Laura Diaz‐Berenstain1, Rania K. Abbasi2, Lori Q. Riegger3, James M. Steven4, Susan C. Nicolson5, and Dean B. Andropoulos6 1 Division of Pediatric Cardiac Anesthesiology, Cincinnati Children’s Hospital Medical Center, Cincinnati, OH, USA 2 Division of Pediatric Cardiac Anesthesia, Riley Hospital for Children/Indiana University School of Medicine, Indianapolis, IN, USA 3 Congenital Cardiac Anesthesiology, Service Chief, Pediatric Anesthesia, Department of Anesthesiology, Section of Pediatric Anesthesiology, University of Michigan Medical School, Ann Arbor, MI, USA 4 Division of Cardiac Anesthesia, Medical Advisor to the Risk Management Department, Associate Professor of Anesthesia, Perelman School of Medicine at the University of Pennsylvania, Philadelphia, PA, USA 5 Division of Cardiac Anesthesia, Josephine J. Templeton Endowed Chair in Pediatric Anesthesiology Clinical Education, Medical Director, Cardiac Center Operations, Professor of Anesthesiology, Perelman School of Medicine at the University of Pennsylvania, Philadelphia, PA, USA 6 Department of Anesthesiology, Perioperative and Pain Medicine, Department of Anesthesiology, Baylor College of Medicine, Texas Children’s Hospital, Houston, TX, USA In the early 1970s, Fontan and Baudet [1] and Kreutzer et al. [2] independently described the operative treatment of tricuspid atresia (TA) that resulted in nearly normal systemic arterial oxygen saturation and normal volume work for the single functional systemic ventricle. This procedure subsequently referred to as the Fontan operation, created a series circulation that requires the single ventricle (SV) to pump fully saturated blood only to the systemic circulation, thereby reducing the pressure and volume work to that of a normal systemic ventricle. Systemic venous drainage passes directly through the pulmonary vascular bed without the benefit of a pumping chamber, and pulmonary vascular resistance (PVR) must be low to maintain the pulmonary circulation and the cardiac output (CO) on which it depends. The principle of the Fontan operation has subsequently been applied to the full spectrum of cardiac lesions with one functional ventricle. Suitable physiology for ultimate palliation with a modification of the Fontan procedure is predicated on appropriately timed and executed staged operations designed for the specific patient’s SV physiology. Essentially, all patients with a single functional ventricle now undergo staged palliation, resulting in the completion of the Fontan circuit at 18 months to 4 years of age. The majority undergoes a neonatal palliative procedure, followed by a superior cavopulmonary connection (bidirectional Glenn or hemi‐Fontan procedure) at 3–6 months of age in all patients. This chapter will initially present these principles by using hypoplastic left heart syndrome (HLHS) as the most common SV congenital cardiac lesion. Tricuspid atresia and other forms of SV lesions will then be discussed. Finally, the growing recognition of the implications and sequelae of the long‐term Fontan circulation, along with management strategies, will be described. Figure 30.1 Hypoplastic left heart syndrome. This diagram depicts mitral stenosis and aortic atresia. ASD, atrial septal defect; RA, right atrium; RV, right ventricle; LA, left atrium; LV, left ventricle; PA, pulmonary artery. (Source: Metcalf & Rychik [6]. Reproduced with permission of Elsevier.) Hypoplastic left heart syndrome (HLHS) represents the fourth most common defect presenting in the neonatal period and accounts for 7.5% of newborns with congenital heart disease (CHD) requiring early therapeutic intervention. Although the incidence of HLHS is 1.4–3.8% of all CHD, it results in 23% of deaths from CHD within the first week of life and 15% within the first month [3]. HLHS has a high heritability component, inherited as a complex trait causing a severe form of valve malformation. Thus, it is important to screen first‐degree relatives of HLHS patients [4]. Recent genome sequencing has demonstrated a link between gene defects causing HLHS and those causing cardiomyopathies. Clinically, certain variants associated with these genes could increase the risk of developing myocardial dysfunction in HLHS patients [5]. HLHS is characterized by a single right ventricle (RV) and varying degrees of hypoplasia of the left side of the heart. Most commonly HLHS involves either severe aortic stenosis (AS) or aortic atresia (AA), severe mitral stenosis (MS) or mitral atresia (MA), and a very hypoplastic or rudimentary left ventricle (LV) (Figure 30.1) [6, 7]. Blood returns normally from the pulmonary veins to the left atrium (LA), but obstruction at the mitral and aortic levels means that the majority of flow must be left‐to‐right across the atrial septum. This causes enlargement and dilation of the right atrium (RA) and RV, which must support the outputs of both the systemic and pulmonary circulations. Blood flows from the pulmonary artery (PA) right‐to‐left through the patent ductus arteriosus (PDA) to the aorta, thus supplying the systemic arterial circulation. Although there may be some antegrade flow through the aortic valve, perfusion of the brachiocephalic vessels, aortic root, and coronary arteries occurs mainly via retrograde flow from the PDA into the ascending aortic arch – a hallmark of HLHS. Some variations of left heart hypoplasia involving the LV, mitral valve (MV), and aortic valve (AV) may be amenable to two‐ventricle surgical repairs and are presented in detail in Chapter 27. Without intervention, HLHS is uniformly fatal in the first several months of life. As the PDA narrows and closes, diminished blood flow to the systemic circulation and coronary arteries results in a state of circulatory shock resulting in poor perfusion, lactic acidosis, and respiratory failure. A parallel circulation exists in HLHS. Both pulmonary and systemic venous blood mix in the RA, and the RV, via the PA, then supplies both the pulmonary circulation (through the branch PAs) and the systemic circulation (through