Premal M. Trivedi1, Philip Arnold2, Aarti Shah2, and Athar M. Qureshi3 1 Department of Pediatric Cardiovascular Anesthesiology, Texas Children’s Hospital, Houston, TX, USA 2 Department of Cardiac Anaesthesia, Alder Hey Hospital, Royal Liverpool Children’s NHS Trust, Liverpool, UK 3 Department of Pediatrics, The Lillie Frank Abercrombie Section of Cardiology, Texas Children’s Hospital and Baylor College of Medicine, Houston, TX, USA Few areas in the field of congenital heart disease (CHD) have evolved as rapidly as the discipline of cardiac catheterization. Lesions that were previously managed with surgical intervention alone or considered inoperable altogether are now treated in the cardiac catheterization laboratory (CCL), with benefits ranging from increased survival to decreased morbidity and lengths of stay. Coincident with these advances, however, have been increases in patient acuity and complexity as well as procedural risk. For the anesthesia team, these changes have underscored the need for risk assessment and preprocedural planning to prevent adverse events, and further, awareness regarding procedure‐specific complications and their management. These aspects of anesthetic care are a focus of this chapter and are presented both generally and within the context of individual procedures that may be encountered in the modern CCL. Cardiac catheterizations purely for diagnostic purposes have decreased over the years due to advances in noninvasive imaging. Nonetheless, diagnostic cardiac catheterization remains an important tool in the evaluation of the anatomy and physiology of many patients with CHD. Common indications are as follows [1]: Figure 34.1 Diagnostic catheterization in a child with pulmonary hypertension. A 4‐year‐old child with a late diagnosis of perimembranous VSD and subsequent pulmonary hypertension presented for hemodynamic evaluation to assess candidacy for VSD closure. Measurements were taken on room air and subsequently on 100% FiO2 and inhaled nitric oxide as part of acute vasodilatory testing (AVT). At baseline, Qp : Qs was 0.65 : 1, indicating right‐to‐left shunting at the VSD, and PVRi was 11.4 Wood units/m2. With AVT, no decrement in PAP or PVRi was noted. Surgical closure was therefore deferred and therapy for pulmonary hypertension was initiated with plans to reassess PVR in 6 months. LAP, left atrial pressure; MAP, mean arterial pressure; PAP, pulmonary artery pressure; PPM, parts per million; PVRi, pulmonary vascular resistance indexed to body surface area; RAP, right atrial pressure; RVP, right ventricle; SVC, superior vena cava; VO2, oxygen consumption; VSD, ventricular septal defect. (Source: Mullins and Mayer [2]. Reproduced with permission of John Wiley & Sons.) As implied, the results of diagnostic catheterizations can have an outsized impact on the management strategy for a given patient. For example, the PVR calculation in a child presenting for a cardiac transplant evaluation may determine whether or not the child is eligible for transplantation. These stakes underscore the need to ensure that the hemodynamic variables being measured are as representative of the patient’s baseline physiology as possible. For the anesthesiologist, an understanding of how airway management, common anesthetics, oxygenation, and ventilation can affect these measurements is critical (see Anesthetic techniques). A general strategy to approximate baseline physiology is to conduct the anesthetic on room air with normal ventilation while maintaining an age‐appropriate blood pressure and heart rate. For patients who need oxygen (or who are on oxygen at baseline), the higher partial pressure of oxygen is accounted for in calculations of flows and resistances. The vessels most commonly accessed are the femoral vein and femoral artery. The femoral artery can be used solely for blood pressure monitoring or to evaluate the coronaries, left ventricular end‐diastolic pressure (LVEDP), or MAPCAs as indicated. The internal jugular vein may be used to access the pulmonary arteries (PAs) in patients with Glenn or hemi‐Fontan circulations or to facilitate procurement of endomyocardial biopsy specimens in biventricular hearts. Alternative venous approaches, such as transhepatic or transsplenic, may be indicated in children with a history of occlusions at other sites and are associated with increased risks of bleeding. Following access, pressures and oxygen saturations are obtained in sequence throughout the cardiac, PA, and systemic arterial circulations (see Table 34.1 for normal parameters). These values are then used to calculate shunts (if present), flows (Qp and Qs), and resistances (PVR and SVR; see Table 34.2 for calculations). Stable hemodynamics along with constant oxygenation and ventilation (room air and normocapnea if possible) are essential during the sampling of these values. Flows in children are most often calculated using the Fick principle, which relates oxygen consumption (VO2) to flow. VO2 in this equation can be measured directly using respiratory mass spectrometry or estimated using nomograms based on age, sex, and heart rate [3]. Due to ease, estimations of VO2 are most commonly used, but by virtue of being estimations, they may introduce important error in the calculations of flow and resistance [4–9]. For example, if the estimated VO2 is significantly higher than actual VO2, the resulting cardiac output will be overestimated, while the calculated resistances will be underestimated. The majority of patients undergoing endomyocardial biopsy do so uneventfully, using either general anesthesia with an artificial airway or sedation with a natural one. Certain high‐risk patients, however, warrant discussion, including (i) children less than 6 months of age and less than 8 kg who due to their small size are at increased risk of complications such as ventricular perforation and significant arrhythmias [10], (ii) heart transplant patients with an active concern for rejection or a history of coronary allograft vasculopathy (CAV), and (iii) patients otherwise being evaluated for myocarditis. Children with active rejection or myocarditis may have severely depressed function with coinciding arrhythmias due to myocardial irritability. The process of placing catheters within the heart and taking biopsy specimens can further exacerbate or prompt new arrhythmias. Because of tissue friability, these patients may also be at increased risk of myocardial perforation and tamponade. Preparation should be commensurate with the above risks and entail plans to resuscitate in the event of hemodynamic decompensation (including discussions of extracorporeal membrane oxygenation [ECMO] or prophylactic placement of percutaneous left ventricular (LV) support as a bridge to recovery). If sedation is chosen as the anesthetic technique, one should be prepared as always to escalate to general endotracheal anesthesia (GETA). Of note, in heart transplant patients with signs of heart block and/or rejection, the use of dexmedetomidine may provoke a significant bradyarrhythmia [11]. Attention to the patient’s baseline electrocardiogram (EKG) and risk for heart block can inform the decision of whether or not to use this drug (see section on Anesthetic drugs). The use of dexmedetomidine in a heart transplant patient has also been associated with impaired metabolism of tacrolimus resulting in supratherapeutic levels [12]. Though not immediately relevant to anesthetic management, this risk may indicate the need for more frequent monitoring of tacrolimus levels in the postprocedural period. CAV, or transplant coronary artery disease, is marked by the progressive obliteration of the graft’s coronary vasculature and is a result of immunologic and nonimmunologic factors [1, 13]. Due to the variable reinnervation of the transplanted heart, angina may not occur to warn of its presence, and CAV may only be diagnosed incidentally during the patient’s annual coronary angiography (Figure 34.2). Risk factors for CAV include rejection within the first‐year post‐transplant and increasing graft age [13]. Adolescents are at greatest risk, with only 50% remaining CAV free at 10 years post‐transplant [14]. The patient who should raise one’s concern for CAV would be a teenager several years out from transplant who on prior catheterizations had unexplained hypotension or an elevated right ventricular end‐diastolic pressure (RVEDP) (potentially indicative of microvascular disease). In patients with known CAV, graft survival decreases to 50% at 5 years postdiagnosis [14]. As in any patient at risk for myocardial ischemia, anesthetic goals focus on minimizing myocardial oxygen consumption and maximizing oxygen delivery. Hypotension should be diligently avoided. Table 34.1 Normal cardiac catheterization data BSA, body surface area; PA, pulmonary artery. Right heart catheterization (RHC) remains the gold standard to diagnose and characterize PH, assess the response to AVT, and provide data to guide risk stratification [15] (see Table 34.3). Data is obtained at baseline (or rest) and with AVT. AVT is performed to determine the effect of pulmonary vasodilators on PVR. Protocols and the exact pulmonary vasodilators used vary, but a common process is to deliver a FiO2 of 1.0 followed by iNO at 40 parts per million (PPM) [16]. A positive response is defined as a decrease in mean pulmonary arterial pressure (mPAP) by 10 mmHg without a fall in cardiac output [15]. The presence or absence of a response directs pharmacologic therapy and is prognostic [15, 17]. In patients with CHD with an intracardiac shunt (e.g., an older child with a large ventricular septal defect [VSD] and bidirectional shunting on echocardiography), a decrease in PVR with AVT can influence decision‐making on whether or not to attempt closure (see Figure 34.1). The utility of this data, however, needs to be weighed against procedural and anesthetic risks. Adverse events in children with PH undergoing catheterization range from 2 to 14%, with an incidence of cardiac arrest between 0.8 and 5.7% and mortality between 0 and 1.4% [18–28]. Those at greatest risk include ages < 5 months and those with systemic‐to‐suprasystemic PAP [28, 29]. Children with newly diagnosed PH, depressed RV function (regardless of the estimated PAP on transthoracic echocardiography [TEE]), poor functional status, and need for preprocedural vasoactive and/or respiratory support represent another highly concerning group [30] (Table 34.4). In such patients, the risks of catheterization likely exceed its benefits, and empiric therapy with PH agents may be indicated to provide stability before proceeding. Decisions regarding the timing of catheterization are thus critical to minimizing risk and underscore the need for anesthesia involvement in coordinating with the pediatric PH and catheterization teams. Coincident with decreasing risk is the maintenance of preprocedural PH therapy. Patients on oral agents are instructed to take them as scheduled, including the day of the procedure. Caveats include children on iNO prior to catheterization and those on subcutaneous (SQ) infusions of treprostinil. The preprocedural use of iNO is an indicator of illness severity; however, it may be requested that iNO be turned off during the catheterization to establish baseline conditions. Doing so may induce a PH crisis, however, and is not advised in the critically ill patient. If this is nonetheless thought to be necessary, the process of decreasing iNO should be gradual and with awareness that PH crises are often prompted not with incremental decreases, but by going from 1 PPM to off. The team should, therefore, be prepared to reinitiate iNO and resuscitate as indicated. Options for the management of children on SQ treprostinil are to continue delivery as SQ or transition to intravenous (IV) administration. The rationale for the latter is a more stable and visible site for delivery (due to the risks of dislodgement of SQ sites with positioning, variability in SQ perfusion and absorption during anesthesia, and the inability to monitor if under the drapes). If maintaining the infusion SQ, there is a margin of safety even in the event of dislodgement due to treprostinil’s long half‐life (4 hours). Table 34.2 Hemodynamic calculations performed during cardiac catheterization AO, aorta; AOP, LAP, left atrial pressure; MAP, mean arterial pressure; mPAP, mean pulmonary arterial pressure; mPCWP, mean pulmonary capillary wedge pressure; mSVP, mean systemic venous pressure; MV, mixed venous; PA, pulmonary artery; PV, pulmonary vein; PVRi, pulmonary vascular resistance indexed to body surface area; Qp, pulmonary flow; Qs systemic flow; SVRi, systemic vascular resistance indexed to body surface area; VO2, oxygen consumption Figure 34.2 Coronary angiogram during routine post‐transplant evaluation demonstrating coronary allograft vasculopathy. Arrows indicate gross areas of stenosis. The graft was 11 years old and the child was asymptomatic. He developed profound hypotension following anesthetic induction and a ventricular fibrillation arrest following angiography. He was subsequently retransplanted. (Source: Texas Children’s Hospital Cardiac Catheterization Laboratory.) Table 34.3 Hemodynamic definitions of pediatric pulmonary hypertension based on catheterization data Source: Modified from Rosenzweig et al. [15]. mPAP, indicates mean pulmonary arterialy pressure; PCWP, pulmonary capillary wedge pressure; PH, pulmonary hypertension; PVRi, pulmonary vascular resistance indexed to body surface area; TPG, transpulmonary gradient (mPAP – PCWP or mean left atrial pressure); WU, Wood units Group 1: PAH; Group 2: PH due to left heart disease; Group 3: PH due to lung disease and/or hypoxia; Group 4: PH due to pulmonary artery obstructions; Group 5: PH with unclear and/or multifactorial mechanisms