Mirela Bojan1 and Philippe Pouard2 1 Department of Anesthesiology, Congenital Cardiac Unit, Hôpital Marie Lannelongue, Groupe Hospitalier Paris‐Saint Joseph, Le Plessis Robinson, France 2 Head of Intensive Care, Anaesthesia and Perfusion Unit, Reference Center for Complex Congenital Heart Disease, University Hospital Necker Enfants Malades, René Descartes University, Paris, France Guidelines delineate limits within which decisions can be made regarding the management of specific clinical problems to improve outcome. A more prescriptive approach often takes the form of “goal‐directed” therapy, and the key to the success of any goal‐directed therapy is the selection of the specific goal [1]. A meta‐analysis of randomized studies of adult patients [2] in various postoperative settings demonstrated a strong relationship between the duration and magnitude of oxygen debt and outcomes in high‐risk patients. There was a significant improvement in survival when pulmonary artery catheter goal‐directed therapy was administered early or prophylactically (within 12–24‐hour postsurgery or before organ failure occurred) to increase the cardiac index and oxygen delivery (DO2). The aim of this goal‐directed therapy was intervention before hemodynamic compromise or critical organ system compromise occurred, because no amount of extra oxygen would rescue irreversible oxygen debt, organ failure, or cell death. Maintaining adequate tissue oxygenation, which is accomplished by optimizing oxygen transport balance, i.e., maintaining systemic DO2 and minimizing oxygen consumption (VO2), should prevent oxygen debt and improve outcome. In complex and high‐risk situations, such as following congenital heart disease (CHD) surgery, the use of evidence‐based clinical guidelines and associated goal‐directed therapy has the potential to improve clinical outcomes. Preventing oxygen debt is probably the ultimate goal when treating children who have undergone surgery for CHD. In 1995, Wernovsky et al. published a prospective study comparing deep hypothermic circulatory arrest and continuous low‐flow cardiopulmonary bypass in neonates undergoing the arterial switch operation [3]. The perfusion strategy assignment was not associated with the postoperative hemodynamics. However, the hemodynamic measurements performed on 122 patients revealed that during the first postoperative night, the cardiac index decreased by average 30% in almost one quarter of patients. Consequently, much effort has been put into augmenting CO, and the PRIMACORP study [4] demonstrating the benefit of the preventive use of a potent vasodilator to increase CO was a milestone in the field. Later on, using continuous respiratory mass spectroscopy to measure oxygen consumption, arterial, superior vena cava (SVC), and pulmonary venous saturations to measure DO2 in 14 neonates undergoing the Norwood procedure, Li et al. [5] reported low CO and systemic DO2 during the first 12 postoperative hours; VO2 was high postoperatively and progressively decreased over the first 24 hours. The decrease in VO2 during the first 24 h was the main contributor to improving the VO2/DO2 balance, pointing to the rationality of postoperative strategies to decrease VO2 in critical cases. A pathologic imbalance between VO2 and DO2 is thought to be the mechanism underlying anaerobic metabolism, multisystem organ failure, and death. The oxygen extraction rate (OER), calculated as might best reflect the oxygen consumption/delivery balance. The normal ratio of four to five times the amount of DO2 to VO2 suggests considerable reserve, and decreasing this factor (or increasing the OER) reflects less cardiovascular reserve. At high DO2, VO2 remains both stable and oxygen supply independent. During periods of reduced DO2, aerobic metabolism is maintained initially through increased oxygen extraction by the tissues. Through this mechanism, VO2 remains independent of DO2 until a critical OER is reached. At that point, which is generally thought to correspond to an OER between 0.5 and 0.6, [6], VO2 becomes oxygen supply‐dependent and drops linearly to zero if DO2 decreases further. An OER above 0.5 has a positive predictive value of 74% for postoperative death in infants following CHD surgery [7]. The inflection point between the two slopes of the DO2 curve indicates the critical level of DO2. An illustration of these relationships is shown in Figure 21.1. The relationship is altered further when VO2 increases. When VO2 increases, which is common in the postoperative period, the critical DO2 also increases, as shown in Figure 21.1, meaning that a VO2/DO2 imbalance occurs at higher DO2 levels. Therefore, it follows that one of the postoperative strategies is to increase DO2 to delay the point at which a VO2/DO2 imbalance may occur. Figure 21.1 Pathophysiological changes in the relationship between oxygen consumption (VO2) and oxygen delivery (DO2). (Source: Squara [8]. Reproduced with permission of Springer Nature.) The Fick equation states that where CO is the cardiac output, and CaO2 and CvO2 are the oxygen content of arterial and venous blood, respectively. At steady state, on the basis of the law of mass conservation, oxygen consumption and delivery must be equal. In the setting of CHD, DO2 is a resultant of several factors. In the absence of intracardiac shunting, CO is equivalent to the systemic output (QS) and the pulmonary output (QP). and systemic DO2 is a linear function of CO and CaO2: These indexes change slightly in patients with univentricular physiology, as described below [9, 10]. Following the first stage of the Norwood palliation, the systemic and pulmonary circulations become parallel, and the single ventricle assumes both the systemic and the pulmonary output: If making the assumption that the pulmonary oxygen uptake equals VO2, then one can write separately: and where CpvO2 is the pulmonary venous blood oxygen content. We can combine these equations as follows: which shows that DO2 is a complex function of CO, pulmonary venous oxygen content, VO2 and QP/QS ratio [9]. Following the bidirectional Glenn anastomosis, the blood that normally drains into the right atrium (RA) from the SVC is redirected to the pulmonary artery, and forms a combined upper systemic and pulmonary circulation, which is in parallel with the lower systemic circulation. The overall CO is a sum of the upper and lower systemic output: Since QP = QSVC, it results that On the other hand, states that the whole‐body VO2 is the sum of the upper body oxygen consumption (k × VO2) and that of the lower body [(1 − k) × VO2], where k is the fraction consumed by the upper body. If making the assumption that oxygen uptake through the pulmonary circulation equals VO2, then one can write separately: and We can combine these equations as follows: which shows that DO2 is a complex function of cardiac output, pulmonary venous blood oxygen content, lower body oxygen consumption and QSVC/ QIVC ratio [10]. To resume, albeit in a majority of patients, the postoperative DO2 is a linear function of CO and CaO2, there are cases where DO2 is a complex function of several other factors. Thus, if the goal is to balance postoperative VO2/DO2, then focusing on CO alone is probably not the right strategy. Changes in systemic oxygen balance have been well‐documented in adults and children undergoing surgery with cardiopulmonary bypass (CPB) [5, 11, 12], with VO2 increasing and DO2 decreasing within the first 8–12 hours postoperatively. Fortunately, increases in VO2 are usually closely paralleled by changes in DO2, and OER varies little in patients with stable cardiac function postoperatively [11]. Evidence suggests that 50% of the postoperative increase in VO2 in adults is due to inflammatory responses, and that variations in VO2 are strongly correlated with the circulating levels of endotoxin, tumor necrosis factor and interleukin‐6 concentrations [12]. Alterations in pulmonary function following cardiac surgery result in increased work of spontaneous breathing, which may require the redistribution of up to 15–20% of CO away from other organs [13]. Additionally, even when sedation and neuromuscular blockade was maintained to obviate the confounding effects of spontaneous ventilation, movements, agitation, and pain on VO2, Li et al. [11] demonstrated a mean 14.7% increase in VO2 within 4 hours of CHD surgery. The main determinants of this increase were a low age and an increase in central temperature, with an approximate 11% rise in VO2 per degree of increase in central temperature following hypothermic CPB [11]. The increase in VO2 is more substantial in children than in adults for several additional reasons. First, young infants have greater resting VO2 and experience greater inflammatory responses when compared with older patients with CPB. Deep hypothermia is more frequently used during pediatric cardiac operations, and active rewarming restores only 65% of the heat loss, with muscles and subcutaneous fat remaining hypothermic at the end of CPB [14]. Strong biochemical and hormonal stress responses occur in young children following cardiac surgery, further increasing VO2 [15]. Finally, treatments used in an effort to improve CO may have complex or even adverse effects. Catecholamines augment cardiac contractility and DO2 and also stimulate VO2 through their effect on myocardial work and metabolic rate [16]. If the increase in DO2 is greater than the increase in VO2, then catecholamines will improve overall balance of tissue oxygenation. In neonates, however, catecholamines have additional thermogenic actions through their effect on brown adipose tissue [17], resulting in an exaggerated increase in VO2 (Box 21.1). Equation (21.5) shows that DO2 decreases are a result of a reduction in CaO2, or a reduction in CO. Equation (21.5) can be rewritten as: The main determinants of CaO2 are the hemoglobin concentration and SaO2. The ideal hemoglobin concentration and management of perioperative transfusions largely depend on age and on the underlying CHD and will not be discussed further. Postoperative bleeding is a major risk factor of low cardiac output syndrome (LCOS), through on the other hand, hypoxemia following cardiac surgery is multifactorial. CPB and reperfusion result in an intense generalized inflammatory reaction and diffuse endothelial damage accompanied by an increased vascular permeability, both of which induce pulmonary injury. Alveolar and interstitial edema, which may or may not be clinically apparent, reduce dynamic lung compliance and increase the ventilation/perfusion mismatch. A reduction in functional residual capacity (FRC) after anesthesia and thoracic surgery may result in worsening of hypoxia. However, the major driver of a low postoperative DO2 is LCOS. LCOS is a common complication following CHD surgery in infants. Wernovsky et