the PDA). Prostaglandin E1 infusion must be initiated after birth to maintain patency of the ductus arteriosus. Maintenance of a delicate balance between pulmonary (Qp) and systemic (Qs) blood flow is necessary, with the Qp: Qs optimally kept near 1: 1 to maintain adequate perfusion of lungs and body. As pulmonary vascular resistance (PVR) decreases after birth, Qp: Qs increases and may reach 2: 1 or 3: 1. The resultant pulmonary overcirculation at the expense of the systemic circulation generally results in pulmonary interstitial and alveolar edema, tachypnea, and retractions, potentially culminating in respiratory failure. Increases in FiO2 or hyperventilation will further lower PVR and should be avoided. Even more critical is the “steal” effect, in which blood flow is diverted away from the systemic circulation, compromising flow to both the body and the coronary arteries. Cardiogenic shock can ensue, with poor perfusion and increasing lactic acidosis. Steal of flow from the systemic circulation may also result in mesenteric ischemia and necrotizing enterocolitis. Maneuvers to increase PVR are important treatment strategies if steal is suspected. These strategies can include maintaining FiO2 at 0.21 and allowing PaCO2 to rise via spontaneous respiration or by decreasing minute ventilation for mechanically ventilated patients. The former practice of delivering sub‐ambient FiO2 via mechanical ventilation can reduce both systemic and cerebral oxygenation significantly but has been abandoned in favor of maintaining spontaneous respirations preoperatively and early surgical intervention [8]. Should the PDA narrow, Qp: Qs increases and systemic perfusion becomes compromised, leading to a cardiogenic shock. Figure 30.2 Pathophysiology of the functional single systemic ventricle. Optimal oxygen delivery involves maintaining adequate cardiac output while balancing SVR and PVR. SVR, systemic vascular resistance; PVR, pulmonary vascular resistance; PBF, pulmonary blood flow; AV, atrioventricular; HR, heart rate; PCO2, partial pressure of carbon dioxide; PO2, oxygen tension. Anatomic variables can have a major impact on the observed physiology. If the ASD is restrictive or limited to a patent foramen ovale (PFO), resistance to pulmonary venous return may balance pulmonary and systemic resistances, positively impacting Qp: Qs. Infants who lack that restriction, such as those with a large atrial septal defect (ASD) or unrestrictive anomalous pulmonary venous return, tend to exhibit high Qp: Qs. However, infants presenting with an intact atrial septum and no alternative decompressing vein have severely obstructed pulmonary venous return, leading to left atrial hypertension and an extremely low Qp: Qs. These infants exhibit marked hypoxemia and require intervention to enlarge the atrial communication and decompress pulmonary venous return in order to have any chance of survival. This may be accomplished either via in utero atrial septostomy or immediately after planned delivery in an adjacent operating room (OR) via an ex utero intrapartum treatment (EXIT) procedure [9, 10]. Several other factors, including atrioventricular valve regurgitation (AVVR) and hemoglobin level, can also affect the pathophysiology of HLHS. An overview of SV pathophysiology is presented in Figure 30.2. In the current era, the majority of HLHS cases are diagnosed in utero after an abnormal screening ultrasound at 18–22 weeks’ gestation is confirmed by a detailed cardiac examination. Fetal echocardiography can also reveal additional features including limitation of flow across the atrial septum and abnormal pulmonary venous return flow patterns which, if present, can greatly impact risk and survival [10]. In the Single Ventricle Reconstruction Trial (SVRT), a multicenter study of over 500 HLHS patients sponsored in the US and Canada by the Pediatric Heart Network of the US National Heart, Lung, and Blood Institute, 76% of HLHS patients were diagnosed in utero [11]. As noted, fetal catheter interventions may be attempted for some HLHS patients, either to dilate and stent a restrictive atrial septum or to dilate the aortic valve. An analysis from the International Fetal Cardiac Intervention Registry that examined fetuses with HLHS from 13 institutions between 2001 and 2015 demonstrated a 77% success rate with atrial septoplasty and atrial stent placement procedures. However, complications, including 13% fetal demise, were common [9]. Fetal interventions are discussed in detail in Chapter 19. Although prenatal diagnosis alone has not been demonstrated to improve HLHS survival, late postnatal diagnosis and distance from a cardiac surgical center negatively impact survival, with most mortality occurring before surgical intervention [12]. Therefore, prenatal diagnosis and planned delivery in or near a cardiac surgery center may improve survival. Late diagnosis occurs when the PDA narrows and the infant demonstrates clinical signs of low CO and shock, prompting echocardiographic assessment. Emergent resuscitation of the infant, including immediate initiation of a PGE1 infusion, is essential for survival. The magnitude and the type of acquired vital organ dysfunction usually correlate with the degree of circulatory instability at the time of diagnosis. Infants who have suffered a profound or protracted shock state can demonstrate a wide spectrum of injury to renal, central nervous system, cardiac, gastrointestinal, or hepatic systems [13–15]. These derangements may necessitate a delay in operative intervention to permit recovery. Because 15–30% of HLHS neonates also have extracardiac anomalies or genetic syndromes such as the CHARGE association or chromosomal abnormalities, a thorough evaluation for these problems should also be performed [3]. In 1979, Norwood et al. reported a neonatal palliative operation for HLHS that stabilized native HLHS physiology and eliminated ductal dependence. He later described a Fontan palliation as the second stage operation, resulting in the first long‐term survivors of