamide, mycophenolate mofetil [MMF], and rituximab), intraoperative plasma exchange, post‐transplant plasmapheresis, and T‐ and B‐cell suppression [30–34]. Heart transplantation requires long‐term immunosuppression, frequent invasive procedures, and lifelong medical care. Patients must live close to the hospital during the initial months after surgery, and temporary relocation of the family may be necessary. Prolonged periods of stressful hospitalization are likely. A stable social situation is essential for success, and psychosocial evaluation is an important aspect of the pretransplantation process. Organ transplantation in the United States is sanctioned by congressional mandate through the National Organ Transplant Act (NOTA). An Organ Procurement and Transplant Network (OPTN) was created and is administered by the UNOS. UNOS has three recipient status categories for patients listed for heart transplantation: status IA, status IB, and status II, with status IA indicating the sickest patients who are in urgent need of transplantation for survival. Status seven patients are those previously listed as status IA, status IB, or status II, but considered temporarily unsuitable to receive a heart transplant, e.g. due to active infection. Current waitlist mortality ranges from 17 to 30% depending on country and institution. Waitlist mortality also varies with transplant center volume, with a reported 5% mortality in large volume centers compared to 30% in small volume centers [35]. Waitlist mortality is also influenced by patient factors such as age, CHD, weight <3 kg, status 1A, the need for ECMO, and renal dialysis [36, 37]. Increased adoption of VADs and improved pretransplant recipient support has helped to decrease waitlist mortality over time [38]. The median waiting period from heart transplant listing to actual surgery currently varies between 60 and 80 days, depending on the child’s age, blood group, and list status [39]. Aggressive medical management to achieve stabilization is required and includes supplemental oxygen, diuretics, inotropic support (e.g., dobutamine, dopamine, phosphodiesterase inhibitors), antiarrhythmia therapy, and mechanical ventilation. Children with chronic heart failure often receive digoxin, diuretics including spironolactone, angiotensin‐converting enzyme inhibitors, and β‐blockade therapy (e.g., metoprolol, carvedilol). Patients with severe LV dilation may need anticoagulation, usually coumadin or heparin, to prevent the development of intracardiac thrombi and systemic embolization. Amiodarone is often chosen for treating arrhythmia. Implantable transvenous defibrillators and, more recently, transcutaneous defibrillators have been effective in pediatric patients large enough for these devices. For patients unsuitable for implantable defibrillators either due to patient anatomy or physiology, Life Vest® (Zoll Medical Corp., Pittsburgh, PA, USA) wearable external cardioverter‐defibrillator (ICD) therapy can be a short‐term option to enable home discharge while waiting for transplant. Biventricular pacing is an emerging modality to improve myocardial function. Patients with refractory myocardial failure require mechanical circulatory support as a bridge to cardiac transplantation. Approximately one‐third of patients with LVADs have right ventricular dysfunction. Medical support and protection of the right ventricle should be considered during anesthesia induction, maintenance, and surgical dissection. Perioperative management algorithms for VAD‐supported patients are delineated in a recent comprehensive review [40]. Extracorporeal life support and other mechanical devices as a bridge to transplant are discussed in Chapters 36 and 37. Pediatric transplant centers are developing pretransplant management strategies that target modifiable risk factors to reduce post‐transplant morbidity and mortality [41]. For example, at Stanford Children’s Hospital, identification of perioperative acute kidney injury (AKI) as a risk factor for post‐transplant morbidity and mortality especially in the CHD population led to the development of a perioperative renal‐protective protocol. In the United States and many other countries, solid organs can be obtained for transplantation by donation after brain death (DBD) or by donation after cardiac death (DCD) [42]. DBD criteria were first established in 1968; prior to this, organs were obtained from DCD, including the first heart transplant. Although DBD soon became the standard for organ procurement, scarcity of available organs led to a reexamination of DCD. During DCD, the goal is to initiate organ procurement as soon as possible after ensuring that death from cardiorespiratory arrest has truly occurred, because viability of the donor organ becomes compromised when the warm ischemic time is prolonged. A North American multidisciplinary panel recently concluded that “Death can be declared after the cessation of circulation and respiratory function for 2 minutes” [43]. Despite DCD being legal and with recipient outcomes that are favorable, ethical issues still surround DCD, and DBD currently remains the primary source of organs [44]. The age distribution of pediatric heart donors is similar to that of heart recipients [3]. Echocardiography is useful for assessment of donor heart function. Widespread malignancy or infection in the prospective donor is an exclusion criterion, but cardiac resuscitation and chest trauma are not contraindications, provided the donor’s hemodynamics have been stabilized and inotropic agents are no longer needed or are at minimal doses. Assessing potential donor organs involves evaluating immunocompatability, donor risk factors, travel time, recipient pulmonary hypertension, and donor–recipient size match. In contrast to adult heart transplant, sex mismatch has not been found to significantly affect outcomes [45]. Attempts to limit donor heart ischemia time are important but may be hampered by transport issues. Donor (allograft) ischemic time is defined as time between donor aortic cross‐clamp during organ procurement surgery and donor coronary artery reperfusion during heart transplant surgery [46]. The median donor ischemic time for the current era is 3.5–3.7 hours [46]. Donor ischemic time >4 hours is associated with increased 30‐day, 1‐year, and 5‐year mortality [3, 