al. reported that 25% of neonates following the arterial switch operation showed a decline in cardiac index to <2 L/min/m2, which typically occurred between 6 and 18 hours after surgery [3]. The prevalence of LCOS in other studies was even higher, reaching 40% [19, 20]. This fall in the cardiac index was associated with an increase in systemic vascular resistance (SVR) of approximately 25%, and an increase in pulmonary vascular resistance (PVR) of nearly 40% over baseline values [3]. These findings were confirmed experimentally, showing a 15% decrease in CO occurring 2–4 hours after CPB in piglets [21]. The decrease in CO was concomitant with a 19% increase in PVR and an equivalent increase in systemic arterial elastance, both reversed by the preventive use of milrinone and levosimendan (which are systemic and pulmonary vasodilators). It appears that, by contrast with most of the adults, neonates and infants develop LCOS with high SVR and PVR following CPB. Under normal circumstances, increased afterload is accompanied by a compensatory increase in ventricular contractility to maintain the stroke volume, known as ventriculovascular coupling. During experimental postcardiac surgery LCOS, it has been shown that there is a lack of compensatory increase in ventricular contractility [21], and that therapeutic interventions aiming at reducing afterload actually prevented a reduction in CO. Figure 21.2 shows ventricular pressure–volume loops while varying afterload, preload, and inotropy. It shows that an increased afterload results in a decrease in the stroke volume and an increase in the workload, and thus VO2, of the ventricle. However, several myocardial stresses occur during cardiac surgery, including ischemia–reperfusion injury, inflammatory response, cardioplegia, and oxidative stress, and will invariably cause some degree of postoperative myocardial oxygen debt and dysfunction. Thus, an increase in the afterload creates a challenging situation, where the myocardium has to cope with an increased VO2 while recovering from oxygen debt. Evidence suggests that even patients undergoing atrial septal defect closure with short CPB and cross‐clamping durations suffer from left ventricle (LV) systolic dysfunction, with a decrease in end‐systolic elastance by 40% (Figure 21.3); this occurs independently of the type of cardioplegia and age [22]. Myocardial function is further impaired in patients undergoing complex repairs requiring ventriculostomies and large pericardial patches, in whom myocardial stunning is common. Myocardial dysfunction in neonates may be even more pronounced; neonates are known to have a relatively fixed stroke volume and their CO is known to be highly dependent on heart rate. They have diminished myocardial reserves to increase contractility, due to the immaturity of the neonatal myocardium: greater noncontractile component of myocardium, decreased norepinephrine stores, lower sarcoplasmic reticulum content, and high reliance on extracellular calcium regulation. In univentricular hearts, these reserves are marginal, and efforts to improve DO2 by intropic agents, which increase the myocardial VO2, are more likely to be associated with adverse effects. CPB results in early dysfunction of the pulmonary endothelium and an increase in PVR [23]. Endothelial dysfunction is a consequence of the systemic inflammatory responses, alongside ischemic insult to the parenchyma itself, which occurs as a consequence of exclusive bronchial perfusion during bypass. Bronchial perfusion is further reduced in deeply cyanosed patients, or in those who have major aortopulmonary collaterals, in whom a significant backflow from the aorta to the branch pulmonary arteries via the pulmonary circulation is observed. Endothelial dysfunction is accompanied by sequestration of activated neutrophils within the pulmonary microcirculation, capillary leak and increased extravascular water levels, reduced surfactant function, atelectasis, and an increased pulmonary shunt. Additional postoperative factors, such as pain, hypoxia, hypercarbia, and acidosis, may cause an increase in PVR, particularly in neonates. Increased PVR can be particularly problematic in neonates, in whom PVR is already high, and in young infants with increased preoperative pulmonary blood flow. Right ventricular (RV) failure due to acute pulmonary hypertension (PHT) is often associated with LCOS and hypotension which, added to the increased RV pressure, reduces the RV coronary perfusion pressure, and may further reduce RV contractility. Even transient pulmonary pressure overload may induce persistent RV failure. Figure 21.2 Ventricular pressure volume loops and effect of varying afterload, preload or inotropy on the stroke volume and work load. An increased afterload, as seen in post‐CHD surgery in infants, results in a decrease in the stroke volume and an increase in the workload of the ventricle, a challenging postoperative situation. Decreasing the afterload results in an increased stroke volume and an equivalent work load of the ventricle. Both the increase in preload and inotropy result in an augmented stroke volume, with a concomitant increase, however, in the workload of the ventricle. It appears that, in the context of post‐CHD surgery LCOS in infants, vasodilators increase the stroke volume without affecting myocardial oxygen demand, and represent, therefore, the most effective option. A: aortic valve closes, B: mitral valve opens, C: mitral valve closes, D: aortic valve opens. Figure 21.3 Left ventricular pressure–volume loops from infants and children undergoing closure of atrial septal defect with short bypass and cross‐clamping durations. (A) Pre‐bypass; (B) post‐bypass (Source: Chaturvedi et al. [22]. Reproduced with permission of Elsevier.) Finally, LCOS may develop due to various reasons specific to the underlying CHD and to the surgical procedure. Coronary diastolic perfusion may be decreased following a systemic–pulmonary anastomosis as seen following the Norwood procedure, and results in dysfunction of the single ventricle. Nearly, 50% of patients undergoing repair of tetralogy of Fallot have isolated diastolic RV dysfunction [24], and may develop LCOS due to insufficient preload of the LV. High PVR results in dysfunction of the cavo‐pulmonary anastomosis following a bidirectional Glenn or a Fontan procedure, to a decrease of the single‐ventricle preload, and to LCOS (Boxes 21.2 and 21.3). As mentioned before, the goal of the postoperative hemodynamic manipulation is the optimization of the VO2/DO2 balance. Sedation and analgesia lead to substantial falls in postoperative VO2 [11], which could suffice to maintain the VO2/DO2 balance in critical situations where it is not possible to further increase the DO2. Treatment of hyperthermia reduces the VO2 by 11% for every degree of reduction in body temperature [11]. Anti‐inflammatory strategies have been reported to have similar effects, probably by acting on both the inflammatory state and hyperthermia [25]. In addition, mechanical ventilation with muscle relaxation reduces VO2 by more than 20%, and as well as stabilizing pulmonary gas exchange, it can preserve the limited DO2 for other vital organs [13]. On the other hand, blood transfusions cause a significant increase in DO2; transfusion thresholds are specific to age and dependent upon the underlying CHD; and limitations are due to increases in blood viscosity and PVR. The major priorities, nevertheless, both intra‐ and postoperatively, are the prevention and early treatment of the LCOS, and this section will focus on the pharmacological treatment of LCOS. The pharmacologic optimization of CO in children with cardiac surgery involves the manipulation of systolic and diastolic myocardial function, preload, SVR, and PVR. The prospective study published by Wernovsky et al. in 1995 [3], including invasive hemodynamic measurements in 122 neonates undergoing the arterial switch operation, demonstrated an average 30% drop in cardiac index in almost one quarter of patients, while PVR and SVR increased. The lowest measurement typically occurred 9–12 hours after surgery. Evidence supporting preventive drug therapy for LCOS is derived primarily from the landmark PRIMACORP study [4], which reported a 55% reduction in the risk of postoperative LCOS after administration of high‐dose milrinone. As a result, preventive drug therapy for LCOS in at‐risk patients has become common practice, as revealed by two recent international surveys among pediatric cardiac centers [26, 27]. The ventricular pressure–volume loops shown in Figure 21.2 illustrate the possible therapeutic options to increase the stroke volume in case of a reduced CO with increased afterload. Increasing preload results in an increase in the stroke volume according to the Frank–Starling law, with a concomitant increase, however, in the workload of the ventricle. Ischemia–reperfusion injury and myocardial edema resulting from aortic cross‐clamping aggravates the physiological stiffness of the neonatal myocardium, and it follows that the beneficial potential of volume administration is limited by an excessive increase in the atrial pressure. Inotropic agents augment the stroke volume too, however, their benefit is limited by the concomitant increase in the workload, and thus VO2, of the ventricle. By contrast, vasodilators increase the stroke volume without affecting myocardial oxygen demand, and represent, therefore, the most effective option in the setting of postcardiac surgery LCOS in infants. In the pediatric cardiac setting, the use of cardiovascular drugs is often based on extrapolating the underlying pathophysiology and pharmacodynamics from adult studies, and on anecdotal experience. In 2008, Pasquali et al. published a survey on the use of off‐label cardiovascular medications in children as assessed by the Pediatric Health Information System [28]. The study included observations from 31,432 patients, median age 10.4 months, and reported that 78% of all patients received at least one cardiovascular drug off‐label. The risk of a neonate receiving at least one drug off‐label was 11% higher than that of an older patient. The most common cardiovascular drugs used off‐label were furosemide, epinephrine, dopamine, lidocaine, and milrinone. It is also important to note that there are no clinical trials that have produced recommendations with regards to dosing. Therefore, the suggested dose ranges here should be considered as “guides.” The drugs currently used for the acute hemodynamic management of CHD patients have inotropic, chronotropic, lusitropic, vasoconstrictor, systemic, and/or pulmonary vasodilator effects. Most of the cardiovascular drugs have mixed and dose‐dependent effects, therefore, a functional classification has been proposed, according to their inotropic and peripheral vascular