HLHS [16]. The Norwood stage I palliation involves reconstruction of the diminutive ascending aorta, usually with a cryopreserved homograft patch, along with the creation of outflow from the systemic RV through the pulmonary valve, which also provides blood flow to the native aortic root and coronary arteries. The pulmonary valve becomes the “neo‐aortic valve” (Figure 30.3) [17, 18]. The main PA is transected, and the proximal portion of the PA is incorporated into the aortic repair while the underside of the PA bifurcation is oversewn. In addition, an atrial septectomy is performed to provide unrestricted left‐to‐right blood flow across the atrial septum. Pulmonary blood flow is then delivered via a polytetrafluoroethylene (PTFE) shunt, either via the creation of a systemic‐to‐pulmonary shunt from the right innominate or subclavian artery to right PA (also termed modified Blalock–Thomas‐Taussig shunt [mBTTS]) or by the creation of an RV–PA shunt (also termed the Sano shunt). It is crucial that the area of the anastomosis between the pulmonary valve and the aortic valve is free of obstruction, as it can affect the already tenuous coronary blood flow. The complete removal of any residual ductal tissue to prevent later coarctation of the aorta is also critical. During aortic arch reconstruction, cardiopulmonary bypass (CPB) techniques must provide a bloodless surgical field. Deep hypothermic circulatory arrest (DHCA) or antegrade regional cerebral perfusion (ACP) may be utilized for this purpose. Preference for a particular approach is largely surgeon and institution dependent. Details of each technique along with short‐ and long‐term outcomes are discussed in Chapter 11. Figure 30.3 Norwood Stage I palliation for hypoplastic left heart syndrome. See text for details. (Source: Berenstain & Spaeth [18]. Reproduced with permission of Cambridge University Press.) The systemic‐to‐pulmonary artery shunt is a 3.0‐ to 4.0 mm polytetrafluoroethylene (PTFE) graft that provides continuous flow into the PA. Originally described as a modified “Blalock‐Taussig” shunt after surgeon Alfred Blalock and cardiologist Helen Taussig, more recently, it has become identified as a modified “Blalock‐Thomas‐Taussig shunt” to recognize the primary contribution of Vivien Thomas, a surgical technologist, to its successful development and implementation [19, 20]. Shunt flow is inversely proportional to the length of the shunt and the fourth power of its radius. It is directly proportional to the pressure drop across the shunt and therefore influenced by the ratio of PVR and systemic vascular resistance (SVR). Kinking or distortion of either the shunt or PA can occur and interfere with the flow. Because there is continuous “run‐off” of flow from the systemic circulation into the PA, these patients remain at risk for steal from the systemic circulation as PVR decreases. This is proposed as the major pathophysiological etiology for the frequent instability observed in the early postoperative period in these patients. In 2003, Sano et al. reported improvement in stage I survival in a small number of patients at one institution when pulmonary blood flow (PBF) was provided by a 5–6 mm PTFE graft from the RV to the PA, rather than via an mBTTS (Figure 30.4) [18, 21]. Because the RV–PA shunt arises proximal to the neo‐aortic valve, there is no flow from the aorta into the PAs during diastole, limiting diastolic run‐off and coronary ischemia. The resultant higher diastolic blood pressure has been hypothesized as the physiologic reason for improved survival. Figure 30.4 Norwood Stage I palliation for hypoplastic left heart syndrome with right ventricle to pulmonary artery shunt (Sano shunt). See text for details. (Source: Berenstain & Spaeth [18]. Reproduced with permission of Cambridge University Press.) The SVRT was designed to test the hypothesis that stage I survival would improve with the utilization of the RV–PA shunt, with an endpoint of death or transplantation in the first 12 months [7]. In all, 555 infants were randomized to receive either an mBTTS or RV–PA shunt. Approximately 10% of subjects required cross‐over from their randomly assigned shunt type to the other shunt type because of anatomic considerations. At 12 months, 36.4% of the mBTTS subjects had died or been transplanted, compared with 26.3% for the RV–PA shunt (P = 0.010). However, overall complication rates in the RV–PA shunt group were higher (72 vs. 46 per 100 infants, P = 0.03), and the number of infants experiencing one or more complications was also greater with the RV–PA shunt (39 vs. 28%, P = 0.03). After 12 months, the hazard ratio for death or transplantation did not differ between the two types of shunts. Follow‐up after 6 years demonstrated slightly higher transplant‐free survival (5%) but an increased need for catheter re‐interventions in the RV‐PA shunt patient group; however, there were no statistically significant differences in the hazard ratio for death or transplantation as well as catheter re‐interventions [22]. A recent meta‐analysis of 32 studies by Cao et al. assessed long‐term survival in HLHS with either mBTTS (n = 1348) or RV‐PA shunt (n = 1258). Although the RV‐PA shunt group had significantly higher in‐hospital survival and inter‐stage survival between stage I and II palliation until 2 years post‐stage I palliation, no significant differences in long‐term outcomes between stage II and stage III palliation were identified [23]. In contemporary practice, the shunt type used depends largely on the surgeon and institutional preference. In a publication specifically examining the rate and causes of 30‐day in‐hospital mortality in SVRT subjects, 16% of patients died during the initial hospitalization, with 12% mortality in the first 30 days after Norwood stage I palliation. Although after multivariable analysis shunt type was not identified as a significant risk factor for mortality, the presence of an mBTTS was an independent risk factor for cardiopulmonary resuscitation (CPR). Independent risk factors for