Table 34.4 Determinants of risk in pediatric pulmonary hypertension Source: Hansmann et al. [30]. Reproduced with permission of BMJ Publishing Ltd. These determinants have only level of evidence C because of lacking pediatric data, but are informed by clinical practice and can nonetheless guide preprocedural risk assessment and preparation. BMI, indicates body mass index; BNP, B‐type natriuretic peptide; CI, cardiac index; mPAP, mean pulmonary artery pressure; mRAP, mean right atrial pressure; mSAP, mean systemic arterial pressure; PVRi, pulmonary vascular resistance indexed to body surface area; RA, right atrium; RV, right ventricle; TAPSE, tricuspid annular plane systolic excursion. Anesthetic technique and preparation depend on patient age, comorbidities, disease severity, and institutional practice. Older adolescents or adults who are cooperative can be managed with minimal sedation and local anesthetic infiltration. Upper extremity venous access to perform RHC can be considered to minimize discomfort and the need for analgesics relative to femoral access [31]. Deeper sedation can be used safely in appropriately selected patients but requires attention to avoiding hypoxia, hypercapnea, and acidosis that may prompt either a PH crisis or skew assessment of PAP and PVR. Given the younger age of this patient population, our most common approach is to use GETA. We and others have not observed PH crises related to airway instrumentation [19, 32–34]. Anesthetic induction and maintenance are tailored to the patient’s disease severity. A balanced anesthetic using a combination of opioid, ketamine, midazolam, and volatile agent is well tolerated (see Anesthetic drugs). Ketamine and dexmedetomidine do not adversely affect the pulmonary vasculature and can reduce the amount of volatile agent needed [32, 33]. Most commonly observed following induction is relative hypotension secondary to anesthesia‐induced decreases in SVR, contractility, and preload (with positive pressure ventilation [PPV]). Phenylephrine boluses or infusions of vasopressin or phenylephrine are useful in increasing SVR and preventing RV ischemia that can lead to cardiovascular collapse. For high‐risk patients (those with moderately to severely depressed RV function), infusions of epinephrine and/or vasopressin can be initiated prior to induction. Further, the availability of ECMO should be confirmed in advance (assuming the child is an ECMO candidate). Blood, iNO, vasoactives, adequate vascular access to deliver infusions, and defibrillation pads should be present. A dedicated arterial line should be considered. For all patients following AVT, iNO should be titrated off slowly, particularly in those who have had a positive response. Postprocedural disposition is dictated by disease severity and intraprocedural course. Our practice is conservative, and many of our PH patients are admitted to the intensive care unit (ICU) if only for observation. The number of centers utilizing cardiac magnetic resonance imaging (CMR)‐guided catheterization has increased in recent years [35–39]. These procedures have largely been diagnostic only due to limitations in MRI‐compatible devices and catheters. The sequence of the procedure varies but most often entails CMR prior to catheterization. Other institutions begin the procedure in the CCL where access is obtained, followed by a transition to CMR where imaging and RHC are performed. The child is then transferred back to the CCL where any interventions, if needed, can be done [39]. Common populations currently assessed include post‐transplant patients and those with PH, cardiomyopathy, and intracardiac shunts. The rationale for using CMR‐guided catheterization is the accuracy of the data obtained and decrease in radiation exposure relative to traditional catheterization. Calculations obtained in the CCL are subject to assumptions and sampling error and are further influenced by the child’s underlying anatomy, hemodynamic fluctuations, and the use of oxygen and iNO [35, 40]. CMR avoids many of these confounders and is thus regarded as more precise in calculation of flow and resistance. Other advantages include superior assessment of RV volume, function, and valvar regurgitation relative to other diagnostic modalities and the ability to provide enhanced soft‐tissue characterization. Potential benefits to the latter could include improved myocardial biopsy yields and electrophysiology (EP) ablation success, as well as earlier detection of bleeding complications [41–43]. No less importantly, the radiation exposure to the child and providers is reduced. For example, in a child undergoing pre‐Fontan evaluation who receives CMR prior to catheterization, the fluoroscopy time needed to obtain pressures and saturations can be eliminated. These benefits, however, come with an increase in the time that the patient is anesthetized and the introduction of other risks related to the MRI environment and transfers between CCL and MRI. In a review of their initial experience in patients undergoing MRI‐guided right heart catheterization (MRI‐RHC), Children’s National Medical Center reported that the average anesthetic time for a follow‐up transplant biopsy was 2 hours and 56 minutes and for all procedures 3 hours and 24 minutes [39]. Risk in the MRI environment is multifactorial, but the foremost concern is the inadvertent entry of ferromagnetic objects. Protocols that prompt metal screening before the start of catheterization and once again prior to entry into MRI can be preventative [39]. Hemodynamic instability and cardiac arrest during MRI‐RHC present additional challenges. Much of the equipment used for resuscitation cannot enter MRI safely, and immediate evacuation of the patient to a different location is indicated. Safe and efficient evacuation is dependent on a well‐rehearsed plan that also defines provider’s roles in such an event [39]. Patient transfers between CCL and MRI (and back) further add to complexity and provide opportunities for unplanned extubation or loss of vascular access. Nonetheless, many of the above risks can be mitigated with appropriate planning, team awareness, and attention to detail. Of the 72 procedures for MRI‐RHC at Children’s National, no complications from anesthesia or the MRI‐RHC were reported [39]. A rational approach when first planning for these procedures is to over‐prepare. Simulation involving all team members is helpful in walking through the sequence of the procedure, identifying problem areas, and determining institutional specific responses to critical events. Radiology can often lead team education regarding MRI risk and also help establish protocols for the safe passage of the patient between CCL and MRI. Interval drills to practice evacuation from MRI in the event of emergencies are recommended. Many programs that set out to do MRI‐RHC already have protocols in place for hybrid operating room–MRI suites that can be adapted to the CCL. Patient selection is equally important. Experience first with older and healthier patients can guide subsequent planning on higher risk patients. Anesthetic preparation accounts for setting up MRI as well as the CCL (Table 34.5). Monitors, infusion pumps, and the anesthesia machine within the MRI suite will of course need to be MRI compatible. For patients with PH, an MRI‐compatible iNO delivery system is also necessary. Given the complexity and novelty of this procedure, GETA is reasonable, and the threshold for placing dedicated arterial access for blood pressure monitoring may be lower. Table 34.5 Checklist for anesthetic preparation for CMR‐guided catheterization Source: Modified from Deutsch et al. [39]. CLL, indicates catheterization laboratory; CMR, cardiac magnetic resonance; iNO, inhaled nitric oxide; MRI, magnetic resonance imaging; PH, pulmonary hypertension. The range of interventions offered and the patient types managed in the CCL has broadened significantly in recent years. An overview of common as well as emerging procedures, their anesthetic management, and associated complications are discussed below. Among the most common interventional procedures performed are transcatheter closures of the patent ductus arteriosus (PDA) and certain atrial‐level communications such as secundum atrial septal defects (ASDs) and patent foramen ovales (PFOs). Other cardiovascular communications amenable to device closure include muscular VSDs, coronary fistulae, and Fontan fenestrations. GETA is used in these cases to minimize patient movement, allow for TEE (ASD and VSD closures), and provide stability in higher risk procedures. This latter group includes the closure of VSDs, preterm PDAs or PDAs in patients with PH, and coronary fistulae. Considerations common to patients undergoing device closure include the need for antibiotic prophylaxis and potential for device embolization (Figure 34.3). Depending on the size of the particular device and where it embolizes, hemodynamic instability can result from obstruction to flow or arrhythmias. Thrombus generation can also lead to stroke in devices lodged within the systemic circulation, thereby necessitating team awareness of the patient’s anticoagulation status while attempts at retrieval are made. While most episodes are readily appreciated while still in the CCL, embolization may also occur during the recovery period. The development of new arrhythmias or changes in hemodynamics should alert one to this possibility. Anesthetic strategies to minimize the risk of delayed embolization include deep extubation (excluding infants or patients otherwise in whom the risk of deep extubation exceeds its benefits), aggressive prophylaxis of postoperative nausea and vomiting (PONV), and extended sedation in the initial recovery period. Dexmedetomidine, morphine, ondansetron, dexamethasone, and/or propofol can be useful agents in achieving these goals, but paramount is communication with the cardiologist to identify those patients at greatest risk. For example, in patients undergoing ASD closure, a large ASD and the presence of multiple deficient rims increase the risk for embolization. If catheter‐based retrieval cannot be achieved (e.g., when the device has embolized to the LV and attempts at retrieval may damage the mitral valve apparatus), surgery is indicated. Figure 34.3 (A) A snare (arrow) is used to encircle the device that has embolized into the left atrium. (B) The snare is then used to withdraw the device that is now collapsed (arrow) for removal through the right femoral venous sheath. (Source: Texas Children’s Hospital Cardiac Catheterization Laboratory.) Complications associated with ASD closure are rare. Apart from embolization (discussed above), the device may encroach upon the atrioventricular (AV) valves or over the ostia of the pulmonary or systemic veins upon release. This risk is minimized by the use of echocardiography to ensure that the veins remain unobstructed and that no new valvular insufficiency or stenosis develops following deployment. Less common are catheter‐induced AV block, cardiac perforation, and systemic air embolization (associated with catheter entry into the left heart) [1]. Of note, endovascular and transthoracic echocardiographic (TTE) guidance may occasionally be used rather than TEE to facilitate closure. Among individuals undergoing PDA closure, preterm neonates and infants pose the greatest challenge due to their associated comorbidities and small size at presentation [44]. Such patients have many of the sequelae of prematurity (intraventricular hemorrhage, bronchopulmonary dysplasia, and a history of necrotizing enterocolitis) and can be as small as 700 g [45]. Due to the increased risk associated with this fragile population, protocols for preparation and the transport of these patients to and from the CCL are necessary (Table 34.6). Once in the CCL, attention is given to maintaining normothermia and assessing the adequacy of ventilation. Uncuffed endotracheal tubes (ETTs) placed in the neonatal intensive care unit (NICU) usually prohibit accurate ETCO2 monitoring due to associated leaks. Options are to replace the ETT with a cuffed one, or to gauge ventilation by the adequacy of chest rise or PaCO2 via blood gas once femoral venous access is obtained (the femoral artery is avoided due to the potential for leg ischemia with access). Due to issues associated with lung compliance and the inherent limitations of the anesthesia ventilator, a NICU ventilator is used routinely for patients weighing <2 kg. Inadvertent dislodgement of the ETT or IV lines, always a risk in younger patients in the CCL, is a particular concern in this population and mandates communication prior to any movement of the table. Core temperature is measured with an oral esophageal probe, which also serves as a marker for the aortic end of the PDA on fluoroscopy. This gives the interventional team an additional landmark when placing the device (Figure 34.4). Hemodynamic derangement and desaturations may occur at different points during the procedure. Compression of the chest with the TTE probe can lead to altered ventilation and desaturation (TTE is used in junction with prograde angiography through the venous sheath to assess the PDA and device position before/after release). The catheter used to deploy the device can cause heart block and more commonly can stent open the tricuspid valve. The resulting tricuspid regurgitation (TR) can cause not only hypotension but also desaturation if an atrial communication is present (due to increased right‐to‐left shunting). Prior to device release, TTE is used to ensure that the device is not protruding into the aorta and causing arch obstruction. Following release, embolization may occur, either into the aorta or, more commonly, into the left PA. Table 34.6 Anesthetic management protocol for premature PDA closures