effects into: inodilators, inoconstrictors, vasodilators, and vasoconstrictors [29]. The most commonly used agents are displayed in Table 21.1 and Figure 21.4, according to their potencies and dose‐dependent hemodynamic effects. Most cardiovascular agents in clinical use exert their cardiovascular effects by interacting with adrenergic receptors in the heart and vessels. Vascular α1‐adrenergic receptors increase SVR by constricting the mesenteric, skin, and renal arterioles while redistributing blood flow from the peripheral veins into the arterial circulation. Myocardial β1 receptors, and to a lesser extent, β2‐adrenergic receptors, increase HR and cardiac contractility. Vascular β2 receptors, and to a lesser extent, β1 receptors, oppose the vasoconstrictive effects of α1 receptors and reduce vascular tone, especially in the skeletal muscle. The location and effects of adrenergic receptors are shown in Table 21.2. Vasopressin 1a receptors (V1a) mimic and augment the vasoconstrictive effect of α1 receptors. Table 21.1 Classification of the most popular inotropic and vasoactive agents (Source: Adapted from Jentzer et al. [29].) Figure 21.4 Hemodynamic responses to vasoactive and inotropic drugs. (Source: Adapted from Jentzer et al. [29].) The most commonly used inotropic agents are β‐adrenergic agonists (catecholamines) and phosphodiesterase type 3 inhibitors. These agents exert positive inotropic and chronotropic effects by increasing the intracellular calcium concentration via an increase in cyclic adenosine monophosphate (c‐AMP) and activation of the protein kinase pathway in cardiac myocytes, as shown in Figure 21.5. c‐AMP, in turn, activates calcium channels and lead to increased cytosolic calcium, which improves the contractility of the actin–myosin system through its binding with troponin C. A newer class of inotropes is calcium sensitizers, which binds cardiac troponin C, stabilizes calcium‐induced conformational changes, and increases contractility by promoting a prolonged interaction between actin and myosin filaments. They do not increase cytosolic calcium concentrations. Table 21.2 Adrenergic receptor sites and physiologic actions (Source: From Latifi et al. [30].) Catecholamines play an important role in the management of LCOS after cardiac surgery. Endogenous catecholamines, such as dopamine, epinephrine, and norepinephrine, are synthesized from tyrosine, which undergoes several transformations to yield dopamine. A portion of the dopamine is then transported into vesicles from the cytoplasm where it is converted to norepinephrine, the main neurotransmitter in the human nervous system. The final step in the pathway, which converts norepinephrine to epinephrine, occurs in the adrenal medulla. Endogenous catecholamines are hormones and neurotransmitters that influence a multitude of physiological functions; however, synthetic catecholamines, such as dobutamine and isoproterenol, target‐specific receptors resulting in selective effects (Table 21.3). The half‐life of catecholamines is approximately 2–5 min, which allows for rapid titration. Since the breakdown of catecholamines by the catechol‐O‐methyl‐transferase and monoamine oxidase is ubiquitous, dosage adjustments are not necessary, even in patients with renal, hepatic, or any other organ dysfunction. Figure 21.5 Schematic representation of the postulated mechanism of action of β1‐agonists, milrinone and levosimendan in the myocardial cell. (Source: Gillies et al. [31]. Reproduced with permission of Springer Nature.) Table 21.3 Hemodynamic effects of the most popular vasoactive and inotropic drugs ATP: adenosine triphosphate; c‐AMP: cyclic adenosine monophosphate; PDE3: phosphodiesterase type 3; PDE5: phosphodiesterase type 5; PVR: pulmonary vascular resistance; SVR: systemic vascular resistance. Epinephrine has a potent agonist of α1‐, β1‐, and β2‐adrenergic receptors (Table 21.3), approximately 100‐fold more potent as an inotrope than dobutamine or dopamine. Low epinephrine doses (0.01–0.1 μg/kg/min) are used to increase stroke volume and to a lesser extent HR through a β2‐adrenergic effects. Combined with afterload reduction through peripheral vasodilatation, low epinephrine doses increase CO, and is therefore recommended in patients with severe systolic dysfunction. At somewhat higher concentrations, β1‐adrenergic receptors are activated resulting in increased myocardial conduction, increased sinoatrial node discharge, increased force of contraction, and increased rate of rise of the intramural pressure. High concentrations of epinephrine may produce serious atrial and ventricular dysrhythmias if the myocardium is sensitized by infarction, surgery, or myocarditis. Higher epinephrine doses (>0.1 μg/kg/min) produce increasing α1‐receptor‐mediated vasoconstriction. This results in increased afterload and increase the myocardial workload, which are undesirable early after CPB, and may be detrimental in infants with functionally univentricular circulation. Even though epinephrine constricts renal and cutaneous arterioles, renal function and skin perfusion may improve due to increased CO. However, high infusion rates (>1–2 μg/kg/min), conditions in which the α1‐adrenergic responses predominate, may exacerbate multiorgan system failure. Figure 21.6 Simulation of the relationship between different epinephrine concentrations and hemodynamic responses in children with different ages and weights. (Source: Oualha et al. [33]. Reproduced with permission of Springer Nature.) Epinephrine is largely used in the postoperative management of CHD [26, 32], although evidence‐based data are currently lacking, and dosing regimens are extrapolated from adult studies. However, the amplitude of the hemodynamic response to epinephrine is difficult to predict. Although the effect of epinephrine is primarily dependent on the plasma concentration, a recent report shows that both age and body weight may play a role in the large between‐subject pharmacokinetic and pharmacodynamic variability observed in children undergoing CHD surgery [33]. The smaller the patient, the smaller the increase in heart rate and blood pressure for a given epinephrine concentration and severity of illness, as shown in Figure 21.6 [33]. It is likely that the postnatal development of the myocardial contractility is associated with changes in the modulatory effects of β‐adrenergic receptor signaling. During cardiopulmonary resuscitation, the α1‐adrenergic‐mediated vasoconstriction induced by epinephrine increases aortic diastolic pressure and, thus, coronary perfusion pressure, which is a critical determinant of successful resuscitation from cardiac arrest. The American Heart Association‐recommended dose of epinephrine for children experiencing bradycardia, asystole, or pulseless arrest is 0.01 mg/kg intravenously, which may be repeated at 3–5‐min intervals, up to 1 mg per dose [34]. Dopamine stimulates the dopaminergic D1 (postsynaptic) and D2 (presynaptic) receptors located in several vascular beds; it also binds α‐ and β1‐adrenergic receptors, although with lower affinity (Table 21.3) doses (> 8 μg/kg/min) induce vasoconstriction via α1‐adrenergic receptor stimulation. Increased PVR has been reported with dopamine doses higher than 7.5 μg/kg/min. The sensitivity of infants to dopamine is controversial, and neonates may exhibit a clinical response to doses as low as 0.5–1 μg/kg/min [30]. Li et al. [35] studied the effects of 5 μg/kg/min of dopamine in neonates undergoing the Norwood procedure. Dopamine did not increase DO2 and seemed to cause an increase in myocardial VO2. A dopamine infusion of 5 μg/kg/hour had a significant thermogenic effect, and termination of the infusion was associated with a substantial 20% reduction in the total VO2 [35]. Dobutamine is administered as a racemic mixture of two compounds, namely, a strong β1‐agonist, and a weak α and β2 agonist. The end result is a drug with broad receptor specificity, a strong dose‐dependent increase in stroke volume, heart rate and CO, and a variable effect on arterial pressure (Table 21.3). It modestly lowers the SVR, except at high doses (> 10–15 μg/kg/min) when dose‐dependent α1‐adrenergic receptor agonism may become more prominent. Low doses, up to 5 μg/kg/min, increase stroke volume without significant tachycardia, however, doses above 10 μg/kg/min produce worsening tachycardia with minimal further increase in CO due to declining stroke volume from decreased diastolic filling time. Dobutamine significantly increases the myocardial VO2 and is frequently used to stress the myocardium. Comparison with phosphodiesterase inhibitors demonstrates similar improvement in stroke volume, although dobutamine induces a more profound decrease in LV filling pressures and vascular resistance [36]. Dobutamine induces a greater increase in heart rate than milrinone, and the beneficial increase in coronary blood flow may be outweighed by an increase in myocardial VO2 [36]. Higher doses of dobutamine (>15 μg/kg/min) can predispose to the development of atrial or ventricular arrhythmias. Despite its widespread use, the exact role of dobutamine in pediatric therapeutics remains unclear, and its use following cardiac surgery remains controversial because of excessive tachycardia, arrhythmias, and variable effect on SVR. Norepinephrine is a potent α1‐receptor agonist and mild β1‐agonist with minimal β2 activity. Increasing doses of norepinephrine may increase CO in some patients through augmentation of venous return, however, it may reduce CO due to the strong increase in afterload in patients with cardiac dysfunction. Norepinephrine reduces myocardial VO2, due to a reflex reduction in heart rate. By increasing diastolic pressure, norepinephrine increases coronary perfusion. Norepinephrine is mainly used as a vasopressor and will be discussed later. The use of catecholamines comes at a price, namely to place continual stress on an organ that is already failing, and placing an increased demand for oxygen at a time when supply is critically limited. Catecholamines increase the myocardial VO2 through their inotropic and chronotropic effects and induce arrhythmogenesis. These are due to an increase in intracellular calcium concentration, produced by an increase in c‐AMP. This is particularly problematic in the neonatal myocardium, in which the functional reserve is limited and which operates under already maximal adrenergic stimulation. If such an increase is not accompanied by a proportional increase in coronary blood flow, a mismatch between myocardial VO2 and DO2 may result. When inotropes are used for the management of adults with acute heart failure, there is an increase in in‐hospital mortality when compared with management without inotropes [37]. Results from the European Society of Cardiology Heart Failure Long‐term registry have shown a detrimental