both 30‐day and total in‐hospital mortality included low birth weight, genetic abnormality, post‐procedure need for extracorporeal membrane oxygenation (ECMO), and open sternum. Longer duration of DHCA was an independent risk factor for hospital mortality. Independent risk factors for major morbidity (renal failure, sepsis, increased duration of ventilation, and hospital stay) included genetic abnormality, lower center/surgeon volume, open sternum, and additional post‐Norwood operations [24]. Inter‐stage mortality occurring after the initial hospitalization for stage I palliation and during the 3–6 months prior to Stage II superior cavopulmonary connection (SCPC) has been a persistent issue. The physiology of the HLHS patients remains tenuous after the initial palliation, as a parallel circulation with all systemic and pulmonary output passing through the single systemic ventricle still persists. The Qp: Qs remains labile, and thus, coronary ischemia continues to be a risk. Significant hemodynamic perturbations may easily result from dehydration due to viral gastroenteritis, upper respiratory infections, or micro‐aspiration episodes caused by a recurrent laryngeal nerve injury that frequently accompanies stage I palliation. In the SVRT, of 426 subjects discharged from the hospital, 50 died inter‐stage (12%) [25]. The inter‐stage death rate was greater for mBTTS patients (18%) than for RV–PA shunt patients (6%, P < 0.001). Risk factors for inter‐stage death included gestational age <37 weeks, Hispanic ethnicity, AA/MA, a greater number of post‐Norwood complications, census block poverty level, and moderate to severe AVVR. Ahmed et al. recently developed a validated risk scoring system to predict mortality or transplant in single‐ventricle patients during the inter‐stage period between stage I and II palliation. Between 2008 and 2015, data from over 2,000 patients from the National Pediatric Cardiology Quality Improvement Collaborative Registry were analyzed to create a weighted scoring system. This novel system, known as the NEONATE score, assessed the following independent risk factors: Norwood type (mBTTS or hybrid vs. Sano shunt), postoperative ECMO, opioids required at discharge, no digoxin required at discharge, arch obstruction, ≥ moderate Tricuspid regurgitation, and extra oxygen plus ≥ moderate tricuspid regurgitation at discharge. During the inter‐stage period, the strongest predictor of death or transplant was ≥ moderate tricuspid regurgitation, which worsened if oxygen was required [26]. With a combined 28% mortality observed in the SVRT in the inter‐stage period between stages I and II, the challenges of caring for this group of patients are evident. Death after stage II SCPC in the first year of life is less common; the stabilizing effect on the pathophysiology of HLHS (see later) is the most significant factor. Figure 30.5 Giessen hybrid procedure for HLHS: stage I and stage II. Giessen hybrid procedure consists of bilateral pulmonary artery banding with ductal stenting in the neonatal period and comprehensive stage II procedure in the infancy period. The arrows show the direction of the bloodstream. BPAB, bilateral pulmonary artery banding. (Source: Yörüker & Akintürk [33]. Reproduced with permission of Elsevier.) Despite continuing improvements in the perioperative management of infants with HLHS, specific populations of patients remain at higher risk and demonstrate a more significant level of morbidity and mortality during and after surgical stage I palliation [27]. Risk factors include birth weight <2.5 kg, prematurity, ascending aortic size <2 mm, MS/AA anatomy, poor RV function, severe tricuspid regurgitation, restrictive atrial septum, obstructed pulmonary venous return, and the presence of additional cardiac or non‐cardiac anomalies [28–32]. Continuing advancements in interventional cardiology techniques have facilitated the development of alternative therapeutic strategies for these subpopulations of patients. Due to the utilization of both surgical and interventional catheterization procedures, these interventions are known as “hybrid” procedures. A hybrid procedure is performed in the catheterization laboratory, OR, or “hybrid” procedure suite. It consists of median sternotomy without CPB, surgical placement of bilateral PA bands, and placement of a stent in the PDA by interventional cardiology via access obtained in the main PA (see Figure 30.5) [33–35]. The atrial septum can also be stented if restrictive. Advantages of hybrid procedures include avoiding CPB and/or DHCA in the neonatal period and providing an extended waiting period for infants who require cardiac transplantation or who may be eligible for biventricular repair. The hybrid procedure may also be utilized to delay a planned Norwood operation or as a salvage procedure for patients unsuitable for early Norwood operation [35]. The hybrid procedure is further detailed in Chapter 34. Modifications to the hybrid procedure continue to occur as newer devices become available. One such example is the internal self‐expanding flow restrictor, which can be utilized in place of external, surgically placed PA bands, allowing all components of the hybrid procedure to be accomplished in the catheterization laboratory [36]. As with surgically palliated patients, inter‐stage mortality continues to be an issue after the hybrid procedure. Close echocardiographic monitoring for tricuspid valve regurgitation and/or RV dysfunction has been advocated, as these can indicate obstruction at the atrial level or through the PDA stent. Re‐interventions in the catheterization laboratory are not infrequently required to relieve such obstructions [37]. The most concerning and potentially life‐threatening complication involves the development of reverse or pre‐ductal coarctation, resulting in diminished coronary and cerebral perfusion. This can occur either secondary to uncovered ductal tissue that remains capable of closure or as a result of ductal stent placement. Once recognized, additional retrograde