46]. Conversely, donor ischemic time has not been shown to be associated with major post‐transplant morbidity such as CAV or renal dysfunction. Some centers may opt to accept longer donor ischemic times for patients with a high risk of waitlist mortality. At Stanford children’s Hospital, our standard strategy is to reperfuse the donor heart for a minimum of one‐third the ischemic time prior to cardiopulmonary bypass (CPB) separation. The anesthetic management of a pediatric organ donor is beyond the scope of this chapter [47, 48]. Donor–recipient size matching has important consequences for both organ allocation and post‐transplant outcomes for pediatric heart transplantation. Undersized cardiac allografts have the risk of not being able to provide sufficient cardiac output (CO), and oversized allografts confer a risk of compressing neighboring structures such as lungs and subsequent physiological effects [49]. Recent publications have also questioned the approach of using oversized donors for patients with pulmonary hypertension [50]. Approximately 20% of potential pediatric donor allografts without marginal/high‐risk characteristics are not used [51]. Innovative techniques to improve donor organ utilization have the potential to reduce pediatric waitlist mortality. Historically, standard clinical practice has been to use donor–recipient body weight ratio (DRBW) and donor–recipient height ratios for allograft size matching. At the time of listing transplant, institutions will define a lower and upper weight limits that they are willing to accept for their patient. A typical range would be 80–160% of recipient body weight. Estimating size match eligibility using traditional measurements such as weight and height assumes a predictable correlation with total cardiac volume. Total cardiac volume, however, may not always correlate with somatic growth. For example, children with advanced heart failure may have a lower than predicted body weight because of failure to thrive or a higher body weight due to fluid overload. Body weight is also an inaccurate correlate of heart size in patients with CHD or RCM where the heart size is often smaller and in patients with dilated cardiomyopathies and cardiomegaly. Heart size matching based on weight also does not account for the complexities of implantation in patients with heterotaxy and cardiac malposition. Waitlist mortality and allograft shortages have led clinical transplant programs to widen their acceptable DRBW to increase the donor pool. Innovative approaches to performing virtual heart transplant fit assessments include using allograft total cardiac volume prediction models and advanced donor imaging such as CT and MRI for a direct virtual transplant assessment [52–54]. Adoption, development, and standardization of these alternative approaches may improve organ utilization. Figure 32.5 “Bicaval” cardiac transplantation. (A) The recipient cardiactomy has been completed. Note the entire recipient right atrium has been removed. (B) The completed implant. The sequence of anastomoses is (i) left atrial, (ii) aortic (cross‐clamp removed and heart perfused), (iii) inferior vena cava, (iv) pulmonary artery, and (v) superior vena cava. (Source: Backer et al [56]. Reproduced with permission of Taylor & Francis.) Orthotopic heart transplants whereby the recipient heart is excised and replaced in the correct anatomical position by the donor heart is the standard surgical approach. The biatrial anastomoses originally described by Lower and Shumway [55] has now been abandoned in most centers and replaced by the bicaval left atrial cuff technique – the current standard approach for pediatric heart transplant. A small cuff of recipient left atrial tissue is left in place, incorporating all pulmonary veins, and the entire right atrium of the recipient is removed. Standard implantation of the donor heart involves five anastomoses – left atrial cuff, aorta, inferior vena cava (IVC), superior vena cava (SVC) (bicaval anastomoses), and main PA. Surgeons will vary with regard to the order of anastomoses. Two golden rules that apply for all implantations are that the left atrial anastomosis is performed first, and once the left atrial anastomoses and aortic anastomoses are completed, the aortic cross‐clamp can be removed and donor heart reperfusion commenced (Figure 32.5) [57]. A meta‐analysis found superiority of the bicaval technique in comparison with the biatrial procedure for early atrial pressure, perioperative mortality, tricuspid valve regurgitation, and sinus rhythm [58], although others found no difference in long‐term survival [59]. Cardiac transplantation for children with CHD can involve more complex anastomoses and vascular reconstruction such as branch PA reconstruction and aortic arch reconstructions for recipients with palliated single ventricle physiology. Deep hypothermic circulatory arrest may be employed in patients requiring extensive vascular reconstruction. Children listed for heart transplantation have limited physiological reserves. Even minor changes induced by anesthesia, and surgery can trigger cardiovascular instability and decompensation. Prior to surgery, young infants and children with decompensated heart failure may already be in intensive care with invasive lines and inotropic and respiratory support. More stable patients may be admitted from home at the time of donor acceptance and could have eaten recently. Several hours usually elapse before surgery, but therapy to modify gastric pH and volume and the application of continuous cricoid pressure during induction might be required. Up to 30% of pediatric heart transplants are bridged with a VAD and may be either inpatient or outpatient and will be on some form of anticoagulation and antiplatelet therapy [60]. Communication between the transplant surgeons, anesthesiologists, operating room staff, and donor procurement team is vital to coordinating care and ensuring allograft ischemia time is minimized. At Stanford Children’s Hospital, we hold a preoperative “huddle” attended by the heart failure and transplant teams, intensive care team, the surgeon, anesthesiologists, and perfusionists. At this meeting, donor‐ and recipient‐specific issues are addressed, including allosensitization and plasmapheresis, vascular access, invasive monitoring