association between the use of inotrope and/or vasopressor and both in‐hospital and long‐term mortality [38]. Both dopamine and epinephrine were associated with the highest risk of death in adults [38, 39]. The recent guidelines of the European Society of Cardiology for the treatment of acute heart failure restrict the use of inotropic agents to patients with symptomatic hypotension and poor perfusion [40]. For many years, it has been acknowledged that chronic exposure to catecholamines can exert a toxic effect on the myocardium and increase apoptosis. In vitro studies in cultured cardiac myocytes show that tonic exposure to norepinephrine increases the number of apoptotic myocytes via stimulation of the β1‐adrenergic receptor pathway [41]. Although the link between myocardial apoptosis and myocardial failure remains to be proven, these observations provide a rational to limit the use of β1‐agonists following cardiac surgery. In addition, the variability in the pharmacologic effect of β‐agonists in neonates makes their use very challenging in this age group. Studies of myocardial biopsies identified an adult‐like myocardium, with low‐density and high‐affinity β‐adrenergic receptors, and a fetal‐like myocardium, with high‐density and low‐affinity β‐adrenergic receptors [42]. Neonates had the highest density and the lowest affinity β‐adrenergic receptors of all, and they have lower affinity β receptors in the LV when compared with the RV (whereas immature and adult hearts did not have ventricular differences) [42]. Additionally, experimental studies demonstrated downregulation of the LV β‐adrenergic receptors during chronic hypoxemia [43]. Long‐term exposure of myocardial β‐adrenergic receptors to circulating endogenous or exogenous catecholamines leads to receptor desensitization, which is mediated by uncoupling of the receptor–effector system and by a decrease in receptor density. In patients with severe cardiac failure, β1‐adrenergic receptor density is selectively reduced due to exposure to endogenous norepinephrine. It has been shown that the severity of the left‐to‐right shunt and of PHT is correlated with norepinephrine levels in pediatric CHD patients [44]. Consistently, children with severe CHD were found to have an up to 50% reduction in the number of right auricle β‐adrenergic receptors, with the lowest receptor densities found in critically ill neonates with congenital aortic stenosis and transposition of the great arteries [45]. Catecholamines increase the overall metabolic rate, which manifests as an increase in the overall VO2. β1, β2, and particularly β3‐adrenergic receptors, as well as dopaminergic receptors are densely expressed at high levels in brown adipose tissue, which is abundant in human neonates, where it plays a crucial role in nonshivering thermogenesis. Catecholamines, especially norepinephrine and dopamine, simulate heat production by stimulating fatty acid oxidation and uncoupling the respiratory chain from adenosine triphosphate (ATP) synthesis; thus, neonates may be particularly susceptible to the metabolic effects of catecholamines, which may increase VO2 to much higher levels than in older patients. Catecholamines also accelerate aerobic glycolysis and induce insulin resistance, a phenomenon that is exacerbated in younger patients, resulting in a concentration‐dependent increase in glycemia and lactate production, as shown in Figure 21.7 [33]. Figure 21.7 Time‐course simulations of epinephrine concentration, plasma glucose and lactate levels following 0.02–0.25μg/kg/min epinephrine infusions in a child weighting 5 kg. (Source: Oualha et al. [33]. Reproduced with permission of Springer Nature.) In view of the aforementioned challenges, the use of β‐adrenergic receptor agonists for the prevention and/or management of LCOS following CHD surgery should be questioned, particularly considering that the evidence of their use is based solely on pharmacological action rather than an assessment of meaningful clinical outcomes. Bipyridines were developed in the 1980s to improve myocardial contractility in the chronically failing ventricle. They selectively inhibit intracellular cardiac phosphodiesterase type 3, thereby slowing the degradation of c‐AMP. This results in a positive inotropic effect through increased intracellular calcium levels during excitation–contraction coupling, and a positive lusitropic effect through faster sequestration of calcium that is released into the sarcoplasmic reticulum due to the concomitant activation of phospholamban (Figure 21.5). Vasodilation occurs in both arterial and venous vessels, via increased c‐AMP and opening ATP‐sensitive potassium channels in vascular smooth muscle cells (Figure 21.8). Arrhythmogenicity is mediated by an increase in intracellular c‐AMP and calcium levels. No tachyphylaxis is observed. Amrinone, the prototype bipyridine, acts mainly as a vasodilator, and has some inotropic effects [47–49]. Amrinone is metabolized in the liver via N‐acetylation and is then excreted in the urine; slow acetylators show a two‐fold increase in the half‐life, compared with that of rapid acetylators [50]. The half‐life is about 2 hours, and clearance is reduced in patients with hepatic and renal failure. Reversible dose‐dependent thrombocytopenia is common during prolonged therapy. The common dosage is 5–10 μg/kg/min. Amrinone is now rarely used since milrinone became available. Milrinone is the most popular bipyridine drug, and it is the one that has been subjected to careful evaluation in the placebo‐controlled multicenter PRIMACORP trial [4]. It has a half‐life of about 1 hours, which decreases linearly with age and increases in the presence of renal failure [51]. The drug shows inotropic and vasodilatory effects, although it appears that the increase in CO is mainly related to the vasodilatory effect [52]. Chang et al. reported that, when given to neonates with LCOS, milrinone increased the cardiac index from 2.1 to 3.1 mL/min/m2, concomitant with a reduction in filling pressures, in SVR by approximately 30%, and in PVR by about 25% [53]. These improvements in global hemodynamics were associated with an improvement in the myocardial VO2/DO2 ratio [53]. The multicenter PRIMACORP trial [4], including 238 neonates and infants demonstrated that the prophylactic administration of high‐dose milrinone (i.e., a bolus of 75 μg/kg, followed by an infusion of 0.75 μg/kg/min) decreased the incidence of LCOS from 25.9% to up to 11.7%, whereas a low dose (a 25 μg/kg/min bolus followed by a 0.25 μg/kg/min infusion) had no effect, when compared with placebo. In an experimental study under constant ventricular leading conditions, milrinone only very slightly increases heart rate and stroke volume [52]. Thus, unlike dopamine, milrinone does not increase myocardial VO2 [36] nor myocardial OER (17). Instead, milrinone reduces coronary vascular resistance, suggesting that it exerts a protective effect on the myocardium. Furthermore, the positive lusitropic properties make milrinone a useful drug when LCOS results from diastolic ventricular dysfunction. The pulmonary vasodilator effect of milrinone has been demonstrated in infants with PHT, and it is largely dependent on the degree of pre‐existing PHT and the severity of ventricular dysfunction [53]. Milrinone induces pulmonary vasodilatation by increasing pulmonary vascular c‐AMP levels, and, possibly, by attenuating the release of inflammatory cytokine [54]. Hypotension is a commonly reported side effect, occurring particularly during the administration of the loading dose. Ensuring an adequate preload can minimize it. The incidence of tachyarrhythmia increases up to three‐fold when using common dosing regimens of milrinone (a loading dose of 50 μg/kg, followed by a 0.25–1 μg/kg/min continuous infusion), independent of the concomitant use of catecholamines and of disease severity. As a result, many centers use milrinone without a loading dose, and begin the infusion by the end of CPB. However, a recent pharmacokinetic study of milrinone in a wide pediatric age range demonstrated that age, disease, and surgery differently impact the pharmacokinetics of milrinone, and that current milrinone dosing for LCOS is suboptimal to maintain the therapeutic target range across the entire pediatric age range [55]. It is likely that hypotension and arrhythmogenesis are responsible for the huge variability in the dosing regimens used at different centers [32]. Thrombocytopenia is less common (4%) and is seen when the infusion duration exceeds 3 days [56]. Milrinone is the drug most commonly used for the prevention and treatment of LCOS in CHD surgery [26, 27, 32]. However, the recent Cochrane meta‐analysis on its prophylactic use following CHD surgery [57] yielded a very disappointing conclusion indicating a lack of evidence of effectiveness on the incidence of LCOS and on mortality. For methodological reasons, the meta‐analysis excluded many valuable reports, and included only 393 participants from a mixed population aged 0–12 years. However, because of their specific physiology, including noncompliant ventricles and a high baseline sympathetic tone [58], neonates and young infants are the most likely to develop LCOS with high SVR and PVR, and to benefit from prophylactic milrinone. Levosimendan, a pyridazinone–dinitrile, binds cardiac troponin C and stabilizes calcium‐induced conformational changes, which, in turn, promotes the prolonged interaction between actin and myosin filaments during systole, and increases myocardial contractility, as shown in Figure 21.5 [59]. The resulting increase in contractility is unmatched by milrinone [21]. Levosimendan has vasodilatory effects, which are mediated by opening ATP‐sensitive potassium channels in the systemic, pulmonary, and coronary vascular smooth muscle cells [59–61]. In adult patients, levosimendan is responsible for a 45% increase in the coronary blood flow and a 9% reduction in myocardial oxygen extraction [62]. The impact of levosimendan on mitochondrial functions prevents calcium overload during ischemia–reperfusion injury and protects the myocardium against damage [63]. Levosimendan reduces the time constant for diastolic relaxation and improves diastolic function [21]. By improving the diastolic function, it is of particular interest when LCOS is due to LV or RV diastolic dysfunction, a common finding in patients with residual obstruction of the left or right outflow tract. It is also of particular interest in neonates in whom the ventricles are less compliant. Levosimendan has weak pulmonary vasodilator effects in the normal circulation [64], but has a potent effect in preconstricted pulmonary circulation [60]. In addition, it may attenuate endothelin‐1‐induced vasoconstriction, which would be of particular relevance in the post‐CPB setting [61]. Experimental work demonstrated