Source: Modified from Hubbard et al. [44]. CCL, indicates cardiac catheterization laboratory; CPAP, continuous positive airway pressure; F, Fahrenheit; NICU, neonatal intensive care unit; PICC, peripherally inserted central catheter; PIV, peripheral intravenous catheter. Figure 34.4 Patent ductus arteriosus closure in a premature infant. (A) Anatomy on prograde venous angiography. dAO, descending aorta; LPA, left pulmonary artery; MPA, main pulmonary artery; PDA, patent ductus arteriosus. Note relationship of esophageal temperature probe (arrow) to the dAO. (B) Device occlusion of the PDA. (Source: Texas Children’s Hospital Cardiac Catheterization Laboratory.) Total IV anesthesia using low‐dose ketamine, fentanyl, and muscle relaxant is a commonly used technique in infants weighing <2 kg due to the use of an NICU ventilator. These drugs have the ancillary benefit of promoting hemodynamic stability. For larger and less tenuous preterm infants for whom an anesthesia machine is appropriate, low‐dose volatile agent is often added. Blood and vasoactive agents should be immediately available. Our practice is to leave these patients intubated for return to the NICU. Transcatheter closure of VSDs is similarly fraught with the potential for hemodynamic derangements [46]. These can include heart block or tachyarrhythmias, AV valve (AVV) and/or aortic valve regurgitation, volume overload, bleeding, and cardiac arrest. Heart block can develop either secondary to catheter‐ or device‐induced injury. When deployed in proximity to the conduction system, the device’s radial force can compress the surrounding tissue. Consequently, attempts at closure of membranous VSDs are associated with a higher incidence of complete heart block (CHB) than muscular VSDs. Though steroids have been administered prophylactically to prevent and subsequently treat device‐related CHB (presumptively to decrease inflammation‐mediated edema), their use remains speculative [47–50]. Valvular regurgitation can similarly occur due to device deployment near the AV or aortic valve, or separately as a result of catheter‐induced trauma. The approach to the VSD, whether retrograde through the left heart or antegrade through the right heart, can cause injury to the aortic valve either alone or both the tricuspid and aortic valves, respectively (Figure 34.5). Notably, recent advancements in delivery systems and softer devices have led to an improved rate of successful closure of various VSDs with lower rates of heart block and arrhythmia [50]. Transcatheter closure of perimembranous VSDs remains controversial, however, due to concerns of heart block, aortic valve injury, and hemolysis associated with residual defects. Given the prevalence of catheter‐induced disturbances, vasoactive support is commonly initiated to provide hemodynamic stability. Adequate peripheral venous access and dedicated arterial monitoring are, therefore, recommended. Transvenous pacing is indicated if heart block persists (and the availability of this equipment should be confirmed prior to starting). Volume overload secondary to flush and contrast administration is common and managed with IV furosemide. Postprocedural disposition can vary depending on the procedure’s course, but ensuring the availability of an ICU bed is advisable should continued intubation or pacing be needed. Coronary artery fistulas (CAFs) are anomalous communications between a coronary artery and a chamber of the heart or another blood vessel (Figure 34.6). In the absence of any other cardiac lesion, these fistulas can be a source of pulmonary overcirculation (in patients with fistulas ending in the RV), myocardial ischemia due to coronary steal, or infectious endocarditis. Interventions to close CAFs can be associated with spasm, thrombosis, or dissection of the coronary [51]. Attention to signs of ischemia on EKG and the availability of vasoactive agents to promote coronary vasodilation (nitroglycerin) or hemodynamic support in the event of hypotension (vasopressin, phenylephrine, and epinephrine) are central tenets of anesthetic management. A dedicated arterial line is indicated as the procedure is performed through the femoral arterial sheath. Following occlusion of the fistula, altered flow dynamics that could lead to stasis and thrombosis proximal to the device with subsequent myocardial ischemia/infarction necessitate the initiation of anticoagulation and postprocedural monitoring in the ICU. A continuous heparin infusion is commonly initiated prior to departure from the CCL to avoid delays in anticoagulation associated with transfer of care. Figure 34.5 Transcatheter ventricular septal defect closure. (A) Antegrade device deployment over an arteriovenous loop. The arterial end of the loop is a wire (arrow) advanced in sequence from the femoral artery through the aortic valve, left ventricle, ventricular septal defect, and into the superior vena cava. The venous end of the loop snares the arterial wire and is externalized through the femoral venous sheath. The delivery sheath (dotted arrow) is then advanced antegrade over the venous end of the loop. (B) Retrograde device deployment entirely from the arterial side. (Source: Morray [50]. Reproduced with permission of Elsevier.) Figure 34.6 Coronary artery fistula. Angiogram demonstrating right coronary artery aneurysmal dilation and continuity with the right ventricle. This 10‐year‐old child was asymptomatic but had pulmonary overcirculation as a result of the fistula. Device occlusion was targeted at the distal narrowing (arrow). (Source: Texas Children’s Hospital Cardiac Catheterization Laboratory.) Children with pulmonary stenosis (PS) can range the spectrum from critically ill neonates to older, otherwise healthy, children. The severity of PS and any associated hypoplasia of the RV and tricuspid valve accounts for these differences in presentation. Neonates whose PS is so severe as to require ductal patency for pulmonary blood flow (PBF) are described as having critical PS [52]. These patients, therefore, have single‐ventricle physiology and are managed with prostaglandin (PGE1) in the periprocedural period. Cyanosis is common in the setting of an atrial‐level communication due to the presence of suprasystemic RV pressures, RV diastolic dysfunction, and TR that promote elevated right atrial pressure and right‐to‐left shunting [53]. The RV is typically hypertrophied with preserved systolic function, but occasionally may demonstrate significant dysfunction. GETA is recommended, and attention to oxygenation and ventilation is necessary to balance Qp : Qs in the setting of the patent ductus. Diastolic hypotension is avoided as subendocardial ischemia can develop in the pressure‐overloaded RV. During the procedure, because the valve is already severely restrictive and PBF is preserved through the ductus, periods of balloon dilation are well tolerated (Figure 34.7). Major complications such as transient or permanent heart block, tricuspid papillary muscle rupture, balloon rupture, and PA tears are rare [53]. Transient bradycardia or catheter‐induced arrhythmias are more common. Postprocedure, PGE1 may be stopped or continued depending on the size and function of the RV. Importantly, even with the complete relief of obstruction, RV diastolic dysfunction will persist as RV hypertrophy subsides over several months. A gradual wean of PGE1 may be needed in the ICU to assess the ability of RV to promote PBF. For those demonstrating persistent ductal dependency, either ductal stenting or a surgical shunt is indicated. Older infants and children presenting for pulmonary valvuloplasty are usually at lower risk. They tend to have a normal‐sized RV and tricuspid valve and are able to generate adequate prograde PBF without the need for a PDA. Cyanosis is, therefore, not common but can still occur in the patient with severe obstruction and an ASD. Overarching anesthetic goals include maintaining euvolemia and avoiding hypotension to minimize the risk of ischemia in the thick and pressurized RV. For those rare patients who present with RV dysfunction due to long‐standing severe PS, inotropes and vasopressors may be needed to bolster cardiac output and achieve normotension while anesthetized. The process of ballooning causes a transient pause in PBF (in contrast to patients with critical PS) and produces predictable changes in hemodynamics and oxygen saturations depending on whether or not an ASD is present. In patients without an ASD, ballooning is associated with periods of hypotension, bradycardia, and desaturation due to the acute loss of LV filling and PBF. In patients with an ASD, blood pressure is typically preserved during ballooning due to right‐to‐left shunting across the ASD, but desaturation occurs nonetheless. These events usually self‐resolve following balloon deflation, but boluses of phenylephrine may be necessary to treat hypotension in at‐risk patients who do not recover within seconds. Preoxygenation prior to ballooning can help minimize the severity of desaturation. Development of severe infundibular obstruction after ballooning, although rare, has been reported [54–56]. Adequate preload and a slower heart rate can ensure ventricular filling in these cases. Beta‐blockade is rarely necessary. Although this procedure can be performed with sedation in the compensated child or adolescent, the variable length of the procedure and the significant stimulation associated with ballooning lead us to use GETA. If uneventful, patients are commonly discharged on the same day. Further along the continuum of PS is pulmonary atresia with intact ventricular septum (PA/IVS). As with critical PS, this lesion is ductal dependent for PBF, and decompression of the RV is sought to establish prograde PBF that may allow for a future biventricular or 1 ½ ventricle repair. These patients, however, may have abnormalities in their coronary anatomy that preclude RV decompression. The pressurized RV can result in the formation of fistulous connections between the RV and the epicardial coronary arteries, and when combined with proximal coronary stenosis or occlusion (present in 9–34% of patients with PA/IVS)[57], an RV‐dependent coronary circulation (RV‐DCC) results [58] (Figure 34.8). Decompression of the RV in this setting would lead to myocardial infarction. Consequently, the first procedure undertaken in the CCL is a diagnostic coronary and RV angiogram to rule out the presence of RV‐DCC. For patients without RV‐DCC, RV decompression and prograde PBF can be established with wire or radiofrequency (RF) perforation, followed by ballooning of the atretic valve. Given these additional steps, the duration of the procedure is longer and the risks of complications greater than with balloon valvuloplasty alone. Risks can include heart block, right heart perforation, tamponade, volume overload, dilutional anemia, and acid–base derangement [59, 60]. Ductal stenting may be performed in the same setting or at a later date (see PDA stenting to augment PBF). Timing is often dependent on the size of the ductus and stability of the patient following what may be a lengthy intervention at the atretic valve [61]. Figure 34.7 Balloon valvuloplasty in critical pulmonary stenosis. (A) Right ventricular (RV) angiogram in a neonate with critical pulmonary stenosis. A very thin jet of antegrade flow can be seen across the pulmonary valve (arrow). Also noted is filling of the coronary arteries (dotted arrows) via fistulous connections (non‐RV dependent in this case). (B) The pulmonary valve was crossed with a fine wire and dilated with a balloon. (C) After pulmonary valvuloplasty, there is a wide jet of antegrade flow (arrow) across the pulmonary valve. (Source: Texas Children’s Hospital Cardiac Catheterization Laboratory.) Figure 34.8 Right ventricle (RV)‐dependent coronary circulation. (A) RV angiogram in a newborn with PA‐IVS. Interruption of the mid‐left anterior descending coronary artery (dotted arrows) with a fistulous connection to the RV is seen. A small circumflex coronary artery (solid arrow) is also seen. (B) Direct angiography in the left main coronary artery confirms interruption of the left anterior descending coronary artery (dotted arrows) and a small circumflex coronary artery (solid arrow). (Source: Cabrera et al. [58]. Reproduced with permission of Elsevier.) As with PS, valvular aortic stenosis (AS) can manifest variably depending on the severity of the obstruction and any associated cardiac lesions. Critical AS is the most severe manifestation and presents in the neonatal period as a lesion that is ductal dependent for systemic blood flow. Definitive treatment entails relieving the valvular obstruction. Medical management prior to arrival in the CCL includes the initiation of PGE1, and in the subset of patients with compromised end‐organ perfusion and respiratory failure, inotropic support, and mechanical ventilation [62]. Of note, hemodynamic and respiratory status should be regarded as tenuous even in the neonate presenting on room air and PGE1 alone. The LV is most commonly dilated and dysfunctional at baseline, and the procedure may further exacerbate hemodynamics due to catheter‐induced arrhythmias, decreases in cardiac output with ballooning, and the potential for new aortic insufficiency following valvuloplasty. GETA is, therefore, used. An infusion of epinephrine is often started with induction of anesthesia if the patient is not already receiving it, and vasopressin may be indicated to optimize coronary perfusion pressure. Due to the risk of arrhythmias, defibrillation pads should be immediately available. The vascular access approach planned by the cardiologist can provide additional insight into specific risks as well as the duration of the procedure. The carotid approach offers the most direct and expedient route to