lines, emergency cannulation techniques, immunosuppressants, including steroids, and the need for urgent reversal of anticoagulation such as warfarin reversal with a prothrombin complex concentrate [60]. We also discuss renal risk stratification and employ renal‐protective strategies for patients with a moderate‐high risk of postoperative AKI. Stanford Children’s renal‐protective protocols involve risk stratification of patients for risk of AKI, maintaining adequate renal perfusion through blood pressure management, fluid management, and reducing central venous congestion, pharmacoprophylaxis with pre‐, intra‐, and postoperative aminophylline and a modified induction protocol to avoid highly nephrotoxic medications in the immediate postoperative period. As part of the huddle, we discuss a patient‐specific “flight plan,” which addresses all relevant patient specific perioperative issues and is documented in the electronic medical record (Figure 32.6). Premedication and the method of anesthesia induction depend upon the patient’s age, cardiac lesion, and cardiopulmonary function. Establishing invasive hemodynamic monitoring prior to induction of anesthesia may not always be feasible and so it is imperative to institute noninvasive patient monitoring prior to the administration of medications that alter hemodynamic and/or respiratory function. Focused transthoracic echocardiography (TTE) may have a role in patients where preinduction arterial lines may not be an option. Qualitative assessment of cardiac function in patients with severely reduced function and septal configuration in patients with pulmonary hypertension can be monitored with a parasternal short‐axis view. Anesthesia‐ or surgery‐induced changes in heart rate (HR), preload, afterload, or contractility can precipitate hemodynamic decompensation. Meticulous airway management is vital as hypoxia and hypercarbia aggravate PVR and can further depress CO and high intrathoracic pressures may trigger right ventricular decompensation. Rapid sequence induction may be poorly tolerated in patients with minimal cardiorespiratory reserve. Significant ascites in patients with hepatic dysfunction (e.g. failing Fontan) may necessitate controlled peritoneal drainage peri‐induction. A wide variety of anesthetic agents have been used successfully. The desirable and detrimental cardiovascular effects of anesthetic agents are reviewed in Chapter 10. In children with CHD, a fentanyl/midazolam/muscle relaxant anesthetic technique was reported to preserve cardiac index better than volatile agents, provided HR was maintained [61]. Etomidate has minimal effect on hemodynamics and is a useful induction agent in patients with limited cardiovascular reserve. Propofol decreases systemic vascular resistance and reduces preload to the heart, and a bolus should be used with caution if at all. Nitrous oxide has myocardial depressant and pulmonary vasoconstrictor properties and is best avoided. Ketamine supports the circulation by indirectly stimulating catecholamine release. This may be blunted in children with DCM and impaired β‐agonist responses, or in patients who are on chronic infusions of β‐adrenergic agonists. When the patient’s endogenous catecholamine stores are depleted, the drug’s direct myocardial depressant effects may then predominate. Regardless of the agent used, hypotension must be anticipated and treated promptly to preserve coronary perfusion. Commencing a low‐dose inotrope infusion (e.g. epinephrine 0.01–0.03 mcg/kg/min) prior to induction of general anesthesia provided there are no significant arrhythmias may help avoid hypotension and preserve coronary perfusion. Recent excellent reviews describe the anesthetic management of children in the operating room [62, 63]. A trigger‐free anesthetic should be considered for patients with neuromuscular disease such as Duchenne or Becker muscular dystrophy to reduce the risk of malignant hyperthermia like symptoms or anesthesia‐induced rhabdomyolysis. Monitoring during transplantation surgery does not differ from that used for pediatric open‐heart surgery. Large‐bore vascular access and a rapid infuser device should be available for patients undergoing repeat sternotomy and those on preoperative mechanical circulatory support. Patients with pacemakers or ICDs should have their devices reprogrammed prior to the use of surgical diathermy. TEE is useful for evaluation of intracardiac air, transplanted heart anatomy and function, vascular anastomoses, mural thrombus, and early post‐transplant cardiac function. When PA pressure monitoring is indicated, some institutions prefer to place a catheter directly into the PA rather than use a percutaneous balloon‐tipped PA catheter. Due to the increased risk of perioperative bleeding in patients with CHD, repeat sternotomy and those on mechanical circulatory support prophylactic anti‐fibrinolytics are a useful adjunct. The management on CPB is similar to that in children undergoing cardiac surgery. Ultrafiltration during CPB may benefit the patient by removing excess free water, hemoconcentrating red cells and coagulation factors, and modulating the inflammatory response. Variations in ultrafiltration techniques are institution dependent. Issues of concern include denervated donor heart, global ischemia–reperfusion injury, elevated PVR, right ventricular dysfunction, arrhythmia, hemostasis, and hyperacute rejection. The transplanted heart is functionally denervated and may have an abnormal response to sympathetic stimulation. Resting HR may be higher than normal because of absent vagal tone. The normal beat‐to‐beat variations in response to respiration are lost, as are the normal responses of the heart to alterations in body position and carotid body massage. The donor heart cannot abruptly increase HR and CO in response to stress because the baroreceptor reflex is disrupted, and patients are dependent on circulating catecholamines. With the loss of the baroreceptor reflex, the patient with the denervated heart may initially show an exaggerated response to hypovolemia with a marked decrease in mean blood pressure and then a delayed exaggerated hypertensive and tachycardia response, due to endogenous catecholamine release. The Frank–Starling (pressure–volume) relationship remains intact and compensates for hypovolemia and