stent placement is utilized to address proximal aortic arch obstruction [38]. At the age of 3–6 months, aortic reconstruction, along with removal of the PA bands, ductal and atrial stents, repair of the PAs (if necessary), atrial septectomy, and SCPC, is performed. This is known as a “comprehensive stage II procedure” (See Figure 30.5). Patients subsequently progress to Fontan completion via a surgical approach or placement of a covered stent via catheter intervention [33, 39]. While previous small studies demonstrated no significant difference in survival statistics between the hybrid and traditional Norwood stage I palliation [40–43], a 2018 meta‐analysis of 14 studies comparing outcomes found that the hybrid group had significantly higher early mortality, reduced 6‐month and 1‐year transplant‐free survival rates, and an increased number of required re‐interventions [44]. However, some institutions preferentially perform hybrid procedures with outcomes comparable to those seen with the Norwood procedure [33, 44, 45]. According to the Society of Thoracic Surgeons Congenital Heart Surgery Database, 13 out of 100 institutions performed >50% of stage I palliations as a hybrid rather than Norwood procedure between 2010 and 2012 [46]. With continuing experience and technical modifications, the hybrid procedure may offer the potential for increased survival opportunity with early avoidance of CPB for certain groups including high‐risk patients, those awaiting cardiac transplantation, and those with the potential for future biventricular repair [47]. Given the broad spectrum of pathology observed in HLHS, with LV size ranging from rudimentary to mildly hypoplastic, the best surgical strategy may not always be clear. Patients with “borderline HLHS,” also known as “hypoplastic left heart complex,” or a “borderline LV” often pose particular management challenges, as either single‐ventricle palliation or a biventricular repair may be considered [48, 49]. In recent years, strategies to aid in determining which patients may be suitable for biventricular repair have been developed using echocardiographic and cardiac MRI parameters. While the use of these parameters may differ between institutions, mitral and aortic valve Z‐scores (Z‐score is standard deviation; e.g., a ‐2 Z score for a valve diameter is 2 standard deviations below normal for weight or body surface area) of between ‐5 and ‐2 (excluding atretic valves) are typical. Other critical factors include LV end‐diastolic pressure, LV end‐diastolic volume index, LV length and volume, and the degree of endocardial fibroelastosis (EFE) [50]. A group of 24 Congenital Heart Surgeons Society institutions studied 320 neonates who underwent either Norwood palliation or biventricular repair, leading to the development of hazard models based on various morphologic and functional characteristics [51]. Due to the high risk associated with the primary neonatal biventricular repair, many institutions choose a period of LV recruitment or staged LV rehabilitation, with initial SV palliation. This relieves obstructions and promotes PBF. Other techniques to stimulate LV growth, such as resection of endocardial fibroelastosis (EFE), mitral and/or aortic valvuloplasty, and ASD restriction, are also performed. Additionally, increasing PBF (via an mBTTS plus SCPC versus an RV‐PA shunt) after ventricular septation volume loads the LV and stimulates growth. If these techniques successfully promote adequate LV growth, subsequent take‐down of the SV palliation with conversion to a biventricular repair can occur [50, 52]. The success of biventricular conversion or repair is dependent on many factors, including appropriate patient selection and clinical and technical experience. Further evidence comparing long‐term outcomes between biventricular conversion versus progression with single‐ventricle physiology to Fontan completion is still being gathered [53]. The diagnosis of HLHS is most frequently made in utero. After an initial assessment, immediate treatment in the delivery room for HLHS patients includes initiation of a PGE1 infusion, placement of umbilical arterial and venous catheters, maintenance of spontaneous ventilation on room air, and transfer to a neonatal or cardiac intensive care unit (ICU). A postnatal transthoracic echocardiogram is performed to confirm the initial diagnosis and provide other crucial anatomic information, including LV size, presence of AVVR, degree of atrial septal restriction, or the presence of anomalous pulmonary venous return. Other less common but important details such as the presence of coronary artery fistulae or sinusoids to the LV should also be assessed. Patients with a restrictive atrial septum may require urgent or emergent intervention, requiring either balloon atrial septostomy or early surgical intervention. Cardiac catheterization is rarely performed for diagnostic purposes, and additional imaging (i.e., computed tomography [CT] or magnetic resonance imaging [MRI]) is seldom required unless anatomic questions remain. Certain anatomic and physiologic features have been identified as risk factors that may affect outcomes (see Table 30.1). Table 30.1 Preoperative risk factors prior to stage‐1 palliation Source: Roeleveld et al. [28]. Reproduced with permission of Cambridge University Press. The chest radiograph is followed daily to assess the heart size and status of the pulmonary vasculature. Common preoperative laboratory tests include complete blood count, coagulation studies, and blood type and cross‐match, along with the measurement of serum electrolytes, blood urea nitrogen, creatinine, and glucose. In a recent evaluation of standard preoperative laboratory testing for pediatric cardiac surgery patients in the US and Canada by the Congenital Cardiac Anesthesia Society Hemostasis Interest Group, 11 of 12 centers surveyed reported routinely obtaining preoperative testing. However, 83% agreed that only a type and screen and complete blood count were necessary. No centers reported changes