the aortic valve but can be associated with neurologic injury. It is, therefore, reserved for the smallest of neonates for whom the risk of femoral artery injury is thought to exceed that of the carotid [63–65]. To facilitate carotid access, the cardiologist may request that the patient be turned 180° away from the usual position (such that the airway is furthest from the anesthesiologist). Care with positioning of lines and the ETT is necessary in this circumstance to minimize the risk of line displacement or kinking of the ETT. A more common approach to the aortic valve is retrograde through the femoral artery. Antegrade approaches through the femoral vein (and then in sequence through the atrial septum, mitral valve, and LV) are less common due to the instability associated with passing the catheter through potentially smaller left‐sided structures (with risks of arrhythmia, mitral valve damage, and perforation) [59]. If the femoral artery is used, additional arterial access for blood pressure monitoring is indicated. New, hemodynamically significant aortic insufficiency following ballooning (indicated by diastolic hypotension and ischemic ST changes) requires urgent surgical intervention for valve repair/replacement. Management of reinterventions later in life, or first‐time interventions in older children or adolescents, is marked by the usual concern of maintaining an adequate coronary perfusion [62]. Anesthetic technique and risk assessment can be guided by the patient’s age, LV function, severity of the gradient, and comorbidities. For older patients who have a borderline indication for intervention based on Doppler‐derived mean transvalvular gradients, the peak‐to‐peak gradient obtained during catheterization can provide definitive information to direct therapy. In such cases, light sedation may be preferable to general anesthesia during the diagnostic portion since the latter may lead to an underestimation of the actual gradient. Our preference otherwise is to use GETA to avoid the risk of movement with balloon dilation that may increase the risk of aortic valve injury. Importantly, rapid RV pacing may be used to reduce stroke volume and minimize balloon movement during inflation in older patients. The resulting decrease in cardiac output is typically well tolerated in those with preserved LV function but may necessitate treatment with phenylephrine to expedite recovery. As the approach to the aortic valve will most commonly be through the femoral artery, the need for a separate arterial line for dedicated blood pressure monitoring is dictated by the patient’s baseline LV function. A number of pathologies are associated with PA stenosis, including syndromes such as Alagille or Williams‐Beuren and different forms of CHD in which stenosis can develop either independently or as a consequence of surgery (ductal origin of the PA, palliated single ventricles, Tetralogy of Fallot (TOF), truncus arteriosus, and transposition, among others). Similar to valvular PS, anesthetic management in children with PA stenosis is dependent on the degree of stenosis present and its effects on RV pressure and function. Estimates of RV pressure can be gauged by the presence of a TR jet and compensatory RV hypertrophy on echocardiography. Euvolemia and age‐appropriate diastolic perfusion pressures should be maintained to decrease the risk of ischemia. Catheter‐induced arrhythmias and periodic hypotension (due to TR caused by catheters en route to the PAs) are common, particularly in younger patients. Though well tolerated in children with preserved ventricular function, those with depressed function may need vasoactive support. Critical events associated with PA angioplasty/stenting include hemorrhage due to vessel rupture, lung reperfusion injury, and occlusion of the left mainstem bronchus with stenting of the left PA [1, 66–69]. The potential for life‐threatening hemorrhage, though rare with the introduction of covered stents and vessel occlusion, requires the presence of large‐bore IV access and packed red blood cells in the CCL. Lung reperfusion injury is more common, with a reported incidence of 15–22% in published cohorts (higher when segmental and subsegmental branches with severe stenoses are involved) [69, 70]. This presents as a decrease in both lung compliance and PaO2 : FiO2, and occasionally with blood‐tinged fluid in the ETT. Proposed mechanisms include an acute increase in hydrostatic pressure due to increased flow following angioplasty or the acute increase in LV end‐diastolic volume in a noncompliant LV. Treatment entails conservative management with continued mechanical ventilation, increased positive end‐expiratory pressure (PEEP), and diuresis if oxygenation and ventilation are compromised. Rarely, one lung ventilation is needed. In patients at high risk for developing hemorrhage or reperfusion injury, we have occasionally placed a bronchial blocker preemptively (Figure 34.9). The potential for acute left bronchial compression should be considered in the setting of a recently deployed left PA stent with a new finding of diminished lung compliance and left lung atelectasis (assessed via fluoroscopy) [67]. Bronchoscopy can be used to confirm this diagnosis. Additional risk can be inferred based on patient characteristics. Patients with Alagille and Williams‐Beuren syndromes may present for PA intervention and carry comorbidities unique to each condition – namely, the risk of liver disease and coincident supravalvar AS, respectively [71]. Moreover, those with a history of D‐transposition of the great arteries (d‐TGA) status post the arterial switch operation may be at risk for coronary compression with attempts at branch PA stenting. The LeCompte maneuver in these patients can place the branch PAs in proximity to the translocated coronaries, and so testing is done to assess for coronary compromise prior to stenting [72]. The presence of profound hypotension and/or ST changes on EKG with testing also suggests the need to abort. Pulmonary vein stenosis (PVS) in children can be a relentless disease associated with significant morbidity and mortality [73–75]. Classified as either primary (congenital) or postrepair (acquired), the pathophysiology of PVS is an increase in pulmonary venous pressure that can lead to PH, right heart failure, and, ultimately, death [76–79]. Characteristics associated with increased mortality include earlier age at presentation, bilateral disease, multivessel involvement, and the concurrent presence of CHD and/or prematurity [80–82]. The foundation of management is early surgical or catheter‐based therapy to relieve the stenosis followed by repeat catheterizations as indicated to address disease recurrence and/or progression (Figure 34.10). Due to the risk of RV failure secondary to PH, preanesthetic evaluation focuses on the RV and signs of systemic or suprasystemic PAPs [73]. For those entering the procedure with depressed RV function, preparations to support the RV should include the availability of epinephrine, phenylephrine, vasopressin, iNO, and, in the highest risk patients, ECMO. Consideration should be given to starting epinephrine and vasopressin prior to induction in those with moderately to severely depressed function. Though counterintuitive that a pulmonary vasodilator would be useful in patients with a downstream obstruction, some patients with PVS may respond to iNO with a significant reduction in PAP [83]. Its use may be considered if acute reductions in pulmonary afterload are needed (and can be stopped quickly if the patient deteriorates with its initiation). Figure 34.9 Bronchial blocker with pulmonary artery angioplasty. A left bronchial blocker (arrow) has been inserted prophylactically to contain hemorrhage in a 7‐year‐old child with PA/VSD/MAPCAs undergoing pulmonary artery angioplasty. An angioplasty balloon is seen inflated in the left pulmonary artery. (Source: Texas Children’s Hospital Cardiac Catheterization Laboratory.) Figure 34.10 Pulmonary vein stenosis angioplasty and stenting. (A) Severe left lower pulmonary vein stenosis (arrow) can be seen in a 1‐year‐old. (B) After left lower pulmonary vein stenting (arrow), a left lower pulmonary artery wedge injection shows an unobstructed left lower pulmonary vein. Also noted is a stent in the left upper pulmonary vein. (Source: Texas Children’s Hospital Cardiac Catheterization Laboratory.) Preprocedural respiratory status can also indicate disease severity and is related to the number of veins involved and the degree of stenosis. Children presenting in respiratory distress due to pulmonary venous congestion may be tachypneic with retractions and in need of noninvasive ventilatory support or intubation. Even in the absence of RV dysfunction, such signs indicate limited reserve and the potential for instability with anesthetic induction and maintenance. Because lung compliance, oxygenation, and ventilation can all worsen during the procedure (as discussed below), patients already starting with pulmonary edema may demonstrate less tolerance for additional insults than those without it. Preemptive diuresis with IV furosemide (1 mg/kg) is often used in at‐risk children (and can be re‐dosed as indicated). Procedural risk and duration can otherwise be gauged by the number of veins needing intervention, whether an ASD is present, and if the femoral veins are patent. Multivessel PVS is associated with both a longer intervention and an increased risk of instability. The latter is related to the potential for pulmonary edema and hemorrhage that increases incrementally with each vein requiring intervention. In the absence of an ASD, transseptal puncture or RF perforation is needed to pass through the atrial septum to access the pulmonary veins. Though generally safe, this added step confers the risk of cardiac perforation while also increasing overall procedural time. Stenotic or thrombosed femoral veins can present another challenge to the interventionalist. Recurring use of the femoral veins with repeat interventions can render them inaccessible. Transhepatic venous access may be needed, which also carries a higher risk of bleeding and AV block [84]. A review of prior anesthetics in patients presenting for repeat intervention can often provide insight into the need for these additional procedures and general information regarding how the anesthetics and interventions were tolerated. The previously alluded to complications of pulmonary edema and pulmonary hemorrhage can be anticipated in children with severely stenotic veins. Patients without any prior intervention and evidence of significant disease are at the highest risk. Pulmonary edema can present either gradually with fluid overload related to flush and contrast administration or more acutely with the transient occlusion of a pulmonary vein during angioplasty and stenting. The latter can also cause pulmonary hemorrhage. Balloon inflation in such settings can be associated with an acute increase in pulmonary venous pressure that can lead to airway bleeding. More common, however, is pulmonary hemorrhage in the setting of a PA wedge injection (used to define pulmonary venous anatomy). Injections into lung segments that are drained by nearly occlusive veins will result in markedly increased capillary pressures (Figure 34.11). Such bleeding is usually self‐limited with subtle changes in compliance but can manifest as gross blood in the ETT or an acute decrease in lung compliance followed by profound desaturation. As with the management of reperfusion injury, pulmonary hemorrhage can be treated conservatively with suctioning, continued mechanical ventilation, diuresis, and increased PEEP and FiO2. In severe or persistent cases, endotracheal instillation of tranexamic acid can be efficacious [85]. The need for ECMO is rare, but indicated if hypoxemia is not reversible and contributing to hemodynamic instability. Of note, episodes of either pulmonary hemorrhage or edema that require escalations in hemodynamic and/or ventilatory support should prompt a discussion of whether or not to proceed. If some of the stenotic veins have already been addressed, a reasonable strategy is to return to the CCL once the patient has recovered. If no intervention has yet been performed in the setting of severe, multivessel disease, ECMO cannulation may be needed prior to proceeding. Figure 34.11 Pulmonary hemorrhage with pulmonary artery wedge injection in pulmonary vein stenosis. Right upper pulmonary artery wedge angiogram in a child with an obstructed right upper pulmonary vein. Contrast is seen entering the right bronchus and trachea (arrow). Stents can be also noted in multiple affected pulmonary veins. A peripherally inserted central catheter is also seen entering from the left arm. (Source: Texas Children’s Hospital Cardiac Catheterization Laboratory.) Stenting of the right ventricular outflow tract (RVOT) has gained acceptance as an alternative to the modified Blalock–Taussig shunt (mBTS) in the initial palliation of premature or severely cyanotic infants with TOF (Figure 34.12). In a single‐center review comparing RVOT stenting to the mBTS, patients undergoing RVOT stenting were found to have a shorter hospital length of stay, improved PA growth, and comparable mortality while awaiting complete repair [86]. Common anesthetic concerns in this population include the potential for hypercyanotic episodes, or “tet spells,” prior to stent placement, and the presence of significant noncardiac comorbidities that may influence anesthetic planning. The usual measures taken to prevent a hypercyanotic episode should be employed, such as euvolemia, adequate anesthetic depth, and avoidance of decreases in SVR. In patients who are cyanotic, transfusion to a hematocrit >40% can serve the purpose of providing preload while also increasing