hypotension by increasing stroke volume secondary to an increased venous return. Therefore, it is important to maintain adequate preload, especially if vasodilators are administered. Innervation of the peripheral vasculature is preserved, and changes in peripheral vascular resistance may still occur in response to alterations in sympathetic outflow from the vasomotor center due to signals from stretch receptors in the great vessels. Figure 32.6 Example of Stanford Children’s Hospital pediatric cardiac transplant flight plan. MRN, medical record number; DOB, date of birth; VAD, ventricular assist device; PH, pulmonary hypertension; PVR, pulmonary vascular resistance; NJ, nasojejunal; PLE, protein‐losing enteropathy; F, female; DCM, dilated cardiomyopathy; BIVAD, biventricular assist device; RVAD, right ventricular assist device; LVAD, left ventricular assist device; RA, right atrial pressure; RV, right ventricular pressure; PA, pulmonary artery; PCWP, pulmonary capillary wedge pressure; CI, cardiac index; PVRi, PVR indexed to body mass index; SVRi, systemic vascular resistance indexed to body mass index; WU, Woods Units, UE, upper extremity; LE lower extremity; US, ultrasound; CT, computed tomography; Abd, abdomen; RIJV, right internal jugular vein; RSCV, right subclavian vein; LIJ, left internal jugular vein; LSCV, left subclavian vein; RFV, right femoral vein; RFA, right femoral artery; LFV, left femoral vein; LFA, left femoral artery; CVL, central venous line; PIV, peripheral intravenous line; RA, right atrial; LA left atrial; AKI, acute kidney injury; CVP, central venous pressure; NINJA, nephrotoxic injury negated by just‐in‐time action; PD, peritoneal dialysis; ATG, antithymocyte globulin; CD3, CD3 T cells; IVIG, intravenous immunoglobulin; CMV, cytomegalovirus; PCR, polymerase chain reaction; EBV, Epstein–Barr virus; NSVT, nonsustained ventricular tachycardia; EAT, ectopic atrial tachycardia; PVC, premature ventricular contractions; Coreg; carvedilol; ICD, implantable cardioverter‐defibrillator. Drugs such as atropine, glycopyrrolate, neostigmine, and pancuronium that act on the heart through vagal or sympathetic neuromechanisms usually will not affect HR. However, there are case reports of profound bradycardia and hypotension following glycopyrrolate–neostigmine administration [64, 65]. α‐ and β‐Adrenergic receptors remain intact, and inotropes such as epinephrine and isoproterenol will cause appropriate responses from the heart. The donor organ is subjected to ischemia–reperfusion injury, and patients usually require inotropic support for separation from CPB. LV diastolic dysfunction is common and characterized by a restrictive ventricular filling pattern, with a reduced preload reserve and a relatively fixed stroke volume. Sinoatrial node dysfunction is relatively common. Dopamine, epinephrine, or isoproterenol is often selected, and epicardial temporary pacing can be instituted if necessary to achieve the desired HR. Arrhythmias are quite common in the early postoperative period, usually premature atrial or premature ventricular contractions. Compression of intrathoracic structures may be problematic during closure of sternotomy, particularly if the donor heart is relatively oversized [66]. It is important to preserve donor right ventricular function by keeping PVR low normal. Catecholamine release is reduced by ensuring the patient remains adequately anesthetized. Ventilation is facilitated by muscle relaxants, and normocarbia is maintained. Elevations in central venous pressure with low or normal left atrial pressure and reduced mean arterial pressure might be related to right ventricular failure. Elevated PA pressures can be detected by echocardiography and measured invasively. Additional measures to control PVR may be necessary, including pulmonary vasodilator therapy such as NO [67], prostacyclin, phosphodiesterase inhibitors, and isoproterenol. At Stanford Children’s Hospital, we routinely separate from CPB with inhaled nitric oxide (iNO) 20 ppm, dopamine, epinephrine, and milrinone infusions following pediatric heart transplants. More extreme hypocapnia may help, but also causes cerebral vasoconstriction and leftward shift of the oxygen dissociation curve. Cerebral oximetry monitoring using near‐infrared spectroscopy is helpful. If the patient’s CO remains inadequate despite maximal drug therapy, mechanical right ventricular assist or ECMO may be employed [68]. Blood loss during heart transplantation can be considerable and is associated with increased morbidity and mortality. Blood products are cytomegalovirus‐matched, leukoreduced, and irradiated, but coagulation management is no different from that for other open‐heart surgeries in children (see Chapter 16). For infants, some centers wash packed red blood cells to reduce the potassium load. Citrate‐induced hypocalcemia impairs contractility and coagulation; this may be minimized by initiating a calcium infusion (calcium chloride 10–30 mg/kg/hour). Rapid platelet transfusion may aggravate PVR. Patients on preoperative mechanical circulatory support often have an acquired von Willebrand deficiency, and cryoprecipitate can be a useful adjunct to help achieve hemostasis [69]. A multimodal approach to hemostasis management should be employed and there is an increasing trend for centers to use prothrombin complex concentrates to achieve perioperative hemostasis [60, 70]. Intraoperative immunosuppression regimens are institution specific. At Stanford, this is discussed during the preprocedural huddle and the medications are ordered by the transplant team to arrive in the operating room with the patient. In North America, a growing cohort of pediatric heart transplants are for failing single ventricle palliation. Failing single ventricle physiology accounted for 36% of CHD transplants in the early era (1990–2002) compared to 67% in the more recent era (2006–2017) [11, 12]. Most of the single ventricle transplants are for failing Fontans, followed by the failing Glenn stage [71]. Transplantation after stage 1 palliation is relatively uncommon [71]. Failing Fontan patients may be listed for heart transplant for several different reasons, including ventricular systolic or diastolic dysfunction, atrioventricular valve regurgitation, atrial or ventricular arrhythmias, high Fontan pressures and venous