in management due to preoperative testing, suggesting that preoperative testing requirements are controversial and subject to institutional preferences [54]. Ideally neonatal HLHS patients “self‐regulate” their Qp:Qs prior to stage I palliation, spontaneously breathing with a slightly elevated PaCO2. Diuretics are often used preoperatively to limit blood volume recirculating through the lungs. Nasal continuous positive airway pressure (CPAP) may be helpful to provide positive pressure to relieve respiratory distress and elevate the PVR while maintaining spontaneous respiration. Tracheal intubation and mechanical ventilation are reserved for the most severe cases of respiratory insufficiency or for patients with anatomic variants (e.g., restrictive intra‐atrial communication) that markedly diminish PBF. Early surgical intervention before severe derangements in Qp:Qs ensue is essential to prevent complications. Neonates presenting for stage I reconstruction (i.e., Norwood procedure with mBTTS or Sano shunt) receive an intravenous (IV) induction of anesthesia with meticulous attention to the hemodynamic consequences of the anesthetic agents selected. Phenylpiperidine‐based synthetic opioids, such as fentanyl, are generally preferred because they blunt the endogenous catecholamine response to noxious stimuli at doses that are usually tolerated hemodynamically [55–57]. However, even with these hemodynamically “neutral” agents, larger doses may result in significant cardiovascular changes, such as bradycardia and hypotension. These observations suggest that some endogenous catecholamine release is required in an infant with HLHS to sustain satisfactory hemodynamics. Unfortunately, the threshold narcotic dose that is “sufficient” and not “excessive” varies between patients, necessitating careful titration. Although clinical research conducted in the early 1990s popularized the view that very high doses of opioid analgesics should be administered perioperatively for stress hormone suppression in neonates undergoing cardiac surgery [58], efforts to duplicate these findings have not confirmed the original results [59]. The latter demonstrated that very high doses of fentanyl did not completely suppress the release of endogenous catecholamines, even in combination with benzodiazepine infusions. Additionally, outcome measures were no different in any of the study groups. Emerging evidence has demonstrated potential benefits of the use of dexmedetomidine in pediatric cardiac surgery, including neuroprotection as well as improved morbidity and mortality [60]. According to data from the Congenital Cardiac Anesthesia Society and Society of Thoracic Surgeons Cardiac Anesthesia Database Collaboration, dexmedetomidine use has steadily increased since 2010 [61]. Strategies vary significantly between institutions, with total intraoperative fentanyl doses ranging between 10–100 μg/kg, and the addition of low‐dose volatile anesthetics, benzodiazepines, ketamine, and dexmedetomidine in various combinations described as components of a balanced anesthetic approach to initial palliations for HLHS [62]. Most institutions now utilize fentanyl dosages in the lower range, generally <25 μg/kg. Management of the airway and ventilation assumes great importance during induction of anesthesia. Given the propensity for most neonates with HLHS to have excessive PBF, the anesthesiologist must take care not to employ ventilatory maneuvers that lower PVR, such as hyperventilation or utilization of high inspired concentrations of oxygen (FiO2). In an infant with typical HLHS physiology, manual ventilation should be initiated with room air or a low concentration of supplemental oxygen. The extent to which FiO2 is increased before laryngoscopy should depend upon the magnitude of hemodynamic response to the initiation of controlled ventilation. Infants who demonstrate a significant reduction in systemic arterial pressure despite low FiO2 may not tolerate prolonged exposure to a higher FiO2 without deleterious hemodynamic consequences. Intraoperative monitoring consists of standard cardiovascular, respiratory, and temperature monitors and continuous direct arterial pressure monitoring. An umbilical arterial line, if present, may be used to preserve other arterial access sites for future interventions. Most centers utilize central venous access as well. An umbilical venous catheter positioned in the orifice of the superior vena cava (SVC) at the time of surgery serves as a valuable monitor of mixed venous oxygen saturation, enabling more precise assessment of systemic CO and Qp: Qs [6, 63, 64]. Some institutions may place direct transthoracic atrial lines instead of percutaneous jugular or subclavian central venous pressure catheters due to concern regarding thrombosis in the central thoracic veins in SV patients. However, other institutions have failed to demonstrate the significant risk associated with the use of upper body central venous catheters even in SV patients [65]. Peripherally inserted central catheters (PICCs) are increasingly utilized as well. In a 2020 retrospective review of PICC use in pre‐stage II SV patients, only 2 out of 56 patients with PICCs developed detectable thrombus (4%) versus 3 out of 17 patients with central venous catheters (18%) [66]. Most centers utilize cerebral and somatic near‐infrared spectroscopy (NIRS) as additional non‐invasive monitors which correlate with SvO2. Cerebral NIRS can be used to guide ACP as it has been shown to detect alterations in CBF in neonates undergoing Norwood stage I palliation [67, 68]. The use of cerebral and somatic NIRS in the immediate postoperative period has also been shown to predict early mortality and to highlight the need for ECMO in HLHS patients [69]. Transesophageal echocardiography (TEE) is often utilized depending on the size of the neonate and institutional preference. Epicardial echocardiography after CPB can also be utilized and is particularly useful to assess AVVR and neo‐aortic regurgitation. Measures to clear airway