oxygen delivery and SVR. FiO2 can be increased as needed. Catheter‐induced arrhythmias or TR can precipitate hypotension and are treated with boluses of phenylephrine or the initiation of a vasopressin infusion. TR can also cause systemic desaturation in the setting of an ASD due to increased right‐to‐left shunting. Transient desaturation can be anticipated with balloon inflation and preemptively managed with phenylephrine and preoxygenation. Because RVOT stenting may constitute definitive therapy in those infants for whom operative risk is prohibitive [87], an awareness of the child’s noncardiac risk factors and how they may influence anesthetic management is critical. Such patients may also present for reintervention in the setting of in‐stent stenosis and remain at‐risk for hypercyanotic episodes until the stenosis is relieved. Figure 34.12 Right ventricular outflow tract stenting in Tetralogy of Fallot. (A) Severe right ventricular outflow tract obstruction (arrow) in an infant with Tetralogy of Fallot with hypercyanotic spells. Also noted is severe valvar pulmonary stenosis (dotted arrow). (B) After right ventricular outflow tract stenting (pulmonary valve annulus intentionally crossed), there is significant improvement in antegrade flow across the right ventricular outflow tract and pulmonary valve. (Source: Texas Children’s Hospital Cardiac Catheterization Laboratory.) Procedural risks include perforation of the PA or RVOT, pulmonary edema, tricuspid valve regurgitation, and stent embolization [86]. Perforation of the RVOT can manifest as a pericardial effusion, whereas puncture of the PA can present as hemoptysis or the appearance of contrast in the pleural spaces or lung fields [73]. Significant pericardial effusions require drain placement. Blood drained from the pericardium may be directly transfused back to the patient if the volume is needed. Protamine can be used to reverse anticoagulation in this setting if the activated clotting time (ACT) is prolonged, and surgery may be necessary if bleeding persists. Pulmonary edema develops most often as a result of reperfusion injury following relief of the obstruction to PBF. Treatment is conservative with continued mechanical ventilation, increased PEEP, and diuresis. Catheter‐based management of coarctation of the aorta (CoA) entails balloon angioplasty with or without stent placement. Patient size and age dictate which approach is used. Angioplasty alone is typically reserved for neonates and infants, whereas combined angioplasty and stenting is used in older patients [88]. Common indications for catheter‐based management include the initial treatment of discrete coarctation in older children and adolescents and reintervention in those presenting with discrete recurrent or residual coarctation (Figure 34.13). Anesthetic management of CoA varies with patient age and clinical status (i.e., ventricular function) [88]. Sedation has been used successfully in older children and adolescents, but a deep level is required to blunt the noxious stimulus of aortic ballooning. Our practice is to use GETA regardless of patient age or ventricular function to reduce the movement‐associated risks of aortic dissection or stent migration. Common aspects of care include the need for large‐bore IV access and blood pressure monitoring in the right upper extremity (RUE). Assuming a left aortic arch with normal branching, the RUE blood pressure reflects both cerebral and coronary pressures (as the innominate artery arises precoarctation). In contrast, a lower extremity or left upper extremity blood pressure would underestimate these values and lead to unnecessary treatment if used. Additional arterial access beyond that placed by the interventionalist is usually unnecessary but may be indicated based on individual patient risk and need for postprocedural monitoring (e.g., infants with severely depressed LV function undergoing angioplasty as a temporizing procedure or for recurrent CoA following stage 1 palliation). Of note, the arterial access placed by the interventionalist is most often at the femoral artery but can include the carotid or axillary arteries in smaller patients (<2 kg) to avoid femoral artery injury. In older patients, rapid RV pacing may be used when stenting is planned to minimize the risk of migration with deployment and is well tolerated in those with preserved LV function. Postprocedural disposition is guided by preprocedural clinical status. For most undergoing elective intervention, neither ICU admission nor IV antihypertensives are needed. Figure 34.13 Coarctation of the aorta angioplasty and stenting. (A) Severe native coarctation of aorta (arrow) in an 18‐year‐old adolescent. (B) After bare metal stent implantation (arrow) from a femoral arterial approach, there is unobstructed flow in the descending aorta. (Source: Texas Children’s Hospital Cardiac Catheterization Laboratory.) Acute complications include vessel dissection or disruption, cerebrovascular accidents, stent migration, and balloon rupture [89, 90]. Patients weighing <30 kg presenting for stenting should raise concern for the potential for access site injury. The sheath size necessary to deliver the stent can approximate the size of the femoral or iliac arteries themselves, thereby increasing the risk of vascular disruption with both sheath placement and withdrawal. Acute hypotension unrelated to pacing or ballooning should elicit evaluation for contrast extravasation. Temporary balloon inflation and subsequent covered stent placement are used to temporize in such situations. The risks of both aortic dissection and cerebrovascular accidents appear higher with older age, with patients over 40 years of age at greatest risk [90]. Aneurysm formation at the site of intervention is a late complication more associated with angioplasty alone than stenting. Ductal stents have been used increasingly in recent years in the initial palliation of neonates with ductal‐dependent PBF (Figure 34.14). Data comparing ductal stenting to the mBTS have demonstrated comparable outcomes in terms of death and unplanned reintervention to address cyanosis [91]. Children receiving ductal stents have decreased lengths of stay in the ICU, lower procedural complications, and larger and more symmetric PAs at the next stage of palliation relative to those managed with a mBTS [88]. These positives are balanced, however, by an increased need for subsequent reintervention and a higher initial failure rate due to an inability to stent the duct [91, 92]. This latter concern and the unique complications that may occur during the procedure are of interest to the anesthesiologist. PGE1 can be either continued or stopped in preparation for ductal stenting. If continued, there may be concern that the PDA is tortuous and at risk for spasm with manipulation. More commonly, PGE1 is stopped hours prior to the procedure [1]. This is to produce a reduction in ductal diameter that allows the stent to be well anchored on release, thereby decreasing the risk of embolization. Ductal narrowing is clinically apparent when the patient begins to desaturate. The time interval between stopping PGE1 and the onset of desaturation, often known to the ICU through previous trial and error, can inform scheduling as well as guide urgency. The ideal situation would be to have the child positioned and prepped in the CCL before severe desaturation occurs. In the event of such desaturation, PGE1 can be restarted (patients who are entirely ductal dependent are particularly vulnerable). For those with an ancillary source of PBF (e.g., PS or PA/IVS following RF perforation and dilation), an alternative strategy can be to start vasoactives to augment PBF while the procedure proceeds urgently. The duct can be approached from a variety of access points dictated by its location on the aorta [93]. The particular approach used influences patient positioning and any additional monitoring that may be needed. Patients with a percutaneous carotid or axillary approach may require positioning such that the airway is directed 180 degrees away from the anesthesiologist (called the “flip technique”) [94]. Care in securing the ETT, IVs, and monitoring lines is essential as patient access is limited in this position. Figure 34.14 Ductal stent for pulmonary blood flow. (A) A tortuous ductus arteriosus (arrow) can be seen supplying confluent branch pulmonary arteries in a neonate with ductal‐dependent pulmonary blood flow. Also noted is a percutaneously placed left common carotid artery sheath. (B) A ductal stent is seen (arrow) that has been placed via a percutaneous left common carotid artery approach. (Source: Texas Children’s Hospital Cardiac Catheterization Laboratory.) Beyond standard monitoring, placement of an additional arterial line is often indicated. Because the arterial access obtained by the interventionalist may be used for deploying the stent, a separate arterial line is needed to provide accurate blood pressure monitoring and blood gas assessment both during and after the procedure. The location of the duct and the interventionalist’s approach can guide where to place this line. For example, in a patient undergoing a left axillary artery approach, a left radial arterial line (and the pulse oximeter on that hand) would be blunted. In the absence of central venous access, two peripheral IVs are placed: one to allow for volume resuscitation and bolus drug administration and the other for continuous infusions of vasoactives. It is the norm at our institution to initiate vasoactives during this procedure, whether with epinephrine, vasopressin, or both. For patients undergoing a common carotid approach, cerebral near‐infrared spectroscopy (NIRS) on the side being accessed may be useful to detect changes in perfusion [95]. A Foley catheter is routinely placed. Other concerns common to the neonatal population include maintaining normothermia and normoglycemia. A forced air warmer and the use of a dextrose‐containing carrier fluid can address these issues. Critical events that may occur with manipulation of the ductus include ductal spasm and thrombosis. In patients who are ductal dependent, either event can lead to life‐threatening hypoxia. Boluses of epinephrine (1–10 mcg/kg) can support hemodynamics while attempts are made to open the ductus, and in the case of spasm, PGE1 can be reinitiated at high dose (0.1 mcg/kg/min) or given as a bolus (0.1 mcg/kg). PGE1 should, therefore, always be immediately available. Thrombosis can be managed additionally with a bolus of heparin (100 units/kg). Preparations for ECMO are made if attempts at restoring ductal patency are not rapidly successful. Even in cases when ductal spasm does not obviously occur, passage of the wire through the ductus can also promote desaturation. FiO2 is increased in anticipation of this event, and vasoactives are titrated to optimize PBF. Other intraprocedural complications of note include dissection and stent embolization [1, 93]. The latter may prove difficult to retrieve and can adversely affect systemic blood flow or PBF. Following successful stenting, oxygen saturations should improve. Attention is then shifted to minimizing the risk of overcirculation by titrating down on FiO2 and vasoactive support as able. Patients are most often kept intubated following the procedure to allow for further stabilization and optimization prior to extubation. Prior to leaving the CCL, perfusion to the extremity used for access is evaluated. If there are any concerns for inadequate pulses or a cool extremity, a heparin infusion is started. Further discussion of ductal stenting is presented in Chapter 19. Balloon atrial septostomy (BAS) is indicated in those forms of CHD that require an unrestrictive atrial septal communication. Examples include d‐TGA (to improve systemic saturations through better mixing), hypoplastic left syndrome (to relieve left atrial hypertension), or tricuspid atresia, total anomalous pulmonary venous return, or severe PS/atresia (to off‐load the right heart and augment cardiac output). The procedure is performed through either umbilical or femoral vein access and, in the case of d‐TGA, can often be done at the bedside with TTE guidance. Once the balloon catheter is positioned in the left atrium, the balloon is inflated and a forceful jerk is applied to bring the balloon back to the right atrial–inferior vena cava junction [1]. This is repeated as needed to achieve an unrestrictive atrial communication. A subset of patients with thicker atrial septae for whom a BAS alone would be ineffective include neonates with hypoplastic left heart and infants older than 4–6 weeks. An atrial communication in these children is created using RF perforation or blade atrial septostomy, followed by ballooning and/or stenting of the atrial septum. Given the increased complexity of this group and need for fluoroscopy, these procedures are performed in the CCL. Different anesthetic techniques can be used in the stable neonate undergoing BAS. Our approach is to intubate using low‐dose fentanyl (and/or ketamine) and muscle relaxant, and in those with an uncomplicated preprocedural and intraprocedural course, to extubate following successful ballooning. Those who are critically ill beforehand, such as the hypoplastic left heart syndrome (HLHS) patient with a highly restrictive atrial septum, are often already intubated and receiving significant inotropic and ventilatory support. Management is supportive until the atrial septum is opened. Thereafter, oxygen saturations should increase but can occur at the expense of systemic perfusion. Acute hemodynamic instability can result with the increase in Qp and loading of the RV. Procedural complications are rare but can include balloon rupture and embolization of balloon fragments, stent embolization (if an atrial septal