congestion, lymphatic failure such as plastic bronchitis or protein‐losing enteropathy (PLE), and hepatic or renal dysfunction. Because of this heterogeneous and often‐slowly progressive nature of Fontan failure, there are no clear consensus guidelines for optimal timing for heart transplant listing. Fontan heart transplants represent a high‐risk cohort with increased CPB times, increased donor ischemic times, and increased bleeding risk [71, 72]. Early era multicenter registry studies and single‐center studies reported that Fontan patients had decreased early survival after heart transplant when compared with other forms of CHD and cardiomyopathies. In the current era, expected survival after heart transplant for Fontan patients is comparable to other CHD patients [41]. This is a reflection in improved overall survival for pediatric heart transplantation despite increased case complexity [15, 71]. Improvements in perioperative care, recognition, and mitigation of modifiable risk factors have helped with improved outcomes. Institutions have developed their own multifaceted approach to deal with issues specific to this population [41]. Issues include complex surgical dissection and implantation, longer allograft ischemic times in Fontans (>4 hours), vasoplegia, aggressively treating for and monitoring primary graft failure, AKI, and the impact of PLE [41]. The association between the Fontan circulation and renal dysfunction, driven by renal venous congestion and low CO, has led to increased efforts to target the prevention of perioperative AKI in this population [73]. Data also suggest there may be an opportunity to improve outcomes by optimizing nutrition and reducing malnutrition in Fontan patients [74, 75]. Figure 32.7 illustrates a single‐center checklist to guide perioperative management for Fontan heart or CHLTs. Pediatric patients with relatively simple cardiac defects can successfully undergo liver transplantation with equivalent outcomes reported for children with and without CHD [76, 77]. The consensus remains that severe cardiac defects should be corrected prior to liver transplantation to avoid unacceptable transplantation mortality. Lesions of concern include significant cardiopulmonary disease, pulmonary hypertension [78], hypoxemia (PaO2 < 50 mmHg) − either from cardiac shunt or hepatopulmonary syndrome – elevated central venous pressure (>15 mmHg), and low CO. Successful liver transplantation outcome after bidirectional Glenn palliation for HLHS has been reported [79]. Although the first pediatric CHLT was reported in 1984, this remains relatively uncommon in children [80]. UNOS database shows that only 21 out of 387 heart liver transplants reported to date (2021) were in patients under 18 years of age. Elevated central venous pressure, hepatic congestion, and reduced CO in the Fontan circulation lead to the development of Fontan‐associated liver disease (FALD). All patients develop some degree of FALD after a Fontan, but it is unclear which ones will develop clinically significant liver disease. Studies have shown that the severity of FALD‐related liver histopathology most strongly correlates with overall time since Fontan completion [81]. Almost 100% of Fontan patients have histological evidence of fibrosis by the time they reach adolescence and 40% develop bridging fibrosis at 10 years post Fontan [81]. FALD ranges from bridging liver fibrosis to cirrhosis, portal hypertension, and, rarely, hepatocellular carcinoma [82]. Variceal‐related gastrointestinal bleeding and liver synthetic function derangements remain uncommon. Improved awareness and surveillance for FALD have led to an increasing number of patients being listed for CHLT. Currently, there are no consensus guidelines for diagnosing and monitoring FALD and which patients to list for heart–liver versus heart transplant. A recent study suggested that cirrhosis on imaging should prompt consideration for CHLT rather than heart transplant alone [82]. A multidisciplinary group recently proposed an algorithm of who to list for heart transplant versus heart–liver transplant (Figure 32.8). Some centers have adopted surveillance cardiac catheterization combined with transjugular liver biopsy in all patients 10–15 years after Fontan completion. Liver fibrosis seen in these patients are heterogeneous and, therefore, biopsy sampling error may underestimate the degree of liver involvement. Despite the difficulties with deciding who to list for heart–liver transplant, studies have shown that CHLT patients have 1‐ and 5‐year survival rates similar to heart transplant alone [70, 83]. Although the etiology is unknown, CHLT patients appear less vulnerable to acute cellular‐ and antibody‐mediated rejection (AMR) [83]. The adult transplant literature indicates that CHLT attenuates cardiac allograft vasculopathy by decreasing the rate of plaque volume and plaque index progression, thereby providing better coronary‐related clinical outcomes [84]. A recent single‐center study of pediatric and young adult Fontan recipients also reported comparable post‐transplant outcomes in patients receiving heart–liver transplants and heart transplant alone despite higher inotropic requirements and more advanced liver disease in the heart–liver cohort [82]. Stanford University investigators have published reports of up to nine pediatric heart–liver transplants for failed single ventricle palliation where the organs were procured and implanted en bloc [70, 82]. An advantage of the “en bloc” technique when the heart and liver are implanted together during CPB is that the new heart is protected from the hemodynamic and metabolic derangements that are typically seen when the liver is implanted and reperfused separately after CPB separation. Reliable estimation of perioperative mortality is challenging and requires separate consideration of the risks posed by hepatic and cardiac dysfunction. Important challenges include the intraoperative management of massive transfusion and vasoplegia [70, 82] and postoperative renal dysfunction [82, 84, 85]. Overall risk and transplant listing for CHLTs is influenced more by cardiac status than liver status [86]. Current UNOS policy allows patients waitlisted for heart–liver to be also waitlisted for heart‐only and liver‐only transplantation. If a heart–liver patient is offered