an increase in the RV myocardial efficiency when levosimendan was used to treat RV hypertrophy and failure [65], and it has been proposed for the treatment of RV failure in patients with PHT [66]. When given after cardiac surgery in adults, levosimendan is associated with an approximately 50% reduction of the risk of acute kidney injury and renal replacement therapy [67]. Finally, levosimendan improves the recovery of diaphragmatic dysfunction and increases the chances of successful weaning from mechanical ventilation [68]. The pharmacokinetic profile of levosimendan, which is unaffected by age, makes it highly attractive for clinical use. The recommended dosage is an intravenous (i.v.) bolus of 12 μg/kg over 10 min, followed by a 2 μg/kg/min continuous infusion. Its biotransformation in the intestinal tract gives rise to an intermediate metabolite, OR 1,855, which is inactive, and which is further metabolized in the liver by acetylation into OR 1,896. OR 1,896 is a long‐lasting active metabolite whose concentration peaks at 36 hours after a 24‐hour infusion and remains stable up to Day 8; it is still detectable up to 12 days after levosimendan withdrawal [69]. Dosing adjustments are not required in patients with mild hepatic or renal failure. A randomized controlled study of milrinone and levosimendan conducted in neonates [69] showed that patients in the milrinone group had a greater requirement for other inotropes during the first hours postsurgery. Cerebral regional saturation monitoring suggested that both drugs caused a time‐dependent improvement in blood flow and oxygen availability, but infants on levosimendan showed significantly higher peripheral intravascular oxygenation and a lower difference in the central‐to‐peripheral temperature gradient [69]. Another randomized controlled trial in neonates undergoing complex surgeries reported an almost 40% decrease in the incidence of LCOS when compared with more conventional milrinone and epinephrine infusions, along with a significant reduction in lactate concentrations 6 hours postoperatively [70]. In patients younger than 5 years undergoing cardiac surgery, levosimendan given postoperatively was at least as efficacious as milrinone, and did not increase myocardial VO2 at 24 and 48 hours postoperatively [71]. Troponin values were significantly lower in the levosimendan group, suggesting a cardioprotective effect [71]. Because levosimendan does not increase the intracellular c‐AMP and calcium concentrations, tachycardia and hypotension are less severe than that reported for milrinone, although common during the loading dose [69, 71]. In contrast to those of catecholamines, the effects of levosimendan are not attenuated in patients with chronic heart failure, in whom β‐adrenergic receptors are downregulated, or by concomitant use of β‐blockers. The development of tolerance has not been reported to date. There are currently no official indications regarding the use of levosimendan in patients less than 18 years of age. As a matter of fact, the drug has been studied and used as a rescue therapy both in the pediatric intensive care unit (PICU) and the operating room. The pediatric experience with levosimendan given prophylactically for cardiac surgery in children less than 5 years was summarized in a recent meta‐analysis [72], which, for methodological reasons, only included five randomized controlled trials with a total of 212 participants. Compared with standard inotrope treatments, levosimendan did not reduce mortality or the incidence of LCOS; however, levosimendan significantly reduced time to extubation and length of stay and reduced the risk of circulatory support and of heart transplantation. Levosimendan has potential appeal in children with CHD and may prove to have an important role in the prevention and early treatment of LCOS in high‐risk patients after cardiac surgery; however, its application warrants more detailed investigation. Norepinephrine has potent α‐ and β1‐adrenergic receptor agonist activity, but little β2 activity, as shown in Table 21.3. Therefore, infusion of norepinephrine results in increased SVR because the α‐adrenergic stimulation is not opposed by β2‐adrenergic stimulation. As a vasopressor, norepinephrine is 100‐fold more potent than dopamine and three to five times more potent than epinephrine. Norepinephrine is used in the pediatric setting of shock with profound hypotension; it should be administered after intravascular volume repletion, and its use is best guided by knowledge of the CO and SVR. Although more commonly used in adults, a vasodilatory inflammatory response to CPB may be present in some children early after surgery and is often responsive to norepinephrine. Norepinephrine may also enhance coronary flow in patients with diastolic run‐off after systemic‐to‐pulmonary shunt. The dosage is usually titrated to mean or diastolic arterial pressure, and low doses (0.01–0.05 μg/kg/min) should be sufficient for hemodynamic stabilization. However, injudicious use of norepinephrine compromises blood flow within organs, for example, renal, splanchnic, and hepatic blood flow is reduced in healthy individuals. Infusion of norepinephrine may increase blood pressure, and yet not improve clinical indices of perfusion, and this type of poor clinical response is usually associated with a persistent low cardiac index. Importantly, the pharmacokinetics of norepinephrine in children is not well known. Norepinephrine should be titrated and used at the lowest dose to maintain the mean arterial pressure; doses > 0.5–1 μg/kg/min are considered very high. Arginine vasopressin (AVP) plays a vital role in the maintaining normal arterial blood pressure by regulating venous capacitance and arterial resistance. Vasopressin acts on V1a receptors in the peripheral vasculature to cause intense vasoconstriction through activation of protein kinase C (Table 21.3), ultimately leading to an influx of intracellular calcium. The rationale behind the use of exogenous AVP in the critically ill patients is that neurohypophyseal stores of AVP may become exhausted, leading to vasodilatory shock [73]. Another theoretical advantage of AVP is that it does not rely on adrenergic receptors, which may be downregulated in the presence of chronically elevated catecholamines. Also, signal transmission via adrenergic receptors may be impaired under conditions of metabolic acidosis. AVP depletion following CHD surgery has also been reported in children [74], as well as good response to the vasopressor AVP infusion ranging from 0.0001 to 0.002 U/kg/min in neonates, infants, and children with low postoperative blood pressure and adequate cardiac function [74, 75]. More recently, it has been suggested that lower doses of AVP 0.0003 U/kg/min initiated in the operating room could be beneficial in neonates with complex surgeries, such as the Norwood procedure or the arterial switch operation, to decrease the requirement for fluid resuscitation and catecholamines, and shorten the duration of intensive care stay [76]. A recent meta‐analysis showed that AVP did not confer a survival advantage in adults suffering from vasodilatory shock [77]. Also, safety data for AVP are lacking; a prospective randomized study of critically ill children requiring prolonged mechanical ventilation showed that AVP was associated with an increased incidence of oliguria and hyponatremia [78]. Phenylephrine is a pure peripheral α1‐adrenergic receptor agonist, as shown in Table 21.3, and is used as a bolus or infusion to treat acute cases of low systemic blood pressure or SVR. The pure α1 effects can result in reflex slowing of the heart rate, although this is not as pronounced in young infants as it is in adults. Its main use in CHD is to acutely raise SVR in cases where either ventricle is compromised by outflow obstruction. Such is the case of tetralogy of Fallot, where low SVR lead to increased right‐to‐left intracardiac shunting and cyanosis, and in hypertrophic cardiomyopathy or other left‐sided lesions, in which the gradient across the obstruction is increased by low SVR. A bolus dosing of phenylephrine, such as used on CPB to increase perfusion pressure, is 0.5–5 μg/kg, whereas infusion doses range from 0.02 to 0.3 μg/kg/min. The tolerance of systemic vasodilators is affected by the intravascular status, or by any actual or potential outflow tract obstruction; in such cases, systemic vasodilatation may have disastrous consequences. Careful imaging should be performed to exclude outflow tract obstruction in case of a doubt, prior to administering the vasodilator, and a very low dose or a short acting agent should be given first. Nitric oxide donors mimic the effect of endogenous NO, and by activating guanylate cyclase they lead to increased cyclic guanosine monophosphate (c‐GMP) production and vasodilation (Table 21.3). Low‐to‐moderate doses of nitroglycerin (3 μg/kg/min) increase venous capacitance without affecting the arterial resistance vessels [79]. As a result, ventricular filling pressures fall, whereas the stroke volume is unaffected. High‐dose nitroglycerin and nitroprusside increase venous capacitance and reduce SVR; thus, filling pressures fall and the stroke volume and CO rise significantly. Major indications for the use of nitroglycerin include myocardial ischemia, systemic hypertension, volume overload, congestive heart failure, and pulmonary edema. The net effect is usually an improvement in the myocardial VO2/DO2 ratio. Efficacy has been demonstrated following CHD surgery [80]. Tolerance occurs after more than 24 hours of i.v. therapy, and abrupt weaning after prolonged i.v. infusions may result in rebound hypertension. Extremely rare side effects are methemoglobinemia and cyanide toxicity, which result from the release of nitrite ions when the drug is metabolized. Phenoxybenzamine is a long acting α1‐ and α2‐adrenergic receptor blocker, as shown in Table 21.3, and is advocated by some groups for the routine perioperative management of infants undergoing cardiac surgery involving hypothermic CPB. It has gained popularity for infants undergoing the Norwood operation, in whom systemic vasoconstriction occurs additionally in response to failing systemic perfusion (such as seen at SaO2 > 80%). Such a situation was traditionally managed by manipulating the medical gases to increase PVR and lower SVR. Hoffman et al. carried out a prospective study of phenoxybenzamine use following the Norwood operation [81], where patients received 0.25 mg/kg phenoxybenzamine at the start of CPB, followed by 0.25 mg/kg/24 hours for up to 48 hours postoperatively if the target SvO2 > 50% and target QP/QS ratio of 0.8–1.2 were not reached. There was no evidence of hemodynamic deterioration at high SaO2 [81], suggesting that α‐adrenergic receptor blockade is an effective approach to modifying the