stent is used), vascular injury including avulsion of the pulmonary vein or inferior vena cava, or cardiac injury or perforation [1]. Examples of the latter are mitral valve injury with distal placement of the balloon, or rupture of the atrial appendage. In the child with worsening PH who is unresponsive to medical therapy, catheter‐based options for decompressing the RV and pulmonary vasculature include atrial septostomy/stenting, PDA stenting, and the reversed Potts shunt (left PA to descending aorta). While these procedures result in desaturation due to right‐to‐left shunting, they differ in the end organs affected [15]. With atrial septostomy, all end organs (including the brain and myocardium) are subject to desaturated blood. In contrast, the PDA stent and reversed Potts shunt deliver deoxygenated blood only to the splanchnic and lower extremity circulations (while preserving oxygenated blood flow to the brain and heart). Additional benefits over an ASD include a decrease in RV afterload, with a subsequent improvement in LV performance through a more normalized position of the interventricular septum. The reversed Potts shunt can be achieved via either ductal stenting in those infants and young children who have a still persistent PDA or the de novo creation of a left PA–descending aorta connection. The latter is achieved using RF perforation and the placement of a covered stent [96]. Concerns for both techniques include the risk of too large a shunt: decreased PBF with subsequent hypoperfusion of the brain and heart (due to decreased LV filling) and severe lower extremity desaturation. Alternatively, blood flow from the aorta may be siphoned into the pulmonary circulation when PAP is subsystemic. One‐way valves have been used to address this [97]. Such patients are clearly among the highest risk for hemodynamic decompensation. Established strategies for the anesthetic management of severe PH apply. Children with the Fontan circulation may need a fenestration in the setting of worsening systemic venous hypertension (presenting clinically as protein‐losing enteropathy or persistent pleural effusions) or to achieve intracardiac access for arrhythmia ablation. An opening is most often created using a combination of needle/RF perforation and ballooning (with or without stenting), fluoroscopy, and echocardiography. Procedural risks include bleeding with pericardial effusion or tamponade, systemic embolization of air or thrombus, and volume overload with lengthy attempts at conduit perforation. Anesthetic risk is associated with the underlying Fontan physiology and the negative effects of PPV (on venous return) and anesthesia (on SVR and venous capacitance). The need for reintervention is almost universal among patients with abnormalities of the RVOT and pulmonary valve. Regardless of the prior palliation (whether a RV to PA conduit, transannular patch, balloon valvuloplasty, or replacement of the pulmonary valve altogether), additional interventions can be anticipated over time to address progressive stenosis and/or insufficiency. Conduits, such as those used in truncus arteriosus, pulmonary atresia, some forms of transposition, and the Ross procedure, fail due to stenosis and insufficiency associated with valve degeneration, loss of conduit diameter, and fixed size as the patient grows. The RV consequently becomes pressure and volume overloaded. On the other end of the spectrum are patients with TOF with transannular patches who develop worsening RV dilation due to free pulmonary insufficiency alone. In both cases, RV failure and intractable arrhythmias can result if the stenosis and/or insufficiency are not addressed. Transcatheter pulmonary valve replacement (TPVR) has revolutionized the management of many of these patients since its introduction two decades ago. Candidates include those who have an adequately sized conduit or native RVOT to accept the available valves [98–100] (Figure 34.15). Broader application is currently limited by the size mismatch between current devices and their landing zones (e.g., a child with a transannular patch may have a dilated RVOT too large to accommodate a TPVR). Devices that are self‐expanding and hour‐glass in shape have recently become available, however, and may lead to increased utilization in this population [98, 101]. The status of the RV guides anesthetic management and risk assessment. Patients with preserved RV function undergoing TPVR are at low risk for anesthesia‐induced hemodynamic instability. Anesthetic techniques can include sedation with attention to increasing depth during stimulating portions (e.g., initial access, sheath upsizing for valve deployment, and ballooning and/or stenting of the existing valve or conduit) or GETA. Lengthy procedural times, the potential for significant procedural complications, and the need to avoid movement during valve deployment bias our management toward the use of GETA. Patients with preexisting RV dysfunction in the setting of long‐standing insufficiency or severe conduit or native RVOT stenosis, in contrast, represent a higher risk group. Such patients may already be receiving inotropic support entering the procedure and may require uptitration and the addition of a vasopressor with the induction of general anesthesia. Occasionally, patients with long‐standing RV dilation may also have TR and significant right atrium (RA) dilation, which provides a nidus for atrial dysrhythmias. Maintaining sinus rhythm and age‐appropriate systemic blood pressure is central to avoiding RV ischemia in the setting of RV hypertension and elevated RVEDP. Large‐bore IV access is routinely obtained to allow for volume resuscitation and vasoactive administration. External defibrillator pads and packed red blood cells need to be immediately available. Additional arterial access apart from that placed by the interventionalist is considered in those with RV dysfunction who may become hypotensive upon induction or with the institution of PPV. Patients who tolerate the procedure well are extubated. Given the large size of the venous sheaths placed (22–24 French), aggressive PONV prophylaxis and analgesia are administered to decrease the risk of bleeding associated with emesis or pain. Figure 34.15 Transcatheter pulmonary valve replacement. A morbidly obese 27‐year‐old female status post Ross procedure at age 9, now with severe conduit stenosis and severely depressed RV function, presented for TPVR. (A) A severely narrowed and calcified RV‐PA conduit is seen (arrow) with regurgitation. (B) After angioplasty, stent placement, and transcatheter pulmonary valve placement (arrow), there is no significant residual stenosis or regurgitation. (Source: Texas Children’s Hospital Cardiac Catheterization Laboratory. Major periprocedural complications include (i) coronary or aortic root compression, (ii) RV to PA conduit rupture, (iii) PA injury, (iv) postdeployment pulmonary edema, and (v) valve malposition/embolization [102–106]. Coronary compression can occur with ballooning of either the RV to PA conduit or the native outflow tract due to proximity of the coronaries to these structures. Consequently, a coronary angiogram is performed simultaneously with RVOT ballooning and after stent and valve placement to ensure coronary patency [100, 105]. Aortic root compression can occur similarly with resulting aortic insufficiency. Partial or total conduit rupture is associated with attempts at ballooning noncompliant conduits, such as those that are heavily calcified and severely stenotic. The subsequent hemorrhage is managed with volume resuscitation and pressor support, while the interventional team places a covered stent to limit further extravasation and a pericardial drain if tamponade develops. Surgery may be needed to provide definitive therapy. PA injury is most common secondary to guidewire perforation and can result in hemoptysis or hemothorax [106]. One lung ventilation may be indicated if severe. Pulmonary edema following the relief of stenosis is thought to be secondary to an acute increase in LVEDP (due to increased PBF) in patients with underlying LV diastolic dysfunction [103] (Figure 34.16). Though this mechanism would suggest a relatively acute presentation, it has also been observed in our institution up to 16 hours after valve deployment. This late presentation may indicate the need for close postprocedural monitoring in the highest risk patients: those with chronic and severe RVOT or conduit obstruction and preexisting LV dysfunction. Management entails aggressive diuresis and supportive mechanical ventilation. No devices are currently approved for transcatheter aortic valve replacement (TAVR) in the pediatric population. Limitations associated with current devices include their large size (and larger delivery sheaths) relative to that needed for most children. Nonetheless, a transcatheter approach offers a reasonably efficacious alternative to surgery for a select group of children who would otherwise be poor surgical candidates [107]. Underlying LV function and the severity of aortic insufficiency (AI) and/or aortic stenosis (AS) guide anesthetic management. Because this procedure is currently limited to those with a relative contraindication to surgical repair, comorbidities can further guide assessment. GETA is the rule due to the potential for complications, invasiveness, need for TEE, and procedure length. ECMO candidacy or plans otherwise in the event of intraprocedural cardiovascular collapse are determined in advance. As in PDA stenting, the approach to deploy the valve can vary and can impact the location of monitoring lines and pulse oximetry placement. In older patients who have adequately sized femoral vessels, the transfemoral approach is considered. Smaller vessel size necessitates alternate approaches: a hybrid transapical approach through a thoracotomy and carotid approach via surgical cutdown have been described [107] (Figure 34.17). A dedicated arterial line in addition to the arterial access placed by the interventionalist is obtained, as are large‐bore peripheral IVs. Vasoactive agents, blood, external defibrillation pads, and a Foley catheter are indicated. Periods of rapid pacing may be used prior to valvuloplasty and valve deployment. The location of pacing depends on the child’s cardiac anatomy but is most often accomplished via the RV. Anticipatory support with boluses of phenylephrine before and after pacing is given. Figure 34.16 Pulmonary edema following transcatheter pulmonary valve replacement. (A) The same patient immediately following transcatheter pulmonary valve replacement. (B) 16 hours postprocedure. The patient developed dyspnea and desaturation requiring re‐intubation and ultimately venovenous extracorporeal support. The presumed mechanism was increased preload to a dysfunctional left ventricle resulting in increased left‐sided pressures. (Source: Texas Children’s Hospital Cardiac Catheterization Laboratory.) Complications unique to children undergoing TAVR include the higher risk for vascular dissection/disruption due to mismatch between commonly used delivery devices and vessel size. As for transcatheter pulmonary valves, surgical backup should be available (if not already involved for access). Common risks otherwise include those of valve embolization and coronary compression with valve placement (due to direct obstruction or ostial narrowing with dilation of the annulus) [107]. Figure 34.17 Transcatheter aortic valve replacement via the right common carotid artery. An 8‐year‐old child status post Heartware® ventricular assist device placement, now with progressive aortic insufficiency. Due to the high risk associated with repeat sternotomy, bypass, and cross‐clamping for surgical valve replacement, a hybrid transcatheter approach was selected. (A) The right common carotid artery (arrow) can be seen exposed via a surgical cutdown, chosen for its larger size relative to the femoral artery. Also seen in the picture is the large delivery sheath used for transcatheter aortic valve placement. (B) Significant aortic regurgitation is seen (arrow). (C) A transcatheter aortic can be seen that was placed (arrow), and 3 years later, the transcatheter aortic valve was working well with trivial paravalvar leak (dotted arrow). (Source: Texas Children’s Hospital Cardiac Catheterization Laboratory.) Hybrid procedures refer to joint surgical and transcatheter interventions occurring in the CCL or operating room. Though most commonly associated with the initial palliation of HLHS, hybrid approaches have also been used in periventricular closure of muscular VSDs, stenting of stenotic pulmonary veins, and valve replacement, among other procedures [108, 109]. The focus of this section is on the hybrid palliation for HLHS. Basic aspects of this strategy include surgical placement of bilateral pulmonary artery bands (bPAB) via median sternotomy followed by ductal stenting [110] (Figure 34.18). Variations abound, however, as to (i) whether the duct is stented (some centers may choose instead to maintain ductal patency with PGE1); (ii) when the duct is stented (either during PAB placement or as a separate procedure); (iii) whether or not an atrial septostomy or atrial septal stent is concurrently performed; and (iv) how to address the risk of retrograde aortic arch obstruction (RAAO). Atrial septostomy is often delayed in the absence of an intact/restrictive atrial septum to allow time for the left atrium to grow. With a larger left atrium, a more aggressive ballooning can be performed, leading to a larger and more durable septostomy [111]. RAAO is an important source of morbidity and mortality in HLHS as the coronary and cerebral circulations are reliant on retrograde flow through the transverse arch (entirely so in the variant of aortic atresia). Clinically significant