a heart or a liver, the additional organ from the same donor is also allocated. HLT is covered in detail later in this chapter. Briefly, institutional preferences drive HLT versus single‐ or double‐lung transplant in patients with elevated PVR. Current ISHLT data show comparable outcomes for HLT versus lung transplant or double‐lung transplant for patients with idiopathic pulmonary arterial hypertension [87]. Disadvantages of HLT include significantly longer wait times, the need for higher levels of immunosuppression in patients with double‐organ transplants, and surveillance of two organs for rejection. Currently, HLT is recommended in patients with demonstrable LV dysfunction and/or in patients with complex CHD with elevated PVR. Figure 32.7 Perioperative checklist for Fontan patients undergoing heart or combined heart–liver transplants: Stanford Children’s Approach. CT, computed tomography; US, ultrasound; CPB, cardiopulmonary bypass; MAPS, mean arterial pressures; HD, hemodialysis; INR, international normalized ratio; KCentra, prothrombin complex concentrate; TEE, transesophageal echocardiography; PICC, percutaneously inserted central line; PLE, protein‐losing enteropathy; TXA, tranexamic acid; VAD, ventricular assist device; PRBC, packed red blood cells; FFP, fresh‐frozen plasma; TEG, thromboelastogram; IVIG, intravenous immunoglobulin. Figure 32.8 Algorithm for heart transplant versus combined heart–liver transplant in patients with Fontan‐associated liver disease. (Source: Emamaullee et al. [81]. Reproduced with permission of Wolters Kluwer Health, Inc.) Immunosuppression following heart transplantation is lifelong. Despite advances in immune therapy, rejection continues to be a major source of morbidity and mortality in the postoperative period. Pediatric heart transplant provides a unique immunological opportunity because the development of the immune system extends beyond infancy into childhood. T‐cell responses and phenotype are naive compared with adults, with decreased expression of integrins and adhesion molecules. Younger age at the time of transplantation is associated with better long‐term survival and lower frequency of rejection compared with older children [88]. Infants and young children (up to 5 years of age) have undergone transplantation across ABO barriers without developing clinical rejection as discussed earlier. Choice of immunosuppressives is largely guided by institutional experience and the recipient’s clinical profile, rejection history, and comorbid associations. Currently, there are no randomized trials to guide management. Stanford Children’s Hospital current immunosuppression protocol is attached as an example (Table 32.4). Typical clinical use of these agents is summarized below [89]. Immunosuppression consists of an induction and maintenance phase with additional therapies to manage rejection. Induction therapy. Induction phase allows for rapid establishment of immunosuppression. Approximately 75% of transplant recipients between 2010 and 2018 received induction therapy [4]. The most common agent used are polyclonal antilymphocyte or antithymocyte globulin. Despite its popularity in children, significant benefits of induction therapy for survival, CAV, or incidence of rejection are yet to be demonstrated in children [4]. Results of a prospective multicenter clinical trial are awaited. Table 32.4 Induction and maintenance of immunosuppression following heart transplantation in children: stanford protocol Maintenance therapy. The objective is to prevent acute and chronic rejection while minimizing the adverse effects of immunosuppression. All regimens involve a calcineurin inhibitor. Adjunct therapy may include an antipurine agent or mammalian target of rapamycin (mTOR) inhibitor. Often, corticosteroids are also administered, although many programs attempt to limit or avoid their long‐term use. Tacrolimus and MMF or mycophenolic acid (MPA) are the most common agents for maintenance immunosuppression at the time of hospital discharge [4] (Figure 32.9). Immunosuppressive therapies can be categorized by their actions into seven groups as follows: Overall survival of transplanted patients increased from 40 to 70% after the introduction of cyclosporine (Sandimmune, Neoral) in 1982. Cyclosporin binds to cyclophilin within the cytoplasm of T cells; this complex inhibits calcineurin phosphatase, thus interfering with the transcription of key cytokines such as IL‐2, required for T‐cell activation and proliferation. It is not a myelosuprressant and B cells, macrophages and granulocytes are unaffected. Microemulsified formulation of cyclosporin provides better bioavailability and lower rejection rates than the original preparation that had unpredictable absorption. Therapeutic drug monitoring is essential because the therapeutic window is narrow and other drugs influence drug levels. Cyclosporine trough levels are usually maintained in the range of 100–300 ng/mL. Adverse effects may be dose‐related and include toxicity of renal, hepatic, and neurological systems, hypertension, hyperlipidemia, hirsutism, and gingival hyperplasia. Tacrolimus (Prograf, FK506) binds to a different cytosolic‐binding protein (FK‐binding protein) and has been particularly effective as a rescue treatment in cases where recurrent rejection has occurred with cyclosporine. Overall patient survival does not differ between the two agents, but there appears to be less rejection with tacrolimus and an improved adverse effects profile with respect to hypertension, dyslipidemia, and long‐term renal function [4, 90, 91]. Like cyclosporine, the therapeutic window is narrow and blood levels can be affected by other medications. Trough levels are maintained in the range of 5–15 ng/mL. In recent years, cyclosporine and tacrolimus have been used in a similar percentage of patients and MMF has largely replaced azathioprine use early after transplant (Figure 32.9). There is a trend to shift patients who are 5 years post‐transplant from cyclosporine‐based regimens to tacrolimus‐based regimens [4]. Agents such as azathioprine (Imuran) and MMF (CellCept) inhibit lymphocyte proliferation by interfering with purine synthesis. One of these agents may be added to calcineurin inhibitor therapy. The choice of either MMF or azathioprine did not have an impact on rejection