secretions and assure complete re‐expansion of the pulmonary parenchyma are performed in the terminal phases of rewarming on bypass. The magnitude of reduction in the mean systemic arterial pressure that occurs with the opening of the shunt during the terminal phase of CPB can provide qualitative insights into what PVR might be expected in the early post‐CPB period. Initial ventilatory support should be adjusted accordingly. It is best to begin with a FiO2 between 0.6 and 1 and a pattern of ventilation designed to result in low‐normal PaCO2, recognizing that further adjustments will be indicated by the individual infant’s physiology. Assuming a technically satisfactory repair without unusual risk factors, PVR typically falls within the first few hours after surgery. Until then, some patients may potentially require high levels of supplemental oxygen in the immediate post‐CPB period. While the physiologic goals post‐CPB remain identical to those of the preoperative period, the variables affecting the achievement of these goals differ. The pulmonary circulation now resides either at the distal end of a restrictive prosthetic systemic‐to‐pulmonary shunt or an RV–PA shunt. A variety of subtleties, including technical execution of the shunt, relief of atrial obstruction, and the pre‐existing condition of the pulmonary circulation, can render the ultimate physiology unpredictable. When a Sano shunt has been performed, technical issues of graft insertion, proper graft size (particularly in the neonate <2.0 kg), and the ability to delineate the etiology of insufficient PBF at the termination of CPB can introduce additional complexities. Even with a perfect technical result, the Norwood operation does not reduce the volume or pressure burden on the SV. Supporting and maintaining the physiology of parallel systemic and pulmonary circulations with a Qp: Qs of 1.0 remain the objective throughout the post‐CPB period. Postoperatively, the heart has incurred the cost of insults related to CPB, cessation of coronary perfusion, and DHCA, which may contribute to the cardiovascular frailty often exhibited in the early postoperative period. However, in the absence of major deficiencies in myocardial protection or persistent anatomic residua, such as significant aortic arch obstruction, coronary compromise, or valvular insufficiency, myocardial dysfunction can usually be addressed with relatively modest doses of inotropic agents and vasodilation. Low dose epinephrine (0.03–0.05 mcg/kg/min) and milrinone (0.25–0.5 mcg/kg/min) are commonly utilized after CPB. Vasopressin infusion may be added as well if necessary. While some clinicians advocate routine use of perioperative milrinone in Norwood procedures, others propose a targeted approach, citing the relatively weak inotropic and lusitropic effects of milrinone compared to its vasodilating properties. There is also conflicting evidence regarding the efficacy of perioperative milrinone in reducing mortality after the Norwood procedure [70–72]. In many neonates and young infants undergoing major cardiac interventions, myocardial performance may deteriorate in the first 6–12 hours postoperatively before it begins to improve. As a result, measures to reduce metabolic demands by providing continuous neuromuscular blockade and opioid infusions may be utilized in the immediate postoperative period. The sternum may be left open, covered by SilasticTM or other synthetic material, and stabilized with a plastic strut. This may improve hemodynamic stability as mediastinal edema is frequent in the first several postoperative days. The potentially deleterious effects of positive pressure ventilation are also mitigated. The open sternum also provides access for postoperative mediastinal exploration for bleeding, or the institution of ECMO. Sternal closure can be accomplished at the bedside 24–72 hours postoperatively. A variety of ultrafiltration techniques, including conventional, modified, zero‐balance, and combination ultrafiltration, may be utilized during and after CPB to improve postoperative hemodynamics. Several studies have demonstrated the beneficial effects of ultrafiltration upon hematocrit, hemodynamics, hemostasis, pulmonary function, and central nervous system recovery [73–78]. Perioperative weight gain is significantly reduced, as are the levels of specific inflammatory mediators. Occasionally, the position of the bypass cannulae or the continuous flux of blood through the modified ultrafiltration (MUF) circuit results in unfavorable hemodynamic changes precluding completion of the ultrafiltration. In 99 consecutive patients undergoing stage I palliation between September 2000 and August 2002, all tolerated the hemodynamic perturbations of MUF [79]. Clinical outcomes, however, have not consistently been improved and no single method of ultrafiltration has proven superior over others [73, 80]. Some institutions routinely place peritoneal dialysis (PD) catheters in the OR, and utilize PD in the early postoperative period for removal of excess interstitial fluid and potentially to remove inflammatory mediators. Rapid restoration of normal hemostasis is an essential objective in the post‐CPB period, particularly as the creation of the neo‐aorta in Stage I reconstruction requires substantial arterial suture lines, and cyanotic heart disease is a predictor of postoperative blood loss [81]. Following the completion of MUF and confirmation of satisfactory technical and physiologic repair, heparin effect is reversed with protamine. Despite the theoretical advantages of fresh whole blood, studies have not demonstrated benefit from this strategy relative to the use of reconstituted whole blood [82]. Additionally, fresh whole blood is not available at most institutions. Gruenwald et al. demonstrated that a reconstituted fresh whole blood bypass prime, consisting of packed red blood cells (PRBCs), fresh frozen plasma, and platelets from the same donor, <7 days old, followed by a second such unit given after bypass, reduced bleeding in infants