intense sympathetically mediated vasomotor responses in these infants. However, the irreversible α‐receptor blockade and prolonged effect has limited its popularity. Phentolamine is a reversible α‐adrenergic receptor blocker. Its use is justified by the observation of elevated plasma catecholamine concentrations and increased SVR following CPB. A randomized trial of phentolamine in CHD surgery reported that administration of 0.2 mg/kg of phentolamine during cooling and rewarming for cardiac surgery reduced plasma lactate levels, indicating better tissue perfusion; in addition, the nasopharyngeal–rectal temperature gradient decreased four‐fold, whereas systemic VO2 increased [82]. Hydralazine dilates precapillary arterioles. Most studies of hydralazine were performed in the 1980s, in which the drug was used to treat hypertension following CPB in adults. Hydralazine can be used to treat postoperative hypertension in children (1–5 μg/kg/min), or in other situations that require a reduction in preload, such as severe myocardial dysfunction and extracorporeal membrane oxygenation (ECMO). However, the vasodilatory effect is limited by reflex tachycardia; therefore, β‐adrenergic receptor blockers and sodium nitroprusside are preferred. Nesiritide is a recombinant form of human B‐type natriuretic peptide, which is normally produced by the ventricular myocardium. It binds with the A‐ and B‐type natriuretic peptide receptors of the vascular endothelium and smooth muscle, which leads to increased c‐GMP production and vasodilation. It increases the glomerular filtration rate and inhibits sodium reabsorption, exerting a strong diuretic effect. Nesiritide was first believed to be beneficial for the treatment of acute decompensated congestive heart failure. Although it has also been found beneficial for the prevention of acute kidney injury following cardiac surgery in adults [83], the results of the largest study performed to date showed that nesiritide does not affect mortality or prevent rehospitalizations in adults [84]. No major hemodynamic effect has been reported when used after CHD surgery [85], but an improved urine output. The recommended dose range for infusion in infants and children with heart disease is 0.01–0.03 μg/kg/min. In the early 1990s, PHT was a major cause of post‐CHD surgery mortality [86]. Overall survival has improved since then and the reported incidence of severe PHT (defined as a ratio of pulmonary‐to‐systemic arterial pressure >1) has dropped to 2% [87], most likely due to a better understanding of the pathophysiology of PHT, earlier surgical correction in patients at high risk of PHT, and refinement of intraoperative techniques, including ultrafiltration. It is accepted that an important pathological characteristic of pulmonary vascular reactivity in patients with CHD is dysfunction of the pulmonary endothelium [88]. Such dysfunction suppresses the intrinsic endothelium‐dependent vasodilatory mechanisms, including the nitric oxide c‐GMP system. c‐GMP is generated by the interaction between NO and guanylate cyclase, and it is the final messenger in the pathway that mediates vascular smooth muscle relaxation. It is metabolized by the phosphodiesterase type 5, which is the major phosphodiesterase in the lung; phosphodiesterase type 5 expression is upregulated in patients with PHT and after CPB, causing increased turnover of c‐GMP. A schematic representation of the mechanism of action of pulmonary vasodilators is shown in Figure 21.8. Figure 21.8 Schematic representation of the postulated mechanism of action of pulmonary vasodilators in the endothelial cell. (Source: Adapted from Ghofrani et al. [46].) Pulmonary vasodilators are commonly used to lower PVR and to treat acute postoperative PHT in patients with preoperative pulmonary overcirculation, or pulmonary venous obstruction. In addition, children undergoing cavopulmonary connections for single‐ventricle lesions require low PVR for surgical success. Finally, pulmonary vasodilators are used to reduce the RV afterload, which is often increased following heart transplantation [89]. Historically, the standard therapy for lowering PVR included the induction of respiratory alkalosis due to the known effects of pH on pulmonary artery pressure and PVR; however, although hyperventilation reduces PVR, this comes at the expense of a subsequent increase in SVR and reduction in CO [90]. Other factors are used to lower PVR, including sedation, lung recruitment to improve oxygenation, and metabolic alkalosis. Inhaled nitric oxide (iNO) has been used for more than two decades to selectively reduce PVR. iNO produces pulmonary vasodilation in ventilated lung regions by activating endothelial guanylate cyclase, resulting in increased levels of c‐GMP, which, in turn, relaxes pulmonary vascular smooth muscle. iNO crosses the alveolar–capillary membrane leading to immediate pulmonary vasodilation. High‐affinity binding and immediate inactivation of iNO activity by hemoglobin limits the action of the drug in the pulmonary circulation. An early study by Miller et al. reported that iNO concentrations as low as 2 ppm reduced PVR by approximately 40%, and improved CO by approximately 15% [91]. The pulmonary/systemic artery pressure ratio predicts the response to iNO, with a greater response seen in those with a high ratio (≥0.50). The use of iNO has become more common, and a randomized double‐blinded study demonstrated its usefulness as a prophylactic treatment in patients at risk of PHT: patients treated with iNO suffered fewer PHT episodes and could be extubated more quickly [92]. Morris et al. [90] demonstrated that iNO was as effective as hyperventilation for reducing PVR; however, unlike hyperventilation, iNO did not increase SVR or reduce CO. iNO significantly improves oxygenation, lowers the transpulmonary gradient by 40–50%, and reduces central venous pressure by 15–20% in hypoxic patients undergoing Fontan‐type procedures [93]. There are inherent problems with iNO, which may limit its use. These include a suboptimal response in some patients, the development of rebound PHT upon withdrawal after even relatively short periods of use, and the mode of delivery, which generally, though not necessarily, requires endotracheal intubation. Moreover, the individual responses to iNO are variable. Abrupt withdrawal of iNO or rapid reductions in the dose may lead to rebound PHT [94], even in patients with no pre‐existing PHT. Rebound is due to the downregulation of endogenous NO synthase in the pulmonary vascular endothelium and a reduction in guanylate cyclase activity. Rebound PHT responds to reinstitution of iNO; however, an interesting pharmacological solution is the addition of sildenafil, a selective inhibitor of phosphodiesterase type 5, which prevents the breakdown of c‐GMP until c‐GMP levels become naturally replete. As such, a single i.v. dose of 0.4 mg/kg sildenafil before weaning from iNO prevents rebound PHT in neonates and infants [95]. iNO has few side effects. However, the binding of NO to hemoglobin gives rise to methemoglobin; therefore, methemoglobin levels should be routinely monitored particularly in patients receiving prolonged therapy. Nitrogen dioxide is a byproduct of NO administration and can injure the lung parenchyma; therefore, its concentrations should be maintained below 5 ppm. The initial dose of iNO is usually set at 20 ppm, and reasonable attempts to wean the patient off it should be made every 12–24 hours. iNO has had an undisputed impact on the management of PHT, and it continues to warrant consideration as adjunctive therapy for some of the more severe patients. However, a recent meta‐analysis concluded that iNO does not reduce short‐term mortality [96]. It also concluded that there was insufficient information to determine whether it has an impact on neurodevelopmental outcome, long‐term mortality and length of hospital stay [96]. iNO is not specifically approved by the US Food and Drug Administration (FDA) for the treatment of post‐surgical PHT, although it is approved for the treatment of persistent PHT in term neonates. Arginine and citrulline are substrates for NO synthase. In addition, arginine, an essential amino acid due to the inability to increase its de novo synthesis, plays a central role in cell proliferation, protein synthesis, energy metabolism, and ammonia detoxification, and the question of its relationship with postoperative inflammation and organ dysfunction has been raised. Arginine depletion has been reported after CPB, together with increased expression of arginase, and a fall in both citrulline and glutamine; the mechanism remains uncertain, however, plasma arginine concentrations recovered after day 5 and initiation of enteral feeding [97]. Although the link with NO synthesis has been made, research in the domain of oral arginine or citrulline supplementation has remained inconclusive, with a consequent increase in the plasma concentrations but no impact on the occurrence of PHT postoperatively [98]. Sildenafil is a selective inhibitor of phosphodiesterase type 5, which increases c‐GMP levels in the pulmonary vascular endothelium, as shown in Figure 21.8. When administered orally to healthy subjects, the bioavailability of sildenafil is only approximately 40%. Also, critically ill children may show unpredictable enteral absorption, therefore, i.v. sildenafil may be more appropriate in such cases. Sildenafil is metabolized in the liver, and it has a half‐life of about 4 hours. Oral pediatric doses are between 1 and 5 mg/kg, but the greater bioavailability of the i.v. preparations allows effective doses of 0.5 mg/kg. Sildenafil is at least as effective as iNO for reducing increased PVR in children with CHD [99], and it enhances the pulmonary vasodilator effects of iNO [100]. The use of sildenafil facilitates the successful weaning of patients off iNO [95]. Nevertheless, unlike iNO, because sildenafil is relatively nonselective, systemic administration is associated with a significant increase in intrapulmonary shunting and a clinically nonsignificant fall in arterial blood pressure [99, 100], which may sometimes outweigh its benefits. Intrapulmonary shunting is caused by vasodilatation of the pulmonary arterioles supplying nonventilated areas of the lung, and results in hypoxia. Although currently approved for use in adults with PHT, sildenafil is extensively used off‐label to treat neonates, infants, and children with PHT since 2005. A recent randomized controlled trial of sildenafil for the long‐term therapy of children with PHT (STARTS‐1) [101], including idiopathic PHT, and PHT associated with connective tissue disease and CHD, demonstrated a decrease in pulmonary artery pressure and PVR with medium and high‐dose sildenafil, and no significant change with low‐dose sildenafil. However, in the long‐term extension