RAAO is present in 10% of HLHS neonates at birth and can occur in an additional 24% following the hybrid procedure [108, 112]. To decrease this risk of myocardial and cerebral ischemia, some centers also perform a reverse Blalock–Taussig shunt (main PA to innominate artery) at the time of ductal stenting in at‐risk patients. Others view preexisting RAAO as a contraindication to the hybrid procedure [113, 114]. For patients who develop RAAO subsequent to the hybrid procedure, treatment is achieved with retrograde arch ballooning and stenting. Figure 34.18 Hybrid procedure for hypoplastic left heart syndrome. Common elements include bilateral pulmonary artery bands, transcatheter stenting of the patent ductus arteriosus, and an atrial septostomy or atrial septal stent. (Source: Naguib et al. [110]. Reproduced with permission of John Wiley & Sons.) The choice of when to use the hybrid approach versus conventional surgical management (the Norwood procedure) is institution specific and often dependent on patient factors. Advantages to hybrid palliation center around its ability to balance Qp : Qs without the morbidity associated with cardiopulmonary bypass (and deep hypothermic circulatory arrest or selective cerebral perfusion) in the neonatal period. While few centers use the hybrid approach preferentially, others reserve it for those patients thought to be at highest risk for Norwood palliation. Such patients include those with prematurity, birth weight <2.5 kg, presence of LV to coronary fistulae, intact or restrictive atrial septum, severe RV dysfunction, moderate‐to‐severe TR, intracerebral hemorrhage, or persistent end‐organ injury secondary to shock at initial presentation [115–117]. Disadvantages to the hybrid approach focus on the risk of RAAO, need for repeat catheter interventions prior to stage 2 palliation, impact of the bPAB on PA growth, and the complexity of the comprehensive stage 2 procedure (combined Norwood and bidirectional superior cavopulmonary anastomosis). Anesthetic concerns specific to the hybrid approach include the potential for instability with bPAB placement and acute RAAO with ductal stent deployment [59, 110]. Arrhythmias, hypotension, and bradycardia may occur with bPAB placement as a result of contact with the left atrial appendage or manual compression of the ductus. Both are more likely to occur with manipulation of the left PA due to its proximity to these structures. Adenosine and cardioversion can be used to treat persistent supraventricular tachycardia (SVT). Compression of the ductus can be particularly threatening due to the transient decrease in myocardial perfusion. Decreases in heart rate and cerebral saturation may be the first signs of ischemia along with ST depression. Epinephrine in bolus doses of 1–10 mcg/kg may be needed to facilitate recovery. Following placement of the bPAB, systemic blood pressure should increase with a coincident decrease in oxygen saturation. If proceeding with ductal stent placement, a sheath is then placed in the main PA and the stent is deployed under fluoroscopic guidance. Because systemic blood flow is transiently altered during stent deployment, instability can also arise during this time. RAAO secondary to stent encroachment into the aorta can manifest as bradycardia, hypotension, and arrest. Monitoring of a right radial arterial pressure and cerebral saturation is recommended to allow prompt detection [59, 110]. Despite these risks, the procedure appears to be well tolerated. Naguib et al. in a review of the anesthetic management of 77 patients undergoing hybrid palliation reported that epinephrine infusions were initiated in only 4 patients and epinephrine boluses were required in only 5. 27% were transfused blood, and 60% of those who were intubated solely for the procedure were extubated at the end of the case. See Chapter 30 for additional discussion of the hybrid approach for HLHS. The need for pericardiocentesis may arise acutely during interventional catheterization or more electively in a child with a postsurgical, neoplastic, or infectious effusion. The former may occur during endomyocardial biopsies, interventions at the RVOT (RF perforation in PA/IVS, RVOT stenting, transcatheter PVR, or conduit interventions), or transseptal approaches to the left atrium. Clinically, these acute bleeds can result in precipitous hypotension due to tamponade. Treatment requires emergent pericardial drainage with autotransfusion as indicated [118]. Autotransfusion entails siphoning the blood that is drained (a stopcock can be placed on the drain catheter for pulling blood into a syringe) and immediately returning it to the patient without any delay or storage (see Figure 34.19). Additional nonautologous transfusion is often needed in neonates and infants to maintain intravascular volume. Blood pressure is supported with epinephrine, and protamine may be needed to reverse heparin‐induced coagulopathy. Surgery is indicated if the bleeding does not stop with these measures. Children presenting to the CCL for primary drainage of a pericardial effusion can vary in their clinical presentation. Rarely do they present with signs and symptoms of frank tamponade: low cardiac output as evidenced by anxiety, cool extremities, tachycardia, tachypnea, pulsus paradoxus, and marginal blood pressures [119]. More often, patients appear stable, belying their potential for decompensation. Anesthetic management entails ensuring adequate preload and maintaining SVR, contractility, and heart rate (i.e., fast, full, and tight) [119]. Because stroke volume is relatively fixed in tamponade, marked decreases in heart rate will lead to proportional decreases in cardiac output. Postcardiac surgery patients are often on aggressive diuretic therapy preprocedurally in attempts to decrease the size of their effusions. This population needs volume resuscitation prior to anesthesia. GETA is avoided to minimize the additional risks of PPV. Spontaneous ventilation is maintained using a combination of ketamine and midazolam. Morphine is not used predrainage because of its potential to decrease SVR, nor are rapid boluses of dexmedetomidine administered due to associated bradycardia. In children in whom the above efforts are ineffective, an alternative strategy is to use low‐dose sevoflurane (2–3%) to maintain spontaneous ventilation. Vasopressors and inotropes should be at the ready if needed. Positioning of the patient in the CCL is in the semi‐Fowler’s position with back elevated at 30°–45° to allow the pericardial fluid to accumulate inferiorly. The airway remains accessible in this position, but if intubation is required subsequently, the patient is positioned supine. The effusion is drained under echocardiographic guidance following local anesthetic administration. Procedural complications include needle‐ or wire‐induced laceration of the ventricle, coronary artery or vein, arrhythmias, or injury to the lungs or esophagus. Postprocedurally, pulmonary edema may develop due to LV dysfunction associated with the acute increase in LV preload [119]. Additional discussion of pericardial effusion is presented in Chapter 31. Figure 34.19 Autotransfusion following an acute pericardial bleed. A filter is used prior to reinfusion to avoid embolization of thrombi or fat particles from the pericardial space. Autotransfusion should not be performed when there is suspicion for an infectious or neoplastic effusion to prevent systemic spread. (Source: Mossad [118]. Reproduced with permission of Elsevier.) The role of the interventionalist in mechanical circulatory support (MCS) has grown in recent years in parallel with the increased utilization of percutaneous ventricular assist devices (PVADs) in the management of pediatric cardiogenic shock. Such devices may be placed as the primary procedure (e.g., LV decompression while on venoarterial ECMO or as a bridge to a durable VAD, transplant, or recovery) or as a supportive intervention in the setting of another high‐risk procedure (e.g., PVAD backup for an endomyocardial biopsy in a patient with presumed acute graft rejection and tenuous hemodynamics). The oldest of these devices is the intra‐aortic balloon pump (IABP). Used primarily in adults, IABPs have also been used in infants and children [120–124]. IABPs assist the failing ventricle by augmenting diastolic perfusion pressure and decreasing afterload by timing balloon inflation to diastole and deflation to systole [124]. Constraining its use in pediatrics has been the limited support it offers in florid ventricular failure, and technical challenges in timing balloon inflation and deflation to changes in rate and rhythm. Nonetheless, as volumes of adults with CHD increase in pediatric centers, this therapy may experience a resurgence. A more recent introduction has been the off‐label use of the Impella® Heart Pump (Abiomed Inc., Danvers, MA) [125, 126]. The Impella is a continuous axial flow device that extracts blood from the failing ventricle and delivers it to the aorta (Figure 34.20). In doing so, it unloads the ventricle and augments cardiac output. Available configurations include the Impella 2.5, cardiac power (CP), 5, and 5.5, which, respectively, provide flow rates of up to 2.5, 4.3, 5, and 6.2 L/min (Table 34.7). The specific device used is guided by patient size and the indication for support. Most commonly, the 2.5 and CP are used for younger patients or those on ECMO in need of LV decompression. The 5 and 5.5 are used for larger patients and those in need of the higher flows that these devices can provide. The device used, procedural urgency, and anticipated duration of support inform placement at either the femoral artery or surgical cutdown at the axillary artery. Fluoroscopy and TEE guide placement. Echocardiographic features predicting successful placement include a minimum LV apex to aortic valve length of 7.5 cm and an aortic annulus >1.5 cm, corresponding with an average weight of 23 kg and body surface area (BSA) of 0.89 m2 [127]. (Morray 2019). The youngest patient to date supported with an Impella was a 4‐year‐old weighing 15 kg with a BSA of 0.67 m2. RV support can also be provided by the Impella RP, which has been used in a child as young 14 years of age with a BSA of 1.4 m2 [126]. Figure 34.20 Left ventricular support with the Impella 5.5. (A) Blood is entrained through the device inlet located in the left ventricle (arrow) and ejected into the ascending aorta through the blood outlet (dotted arrow). (B) Impella placement (arrow) via a left subclavian cutdown approach in a 17‐year‐old status post heart transplantation with acute rejection. A pulmonary artery catheter is also seen and used for postprocedural management. (Sources: Image A from https://www.heartrecovery.com/products‐and‐services/impella/impella‐55‐with‐smartassist. Image B from Texas Children’s Hospital Cardiac Catheterization Laboratories.) Table 34.7 Characteristics of and indications for the different Impella heart pumps ECMO, indicates extracorporeal membrane oxygenation; LV, left ventricle; OHT, orthotopic heart transplant; RV, right ventricle; VA, venoarterial; VAD, ventricular assist device. Although sedation has been used for femoral Impella placement, our usual strategy in patients not already intubated is to proceed with GETA. The usual considerations for high‐risk patients apply and include the availability of blood, vasoactive agents, adequate venous and dedicated arterial access, defibrillation pads, and the preprocedural determination of ECMO candidacy in the event of arrest. A surgical cutdown at the axillary artery with placement of a chimney graft adds to the duration of the procedure both due to the dissection and the time needed to pass the device through the graft. If the arterial line used for monitoring is on the same extremity as that used for axillary device placement, a brief period of dampening is to be expected. Arrhythmias during placement are common due to ventricular ectopy. TEE guidance is essential to diagnose complications such as deep placement within the LV that may entangle the mitral valve chordal apparatus, or incomplete coaptation of the aortic valve leaflets causing new insufficiency [128]. The Impella monitoring screen can help in assessing device placement once TEE is removed [129] (Figure 34.21). Once proper positioning is confirmed, the Impella is initiated between P0 and P9: the higher the number, the greater the revolutions per minute and therefore flow support. Anticipated hemodynamic changes include an overall increase in cardiac output with a decrease in native stroke volume and pulmonary capillary wedge pressure. Vasoactive support is usually not changed in this acute period as the RV may still need support to provide LV filling. Prior to returning to the ICU, a Swan‐Ganz catheter is commonly placed to guide ICU management for escalating or weaning support. Periprocedural complications include limb ischemia, vascular injury, bleeding, hemolysis, suction events, and device failure [125]. Anticoagulation is maintained with heparin through the device’s purge fluid, and if inadequate, systemic heparin is added. The use of ECMO in the CCL, still the most common type of MCS in children, warrants further discussion. For high‐risk patients who may require ECMO during the procedure, consideration should be given to scheduling the procedure in the lab that is most suited to ECMO. Such a lab is usually large, with adequate oxygen and air attachments to accommodate both the ECMO circuit and anesthesia machine. Moreover, the ECMO circuit should be easily positioned within the room, with unimpeded entry into and exit from the lab. Similar considerations apply for patients already on ECMO who need a diagnostic or interventional procedure. Extensive further discussion of MCS is presented in Chapter 37.