rate within the first year in the pediatric heart transplant population [4]. Azathioprine is a purine antagonist that inhibits T and B cells. Bone marrow depression is common, and dosing is guided by the white blood cell count. Its use has declined considerably in the past decade. MMF converts to MPA, an inhibitor of purine synthesis. B‐ and T‐cell lymphocytes are suppressed because they lack a salvage pathway. Absorption is variable, and dosing may be guided by blood levels. Gastrointestinal side effects rather than bone marrow depression are the usual dose‐limiting factor. Figure 32.9 Maintenance immunosuppression at the time of hospital discharge following heart transplantation by era (pediatric transplants, January 2005 to June 2018). Analysis is limited to patients who were alive at the time of the discharge. MMF, mycophenolate mofetil; MPA, mycophenolic acid. (Source: Rossano et al. [4]. Reproduced with permission of Elsevier.) Proliferation signal or mammalian TOR inhibitors, everolimus and sirolimus (rapamycin), are newer agents that provide attractive options for use in heart transplantation because they are immunosuppressive and antiproliferative, inhibit vascular smooth muscle and myofibroblast proliferation, and can prevent progression of CAV. mTOR inhibitors work synergistically with calcineurin inhibitors and thus permit the minimization of calcineurin inhibitors without compromising efficacy. This approach is advantageous for most heart transplant recipients and might provide particular benefit in specific cases, such as patients with cardiac allograft vasculopathy, malignancies, and renal dysfunction, or in patients intolerant to other immunosuppressive agents. Sirolimus may inhibit the process of CAV. Adverse effects include hyperlipidemia, wound healing complications, and proteinuria [92]. These drugs inhibit wound healing. Patients taking mTOR inhibitors and scheduled for elective surgery must be switched 2–4 weeks preoperatively to other medications and then restarted 2–4 weeks postoperatively. Corticosteroids are nonspecific anti‐inflammatory agents widely used in the precyclosporine era. Steroids decrease cytokine production and reduce the circulating CD4+ T cells. Although a mainstay in induction therapy, steroids are currently used in combination with other immunosuppressants early in transplant. Centers try to minimize the dose and taper off by 6 months because of the myriad of side effects including higher infection risk, diabetes mellitus, bone demineralization, and coronary artery disease. Rejection risk may increase when steroids are withdrawn. High‐dose corticosteroid, usually methyl prednisolone, is the first‐line therapy for acute rejection. Recurrent moderate rejection can usually be controlled with enhanced maintenance therapy (tacrolimus, sirolimus) and corticosteroids. Other agents such as polyclonal anti‐T antibodies are reserved for severe rejection that is refractory or causing hemodynamic compromise. Chronic rejection is manifest as coronary vasculopathy. There are no proven therapies that can halt or reverse this process, and retransplantation is the most suitable option for advanced diffuse disease. It remains controversial whether statins affect the progression of allograft vasculopathy or aid in its prevention in children [93].
CHAPTER 32
Anesthesia for Cardiac and Pulmonary Transplantation
Heart transplantation
Diagnostic indications for heart transplantation
Category (abbreviation)
Diagnoses in category
Congenital heart disease (CHD)
Congenital heart defect: HLHS – unoperated; with surgery; without surgery; previous surgery unknown; or valvular heart disease
Dilated cardiomyopathy (DCM)
Dilated myopathy due to: adriamycin; alcohol; familial; idiopathic; myocarditis; postpartum; viral; or other reasons
Retransplant (RETX)
Retransplant due to: acute rejection; chronic rejection; coronary artery disease; hyperacute rejection; nonspecific; primary failure; restrictive/constrictive causes; or other reasons
Other (OTHER)
Arrhythmogenic right ventricular dysplasia; cancer; coronary artery disease; myopathy‐ischemia; hypertrophic cardiomyopathy; muscular dystrophy; restrictive cardiomyopathy (any reason); or other cardiac disease
Dilated cardiomyopathy
Congenital heart disease
Other indications
Retransplantation
Primary DCM
Familial/genetic
Sarcomeric
Mitochondrial diseases
Neuromuscular disorders
Laminopathies
Secondary DCM
Inflammatory
Toxins
Iron
Lead
Cobalt
Arsenic
Anthracycline
Radiation
Metabolic disorders
Endocrinopathies
Thyroid disorders
Catecholamine‐secreting tumor
Parathyroid disease
Diabetes mellitus
Fatty acid oxidation disorders
Carnitine deficiency
Malonyl coenzyme decarboxylase deficiency
GSDs
GSD type II (Pompe disease)
GSD type IV (Andersen disease)
Lysosomal storage disorders
Gaucher disease
Mucopolysaccharidoses
Sphingolipidoses
Nutritional disorders
Thiamine deficiency
Selenium deficiency
Protein energy malnutrition
Structural heart diseases
Valvular heart disease
Ischemia: coronary artery anomalies, coronary artery injury
Single ventricle
Pulmonary diseases
No.
%
Acute rejection
19
9
Hyperacute rejection
7
3
Coronary allograft vasculopathy
111
51
Chronic rejection
16
7
Nonspecific graft failure
34
16
Primary failure
10
5
Other
22
10
ABO‐incompatible heart transplantation
Recipient evaluation
CVS anatomy and hemodynamics
End‐organ dysfunction/chronic noncardiac disease
HLA sensitization and immune function
Psychosocial evaluation of patient and patient’s family
UNOS waitlist status and mortality
Recipient pretransplant management
Donor management
Developments in donor–recipient size matching
Surgical technique
Anesthetic management
Pre‐CPB period
Multidisciplinary preoperative huddle and planning
Anesthesia induction and monitoring
CPB period
Post‐CPB period
Failing Fontan transplants
Combined heart and liver transplantation
Heart–lung transplantation
Immunosuppression
Induction therapy: Administered in the operating room following hemostasis from CPB.
Methylprednisolone: 10 mg/kg (max. dose is 500 mg).
POD# 0–7: 1. Rabbit antithymocyte globulin 1.5 mg/kg/dose; 5 doses given over 5–7 days.
2. Methylprednisolone: 3.3 mg/kg every 8 hours X3 doses and then tapered to 0.4 mg/kg/dose, twice daily. Once tolerating oral medications prednisone/prednisolone at 0.5 mg/kg, twice daily can be started.