study (STARTS‐2) [102], there was increased mortality noted at 3 years associated with high‐dose sildenafil in children with idiopathic PHT, but not in those with CHD‐related PHT. Review of these data by the FDA and European Medicines Agency resulted in contradictory recommendations; sildenafil was approved in Europe in 2011 with a later warning on avoidance of the use of high‐dose sildenafil, and the FDA released warnings against the use of sildenafil in pediatric patients aged 1–17 years. In view of the limitations of the START‐2 extension study and in view of the Pediatric Pulmonary Hypertension Network consensus, the FDA warning was later revised to add that health care providers should consider the risk and benefits of sildenafil for the individual patient. Tadalafil and vardenafil are two other phosphodiesterase type 5 inhibitors that are currently under investigation. Prostaglandins (PGs) are the main metabolites of the arachidonic acid pathway. They are generated and released by the endothelium, bind to receptors on the underlying arterial smooth muscle, thereby activating adenyl cyclase, increasing c‐AMP levels, and inducing smooth muscle relaxation as shown in Figure 21.8. Although PGs are rapidly metabolized by the pulmonary circulation, doses large enough to induce pulmonary vasodilation also induce systemic vasodilation. Inhaled PGs show more selective vasodilator properties than i.v. preparations and, like iNO, they prevent a ventilation/perfusion mismatch by redistributing blood flow to the ventilated aerosol‐accessible regions. PGE1 was the first PG to be isolated. It is used extensively to maintain the patency of the ductus arteriosus in neonates whose systemic or pulmonary circulation is dependent on ductal patency. In addition, i.v. PGE1 has been used to treat PHT. Side effects occur in 20–40% of patients treated with higher doses (0.05–0.1 μg/kg/min), but they are reversible upon reverting to a low dose or discontinuing the drug. Nevertheless, the most common side effects, that is, hypotension, apnea, and hyperpyrexia, may complicate the postoperative course and outweigh any potential benefits. Prostacyclin (PGI2) was first identified in 1976, and early studies of the use of i.v. PGI2 in children suggest that it induces highly selective pulmonary vasodilation within the pulmonary vascular bed, where it is inactivated during a single circulation time. However, the efficacy and pulmonary selectivity of the i.v. PGI2 formulation, epoprostenol, following CHD surgery is similar to that of i.v. PGE1 [103]. The inhaled formulation, iloprost, also shows good pulmonary selectivity. It also has a longer half‐life (25 min), although repeated inhalation is needed for optimum clinical effectiveness. Iloprost may be used as routine therapy of PHT in countries where iNO is not available because of its prohibitive cost [104], since it has been shown to have similar efficacy to iNO in children with CHD [105]. Finally, iloprost may be useful in children who do not respond to iNO therapy or as a rescue therapy when other treatments have failed [104]. Other PGI2 analogues, such as treprostinil (inhaled), or beraprost (oral) may have potential utility for the treatment of postoperative PHT in children. The actions of corticosteroids on the cardiovascular system are mediated via both genomic and nongenomic effects: they exert anti‐inflammatory effects by modulating the transcription of RNA and the subsequent synthesis of proinflammatory proteins, and they represent an adjunct therapy that reduces the need for high doses of inotropes by decreasing the reuptake of norepinephrine. High‐dose corticosteroids have strong anti‐inflammatory effects and are largely used in the perioperative period [91]. Corticosteroid treatment is associated with lower hyperthermia‐related increases in VO2, reduced fluid requirement, a shorter length of hospital stay [20], and improves myocardial protection [106]. Altogether, modulation of CPB‐mediated inflammation by corticosteroids seems clinically beneficial in pediatric patients, especially when targeted to younger and more complex patients. Although the dosing regimen used in children was empirically set at 30 mg/kg prior to CPB, followed by 30 mg/kg at the start of CPB, a recent survey of 36 cardiac surgery centers showed that administration and dosing regimens were mainly based on provider preferences [107]. On the other hand, corticosteroids inhibit norepinephrine uptake by non‐neuronal cells, thereby increasing norepinephrine concentration at α‐adrenergic receptor sites; additionally, by inhibiting the catechol‐O‐methyltransferase enzyme, they lead to increases in the plasma norepinephrine concentrations. Corticosteroids also increase cytosolic calcium availability in myocardial and vascular smooth muscle cells and improve capillary integrity in patients with capillary leak syndrome. Finally, they inhibit PGI2 production and the induction of NO synthase, thereby limiting the pathologic vasodilation associated with the inflammatory response. Corticosteroids can be considered for children with LCOS; however, a formal definition of adequate steroid dosing is required. Interestingly, the use of low doses (100 mg/m2/day of a hydrocortisone equivalent) may be sufficient for use as a rescue protocol in children with LCOS [108]. A recent randomized trial in neonates demonstrated that a prophylactic 50 mg/m2 bolus at the end of CPB, followed by a 50 mg/m2/day continuous infusion reduced the incidence of LCOS [109]. Therefore, adrenal insufficiency has often been invoked in patients with postoperative LCOS following CPB, which might be similar to what is seen in patients with septic shock, and which can be reversed by the administration of a “substitutive” dosage range. Recent work reported a significant decline in the cortisol response to adrenocorticotropic hormone stimulation throughout the first 24 hours following CPB in infants, associated with increased fluid resuscitation and longer length of stay [110]. Another hypothesis is the suppression of the hypothalamic–pituitary–adrenal axis due to exposure to high‐dose perioperative corticosteroids [111], which brings additional arguments against the extensive use of high dosages. Controversy regarding the perioperative use of corticosteroids is ongoing, and the most recent randomized trials of corticosteroids in neonates with CPB reported no improvement in postoperative outcomes with, however, an improvement in fluid balance and shortening of time to extubation [112–114]. Similar conclusions were drawn from the most recent meta‐analysis of the prophylactic use of corticosteroids in CHD surgery [115]. By contrast, a very large study based on data obtained from the Pediatric Heath Information Systems Database, including 46,730 children from 38 US centers from 2003 to 2008, was able to show that the use of corticosteroids was associated with a greater risk of infection, a greater postoperative requirement for insulin, and, finally, longer length of hospital stay (96). The association with morbidity was more prominent in the low‐risk group. Similar conclusions were drawn from a subset study restricted to neonates [116]. Thyroid hormone levels are depressed after CPB. Tri‐iodothyroxine (T3) supplementation has been used in an attempt to increase cardiac index postoperatively with encouraging initial results (99,100): increased cardiac index, decreased inotropic requirement, better fluid balance and shorter time to extubation. Recent meta‐analysis of T3 trials evaluated the impact on clinical outcomes in children and infants with CHD surgery [117, 118]. They reported a very wide variability of dosages, ranging from 0.4 to 5 μg/kg over a 24‐hour period, and a wide variation in the duration of therapy, ranging from a single dose at the end of CPB to continued hormone therapy for the duration of intensive care unit (ICU) stay. The inotrope scores were significantly lower in the T3 group; however, there was no difference in cardiac index, the duration of mechanical ventilation, ICU stay or mortality. No adverse events or delay in the postoperative recovery of thyroid function were reported either. β‐adrenergic receptor blockers are beneficial for the management of chronic heart failure in both children and adults because they improve functional status. Patients with chronic heart failure show a downregulation of β‐adrenergic receptors due to increased sympathetic tone; β‐blockers, such as propranolol, metoprolol, and carvedilol increase the number of myocardial β‐adrenergic receptors and improve myocardial function. In the acute setting of CHD surgeries, β‐blockers reduce the effects of increased sympathetic tone on the RV infundibulum and heart rate in patients with tetralogy of Fallot, thereby improving RV filling. Esmolol, a short‐acting β1‐receptor selective antagonist, is well suited for the perioperatively treatment of tetralogy of Fallot patients [119]. Esmolol (100–700 μg/kg/min) controls postoperative hypertension following coarctation repair [120] and avoids reflex tachycardia that results from the use of selective vasodilators. Administration of calcium in the form of calcium chloride or calcium gluconate helps improve the inotropic function of the heart in patients with hypocalcemia. This is especially important in neonates, in whom plasma membrane Na–Ca exchange and calcium influx channels play a greater role than the sarcoplasmic reticulum in regulating intracellular calcium concentrations; therefore, neonates are highly dependent on extracellular calcium. Neonates receive significant amounts of citrated blood products; however, citrate binds calcium and causes hypocalcemia. Calcium is also a major role in excitation–contraction coupling in smooth muscle, and functions as a vasoconstrictor. However, routine administration of calcium salts upon termination of CPB is a subject of debate. Elevated intracellular calcium levels are associated with injury and cell death during ischemia and reperfusion [121]. This is further aggravated in the neonatal myocardium, where the sarcoplasmic reticulum is underdeveloped and does not insure calcium uptake in the case of an elevation in intracellular calcium levels. Buffering acidosis is essential in patients with, or at risk of, high PVR. The deleterious effect of acidosis on PVR, particularly in hypoxemic conditions, was demonstrated experimentally more than 50 years ago [122], and the patterns of PVR variations as a response to changes in pH are shown in Figure 21.9. More recently, it has been shown that the administration of sodium bicarbonate to increase pH in infants with increased PVR reduces PVR by approximately 50% and increases cardiac index by approximately 35%, even if hypercapnia is present [123]. This agrees with a previous study showing that pH plays a major role in regulating PVR, and