CHAPTER 34
Anesthesia for the Cardiac Catheterization Laboratory
Introduction
Diagnostic catheterization
Procedure overview
Endomyocardial biopsy
Pressure (mmHg)
Newborns
Older children
Oxygen saturation (%)
Right atrium
60–80
a wave
3–8
5–10
v wave
2–6
4–8
Mean
0–4
2–6
Right ventricle
65–75
Systolic
65–80
15–25
End diastolic
2–7
3–8
Pulmonary artery
65–75
Systolic
65–80
15–25
Diastolic
35–50
8–12
Mean
40–70
10–16
PA wedge
95–100
a wave
6–10
8–14
v wave
7–11
10–17
Mean
5–8
7–13
Left atrium
95–100
a wave
4–7
6–12
v wave
6–12
8–15
Mean
3–6
5–10
Left ventricle
95–100
Systolic
65–80
90–120
End diastolic
3–7
2–5
Aorta
95–100
Systolic
65–80
90–120
Diastolic
45–60
60–75
Mean
55–65
70–90
Flows
L/min/m2 BSA
Pulmonary (Qp)
3.5–5.0
3.5–5.0
Systemic (Qs)
3.5–5.0
3.5–5.0
Resistances
Woods units/m2 BSA
Pulmonary (Rp)
8–10
1–3
Systemic (Rs)
10–15
15–30
Pulmonary hypertension study
Flows
Resistances
Shunts
Revised definition (at rest)
Clinical groupsa
Precapillary PH
mPAP > 20 mm Hg
PCWP ≤ 15 mm Hg
PVRi ≥ 3 WU m2
1, 3, 4, and 5
Isolated postcapillary PH
mPAP > 20 mm Hg
PCWP > 15 mm Hg
PVRi < 3 WU m2
2 and 5
Combined precapillary and postcapillary PH
mPAP > 20 mm Hg
PCWP > 15 mm Hg
PVRi≥3 WU m2
2 and 5
Pulmonary hypertensive vascular disease
Biventricular circulations
mPAP > 20 mm Hg
PVRi≥3 WU m2
Cavopulmonary circulation
Mean TPG > 6 mm Hg
PVRi > 3 WU m2
Lower risk
Determinants of risk
Higher risk
No
Clinical evidence of RV failure
Yes
No
Progression of symptoms
Yes
No
Syncope
Yes
Normal (height/BMI)
Growth
Failure to thrive
I, II
WHO functional class
III, IV
Minimally elevated
Serum BNP/NT‐proBNP
Significantly elevated or rising
Normal RV systolic function
Minimal RA/RV enlargement
RV/LV ratio <1
Normal TAPSE
Echocardiography
RV systolic dysfunction
Severe RA/RV enlargement
Increased RV/LV ratio
Reduced TAPSE
Pericardial effusion
CI > 3.0 L/min/m2
mRAP < 10 mm Hg
mPAP/mSAP < 0.5
+ Acute vasoreactivity
Invasive hemodynamics
CI < 2.5 L/min/m2
mRAP > 15 mm Hg
mPAP/mSAP > 0.75
PVRi > 15 WU m2
Cardiac magnetic resonance imaging‐guided catheterization
Interventional catheterization
Device closure of cardiovascular communications
Shared considerations
Atrial septal defects
The preterm patent ductus arteriosus
Weight ≤ 2 kg at time of procedure
Weight > 2 kg at time of procedure
Airway and ventilatory management
Thermoregulation
Blood
Access
Sedation and other medication management
Ventricular septal defects
Coronary artery fistulas
Balloon dilation of cardiac valves
Pulmonary valvuloplasty
Aortic valvuloplasty
Balloon angioplasty and/or stent placement
Pulmonary arteries
Pulmonary veins
Right ventricular outflow tract stenting
Coarctation of the aorta
Patent ductus arteriosus stenting to augment pulmonary blood flow
Creation of cardiovascular communications
Balloon atrial septostomy
Palliation in pulmonary hypertension
Fontan fenestration creation
Transcatheter valve replacement
Transcatheter pulmonary valves
Transcatheter aortic valves
Hybrid procedures
Pericardiocentesis
Percutaneous mechanical circulatory support
Insertion site
Indications
Maximum flow (L/min)
Pump motor/catheter diameter (Fr)
2.5
Percutaneous femoral or axillary arteries
2.5
12/9r
CP
Percutaneous femoral or axillary arteries
4.3
14/9
5.0
Percutaneous femoral or via surgical graft onto the axillary artery
5.0
21/9
5.5
Percutaneous femoral or via surgical graft onto the axillary artery
6.2
19/9
RP
Femoral venous only
4.6
22/11
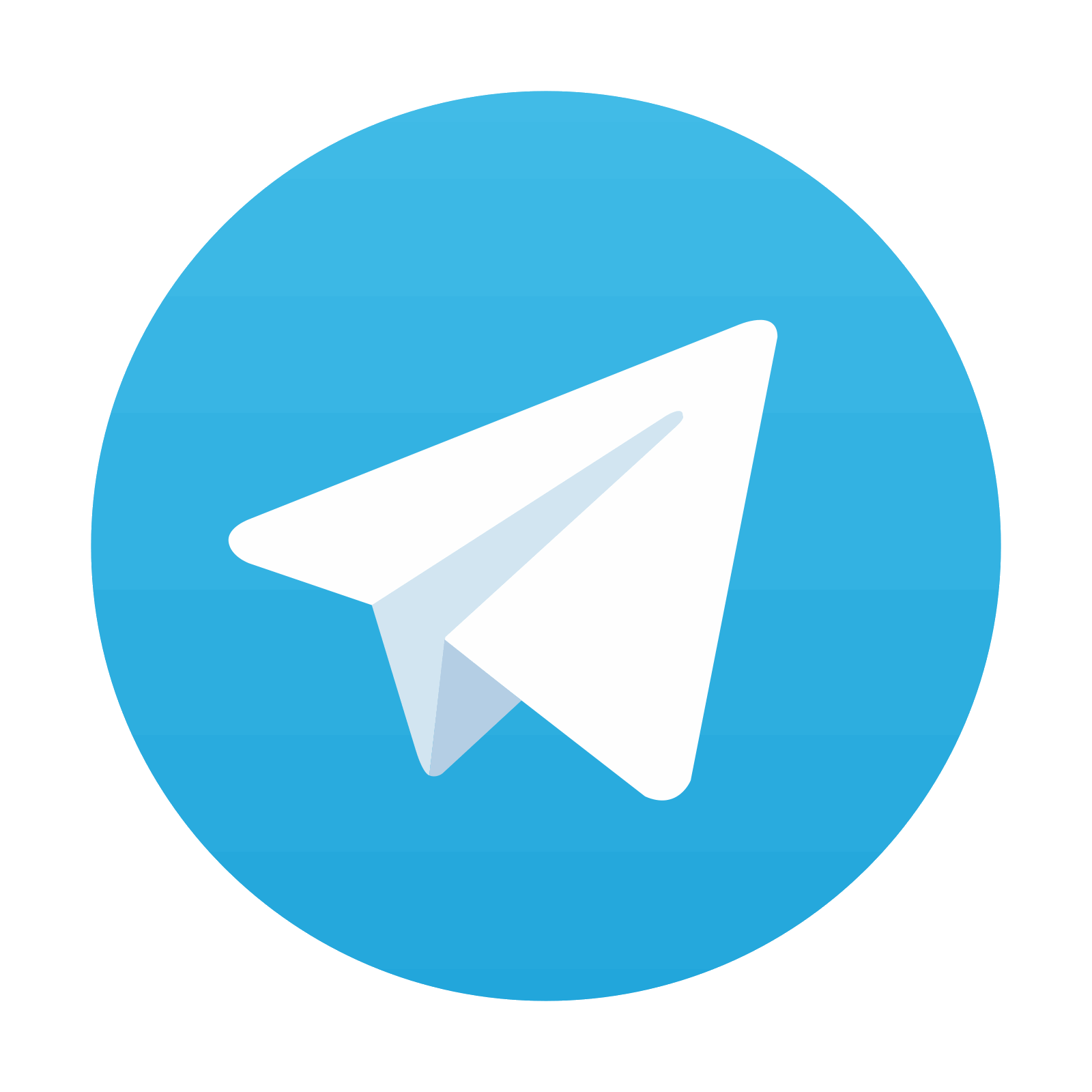
Stay updated, free articles. Join our Telegram channel
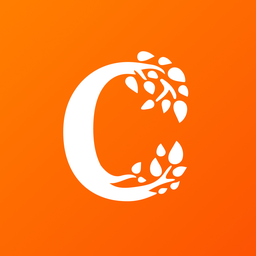
Full access? Get Clinical Tree
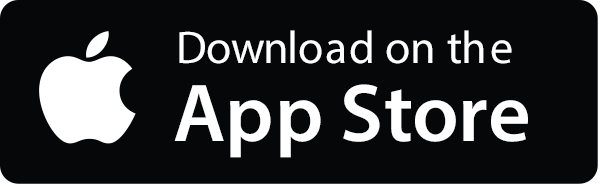
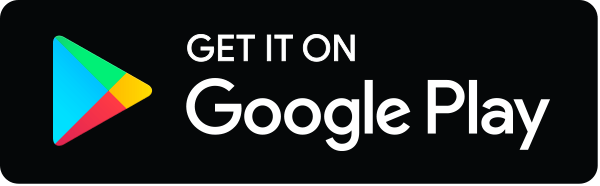