3. Mycophenolate mofetil: 600 mg/kg twice daily.
4. Tacrolimus: 0.05 mg/kg orally started on POD#5 and if renal function is normal.
Maintenance therapy: Patients may be weaned from prednisone based on the following criteria
If first biopsy is negative or on POD #7–14, whichever comes first:
If above criteria are not met, steroids are continued and weaned gradually over 6 months.
Sensitized protocol
If the patient is being treated under “sensitized” protocol due to the presence of antibodies, additional medications may be necessary during the perioperative and induction phase. Plasmapheresis 1.5 volume exchange during CPB.
Intravenous gammaglobulin after separation from CPB.
Calcineurin inhibitors
Antiproliferative agents
mTOR inhibitors
Corticosteroids
Rejection therapy
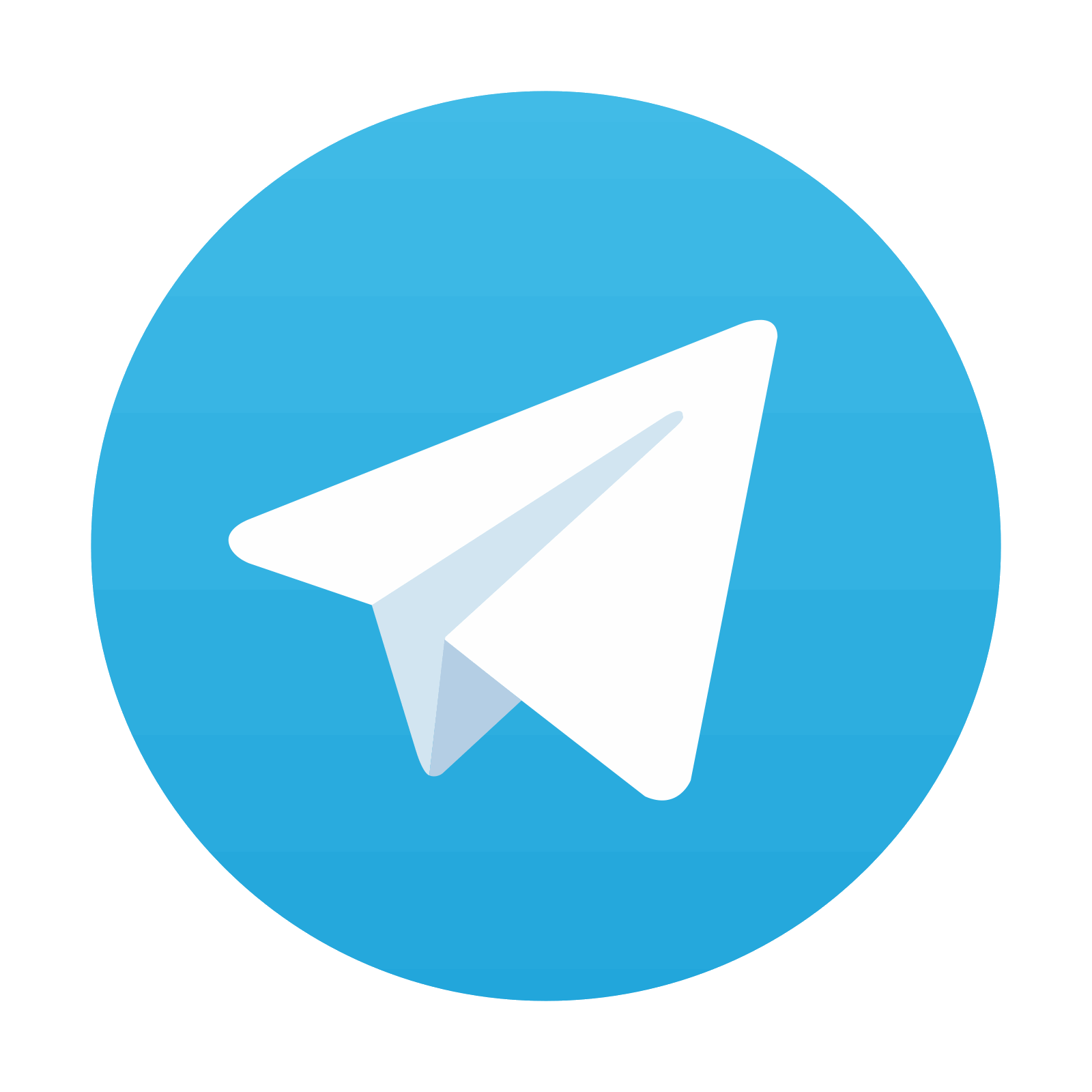
Stay updated, free articles. Join our Telegram channel
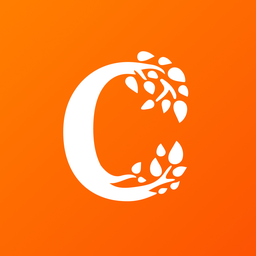
Full access? Get Clinical Tree
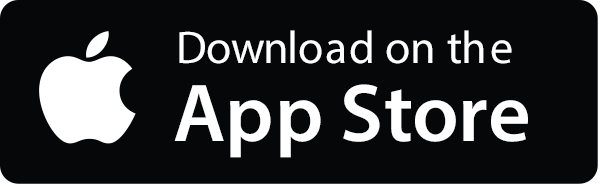
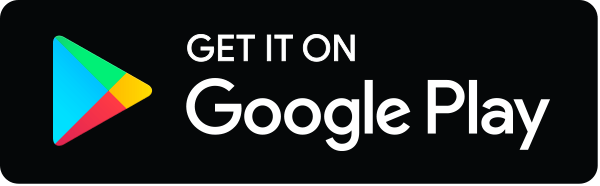