that metabolic alkalosis is as effective as respiratory alkalosis for treating hypoxia‐induced pulmonary vasoconstriction [124]. Lactic acid levels increase due to cellular hypoxia, and lactic acidosis is an adaptive mechanism that enables an organism to survive hypoxic conditions. Lactic acidosis moves the dissociation curve for hemoglobin to the right, thereby allowing improved oxygen transfer to the tissues. Therefore, while extreme values of acidemia (pH < 7.2) interfere with myocardial performance and responses to catecholamines, and should be buffered, a pH lower than normal is essential for adaptation. Bicarbonate buffering has several side effects, including paradoxical intracellular acidosis and further impairment of cardiac function [125], a risk of hypernatremia, the creation of a hyperosmolar state, and a risk of intraventricular hemorrhage in neonates. Figure 21.9 Patterns of pulmonary vascular responses to changes in pH and PO2. (Source: Rudolph & Yuan [122]. Reproduced with permission of The American Society for Clinical Investigation.) Isoproterenol, a nonselective β‐adrenergic receptor agonist, is a synthetic derivative of norepinephrine. Isoproterenol has more chronotropic than inotropic effects and lowers the SVR. It is used to treat hemodynamically significant bradycardia and during cardiac anesthesia in the denervated heart immediately after transplantation. High doses of isoproterenol can be arrhythmogenic, and it is often used in electrophysiologic studies. Isoproterenol induces an increase in myocardial VO2, whereas DO2 decreases as a result of reduced coronary diastolic filling; this may exacerbate or induce ischemia. Therefore, patients receiving isoproterenol should be volume replete. Fenoldopam is a selective D1‐receptor agonist that shows potent vasodilatory effects, particularly in the renal, mesenteric, coronary, and skeletal muscle vascular beds. Fenoldopam maintains adequate renal perfusion while reducing blood pressure at the same time. Few data are available regarding its use following CHD surgery. Small, albeit randomized, studies show that it is safe in neonates and has a beneficial effect on kidney function following CPB when high doses are used (1 μg/kg/min) [126, 127]. Two recent surveys describe the current international practice variation: the EuLoCOS‐Ped European survey [27, 32], completed in 2009, collecting information from 90 centers from 31 European countries, and the survey of the Pediatric Cardiac Intensive Care Society (PCICS) [26], completed in 2015, reporting practices in 62 centers, 69% of whom were North American. Medical treatment of LCOS is constrained by a lack of appropriate drug and dosing guidance as acknowledged by the respondents of the European survey [27, 32]. Overall, the EuLoCOS survey alone reports 24 different drug regimens to prevent LCOS, and 39 drug regimens to treat LCOS [27, 32]. Besides, as mentioned before, 78% of all patients receive at least one cardiovascular drug off‐label, with surgical CHD patients receiving a median of three drugs off‐label [28]. Dobutamine alone is licensed for use as inotropic support in children with LCOS in Spain and Germany [128], although, efficacy data are lacking in neonates. Preventive LCOS therapy is a common practice, reported in 77.8% of all pediatric cases in the EuLoCOS survey. However, there is a large variability in the choice of drugs being used, as shown in Table 21.4. The distribution of the timing of administration is different as well. In 64% of all cases, milrinone in prophylactically administered in European centers to prevent LCOS, however, the dosage and duration of administration differ substantially among hospitals: the bolus dose varies from 20 to 300 μg/kg, the maintenance infusion ranges from 0.2 to 1 μg/kg/min, and the duration varies between 6 and 168 hours. The dose of milrinone appears to be less than that recommended by PRIMACORP [4], which may be due to concerns about the potential side effects, particularly hypotension and age‐specific pharmacokinetic differences in drug elimination [51]. In 32% of cases, milrinone is associated with a catecholamine in the EuLoCOS survey: dopamine, dobutamine, epinephrine, and levosimendan are used in about 16% of cases each, with a large variety of dosages. Of the PCICS respondents, 97% use milrinone to prevent LCOS, most of the time in association with a catecholamine: epinephrine in 43%, dopamine in 36%, dobutamine in 11% and levosimendan in 11% of cases. The high percentage of centers using catecholamines as a preventive therapy, 32% in EuLoCOS and 94% in the PCICS survey, is surprising in view of their serious side effects mentioned before. Table 21.4 Current practices for the management of low cardiac output syndrome after congenital heart surgery LCOS: low cardiac output syndrome, PVR: pulmonary vascular resistance, SVR: systemic vascular resistance Results from the EuLoCOS survey 2009 (left) [27, 32] and from the Pediatric Cardiac Intensive Care Society (PCICS) survey 2015 (right) [26]. The therapeutic strategies to treat LCOS, such as reported by the EuLoCOS and PCICS surveys, are shown in Table 21.4. In keeping with the European survey [32], the six drugs that constitute 90% of total drug use to treat LCOS in children are milrinone, dopamine, dobutamine, epinephrine, levosimendan, and methylprednisolone. Milrinone monotherapy was the preferred drug regimen for the initial treatment of LCOS with elevated SVR, epinephrine was the preferred first add‐on drug and levosimendan was added as the next step. Milrinone monotherapy was the treatment of choice for the initial treatment of LCOS with elevated PVR, the first and second add‐on drugs were iNO and PGI2 derivatives. The PCICS survey revealed milrinone being used as a first choice in only 42% of cases with LCOS, whereas catecholamines (epinephrine and dopamine) were used in 51% of cases. This, again, is surprising in the context of postcardiac surgery LCOS, where the major therapeutic objectives are the decrease of LV and RV afterload, and avoidance of myocardial oxygen debt. Inotropic and vasoactive scores are derived from summing the maximum dose rates, using correction factors to account for differential units of measurement of inotropes and vasopressor medications administered in the first 24 hours after cardiac surgery. The inotropic score (IS) was first proposed in the Boston Circulatory Arrest Study [3] to quantify the pharmacologic cardiovascular support given postoperatively. Later on, it has often been used as a measure of illness severity following cardiac surgery in children even though the score was not created for this purpose. Gaies et al. [129] proposed expanding the IS to include other commonly used vasoactive medications, such as milrinone, vasopressin, and norepinephrine, and called it the vasoactive‐inotrope score (VIS). The scores are calculated as:
CHAPTER 21
Hemodynamic Management
Introduction: Hemodynamic management following congenital heart disease surgery: a goal‐directed approach
The VO2/DO2 balance following CHD surgery
Mechanisms underlying increases in VO2 following CHD surgery
Mechanisms underlying reduced DO2 following CHD surgery
Improving postoperative oxygen transport balance: preventive and therapeutic interventions
Peripheral vascular effect
Inotropic effect
Yes
No
Vasoconstriction
Inoconstrictors
Vasoconstrictors
Epinephrine
Norepinephrine
Dopamine
Vasopressin
Phenylephrine
Vasodilation
Inodilators
Vasodilators
Milrinone
Nitroglycerin
Dobutamine
Nitroprusside
Levosimendan
Phenoxybenzamine
Inotropic agents
Receptor
Receptor location site
Action
α1
Vascular smooth muscle
Arterial vasoconstriction
Heart
↑ inotropic activity
α 2
Vascular smooth muscle
Vasoconstriction of venous capacitance vessels
Presynaptic sympathetic nerve terminals
Local feedback, inhibition of norepinephrine release
β1
Heart
↑ inotropic and chronotropic activity
↑ atrio‐ventricular node conduction velocity
β2
Vascular smooth muscle
Vasodilation peripheral vasculature
Bronchial smooth muscle
Bronchodilation
D1
Vascular smooth muscle (renal, splanchnic, cerebral)
Vasodilation
Renal tubules
↓ Na reabsorption
D2
Presynaptic sympathetic nerve terminals
↓ norepinephrine release
β‐adrenergic receptor agonists
Drug
Receptors
Inotropy
Chronotropy
SVR
PVR
Renal vascular resistance
Cardiac output
Epinephrine < 0.1 μg/kg/min
>0.1 μg/kg/min
β1, β2 > α1 (agonist)
α1 > β1, β2
↑
↑
↑
↑
↔ ↓
↑
↔ ↓
↑
↓
↑
↑↑
↑
Dopamine < 3 μg/kg/min
4–8 μg/kg/min
>8 μg/kg/min
D1, D2 (agonist)
β1, β2 > α1
α1 > β1, β2
↔
↑
↑
↔
↑
↑
↔
↔ ↓
↑
↔
↔
↑
↓
↔
↑
↔
↑
↔
Dobutamine
β1 > β2, α1 (agonist)
↑
↑
↓
↓
↔
↑↑
Milrinone
PDE3 inhibitor
↑
↑
↓
↓
↓
↑
Levosimendan
Troponin C, ATP‐dependent K channel
↑
↑
↓
↓
↓
↑
Norepinephrine
α1 > β1, β2 (agonist)
↔ ↑
↓
↑↑
↑
↑↑
↔ ↓
Vasopressin
V1a (agonist)
↔
↓
↑
↑
↑
↔ ↓
Phenylephrine
α1 (agonist)
↔
↓
↑
↔
↑
↔ ↓
Nitroglycerine
Nitroprusside
Guanylate cyclase activator
↔
↔ ↑
↓
↓
↓
↑
Phenoxybenzamine
α1, α2 (antagonist)
↔
↔ ↑
↓
↓
↓
↑
Inhaled Nitric oxide
Guanylate cyclase activator
↔
↔
↔
↓↓
↔
↔ ↑
Sildenafil
PDE5 inhibitor
↔
↔
↔
↓↓
↔
↔ ↑
Epinephrine
Dopamine
Dobutamine
Norepinephrine
Side effects of catecholamines
Inhibitors of phosphodiesterase type 3
Amrinone
Milrinone
Calcium sensitizers
Vasoconstrictors
Norepinephrine
Arginine vasopressin
Phenylephrine
Systemic vasodilators
Nitric oxide donors: nitroglycerin and sodium nitroprusside
Phenoxybenzamine
Phentolamine
Hydralazine
Nesiritide
Pulmonary vasodilators
Inhaled nitric oxide
Sildenafil
Prostaglandins
Other strategies to improve circulatory function
Corticosteroids
Thyroid hormone
Beta‐adrenergic receptor antagonists
Calcium
Sodium bicarbonate
Isoproterenol
Fenoldopam
Current practices
EuLoCOS survey
PCICS survey
LCOS prophylactic drug regimen
%
LCOS prophylactic drug regimen
%
Milrinone
Milrinone + dopamine
Milrinone + epinephrine
Milrinone + dobutamine
Milrinone + levosimendan
Levosimendan
25
12
11
9
7
7
Milrinone
Epinephrine
Dopamine
Dobutamine
Levosimendan
97
45
38
11
5
Timing of administration
%
Timing of administration
%
Intra‐ and postoperatively
Pre‐, intra‐, and postoperatively
Intraoperatively
Postoperatively
Preoperatively
Pre‐ and intraoperatively
57
21
8
8
4
1
Preoperatively
After anesthetic induction
When on CPB
While coming off CPB
In the Intensive Care Unit
1
1
42
63
29
Treatment of LCOS
with elevated SVR
%
Treatment of LCOS
with elevated PVR
%
Treatment of LCOS
%
Initial treatment
First add‐on
Second add‐on
34
8
8
24
11
9
22
22
12
Initial treatment
First add‐on
Second add‐on
17
13
10
20
11
11
22
14
14
First choice
Second choice
42
36
15
2
39
15
10
9
Inotropic and vasoactive scores
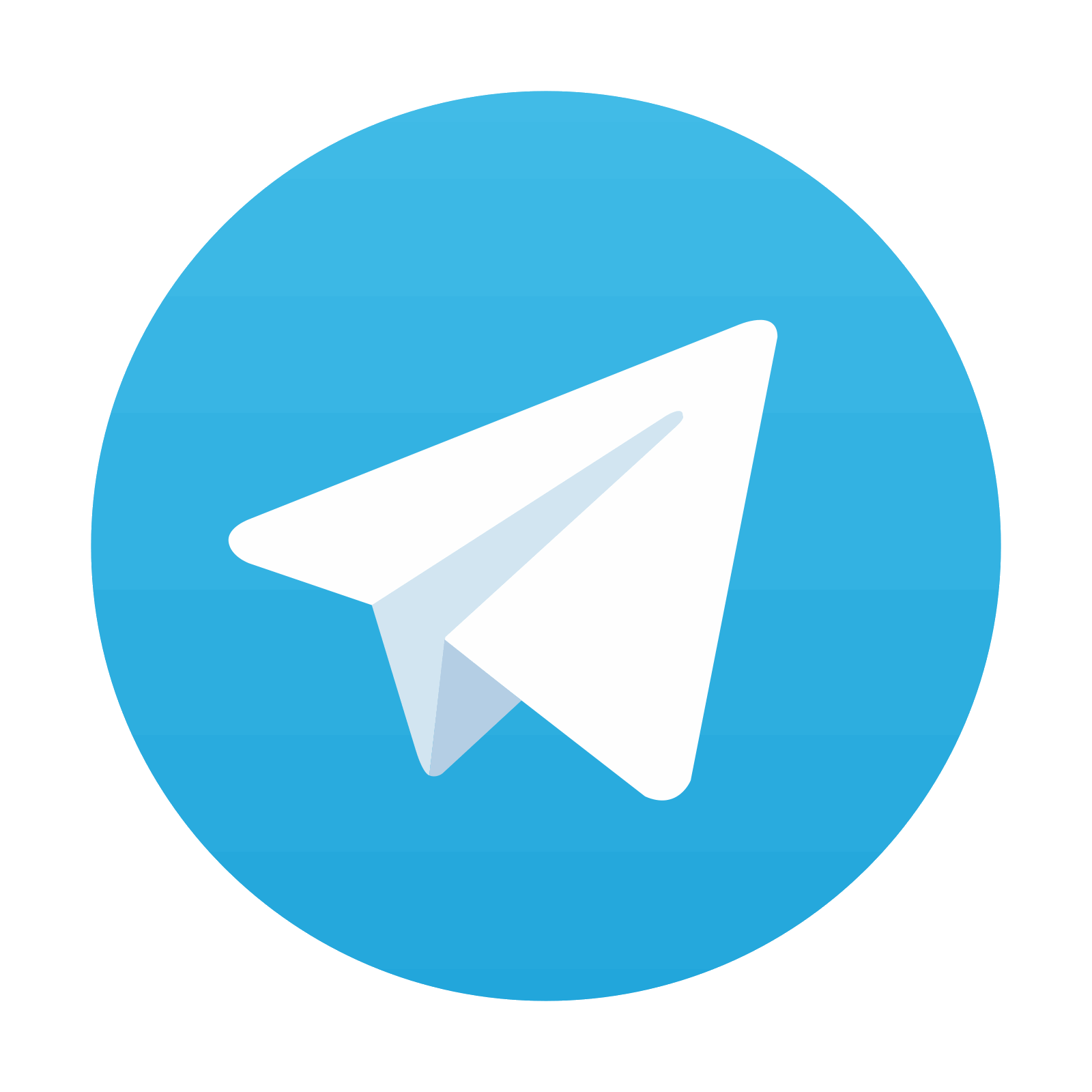
Stay updated, free articles. Join our Telegram channel
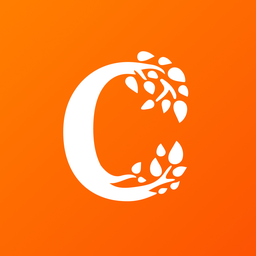
Full access? Get Clinical Tree
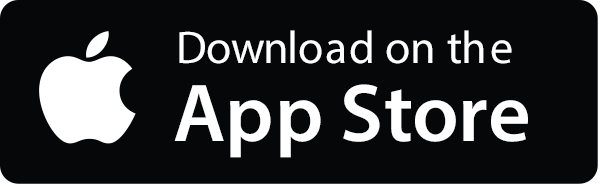
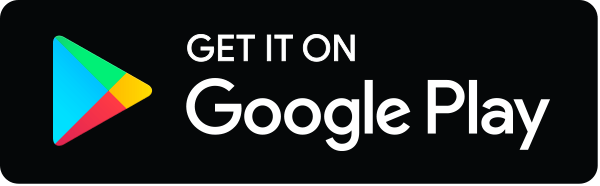