undergoing surgery compared with standard blood component therapy [83]. Should these measures fail to achieve adequate hemostasis despite the elimination of most surgical bleeding sites, laboratory testing (platelet count and function, fibrinogen level, and viscoelastic testing) should be conducted to direct component therapy. Data specifically examining procoagulant medications in infants undergoing stage I reconstruction are sparse. Antifibrinolytic therapies have demonstrated efficacy in infants and children following heart surgery, but data in neonates continues to be very limited [84–87]. Although aprotinin, a serine protease inhibitor that acts as an antifibrinolytic agent with anti‐inflammatory properties, demonstrated effectiveness comparable to other antifibrinolytics in infants and children [88]; this agent was voluntarily removed from the market when it was linked to greater 5‐year mortality following coronary bypass grafting [89]. The lysine analogs epsilon aminocaproic acid and tranexamic acid appear to have comparable efficacy [84, 87], and extensive experience implying safety, making them appropriate substitutes for aprotinin [90]. Recombinant activated factor VII (rFVIIa) has been used as rescue therapy in infants and children following cardiac surgery, including neonates undergoing stage I reconstruction when conventional correction of postoperative coagulopathy with blood product transfusions fails to control hemorrhage [91, 92]. While rFVIIa can aid in controlling intractable bleeding, caution is warranted, as a recent retrospective review of 149 infants and children who received rFVIIa during cardiac surgery demonstrated an increased risk of thrombosis compared to control patients (20% vs. 8%) [93]. Another retrospective review of 414 neonates who underwent cardiac surgery found a 22% frequency of thrombosis in patients who received rFVIIa compared to a 6% incidence in patients who did not receive it. In neonates who underwent the Norwood procedure, those who received rFVIIa and developed thrombus had significantly higher mortality and incidence of requiring ECMO [94]. Recombinant factor VIIa may be capable of producing a hypercoagulable state that poses risks to certain surgical repair elements, including prosthetic shunts. Prothrombin complex concentrates (PCCs) may pose a similar thrombotic risk and should be used cautiously in patients undergoing stage I palliation. The 2019 patient blood management guidelines from the Network for the Advancement of Patient Blood Management, Haemostasis, and Thrombosis (NATA) for neonates and children undergoing cardiac surgery recommend against the use of PCCs in pediatric cardiac surgery except in clinical trials due to insufficient efficacy and safety data [95]. Point‐of‐care viscoelastic tests and transfusion algorithms are increasingly used in pediatric cardiac surgery [95–97]. Cyanotic patients, including those with HLHS, are known to have abnormal thromboelastography parameters compared to normal children, with these coagulation imbalances predisposing to both bleeding and thrombosis [96, 98]. The development of institution‐specific, target‐population transfusion algorithms may lead to reduced intraoperative transfusions and improved outcomes. However, additional data regarding patients undergoing stage I palliation is needed [96, 97, 99]. As PRBC transfusion has been associated with a longer duration of mechanical ventilation and ICU stay in stage I palliation patients [100], the Pediatric Critical Care Transfusion and Anemia Expertise Initiative released recommendations regarding red blood cell transfusion in patients with CHD. These recommendations state transfusions should be avoided “solely based upon hemoglobin, if hemoglobin is >9.0 g/dL in infants undergoing staged palliative procedures with stable hemodynamics” [101]. Further details of coagulation and bleeding management are discussed in Chapter 16. Figure 30.6 Pathophysiology associated with changes in oxygen saturation for shunt‐dependent single ventricle heart defects. The figure illustrates differential diagnoses to consider when oxygen saturation thresholds are breached after Norwood palliation with a modified Blalock‐Taussig shunt for hypoplastic left heart syndrome. Similar pathophysiologic considerations are applicable to the right ventricle to pulmonary artery shunt, hybrid palliation, and other shunt‐dependent single ventricle variants. (Source: Rudd et al. [104]. Reproduced with permission from Texas Children’s Hospital. © 2019 Texas Children’s Hospital.) Excessive hypoxemia represents one of the more commonly encountered problems in the early post‐CPB period. Although inadequate Qp:Qs is often the cause, factors that impair systemic oxygen delivery and reduce mixed venous oxygen saturation can also occur [63, 64, 102, 103]. A progressive increase in systemic oxygen saturation is often observed during MUF, likely due to the effect of hemoconcentration and the resulting increased oxygen delivery on mixed venous oxygen saturation. Post‐CPB, measures directed at maintaining the hematocrit above 40–45% may alleviate excessive demands placed upon the recovering heart to increase systemic output. The distinction between systemic hypoxemia due to low Qp:Qs, low pulmonary venous oxygen saturation, or low mixed venous oxygen saturation (SvO2) is crucial as the therapies for each differ. Measures designed to reduce PVR will impose a further volume load on a heart already struggling to provide marginal systemic perfusion. Patients demonstrating low SvO2 are better served with therapies that promote systemic output, such as inotropic agents or vasodilators (see Figure 30.6) [104]. Similarly, infants with low pulmonary venous oxygen saturation require a strategy of ventilatory support designed to reduce atelectasis and promote gas exchange in impaired alveoli. Although pulmonary vein desaturation is not the most common cause of persistent systemic hypoxemia after stage I palliation, one series found pulmonary vein desaturation in as many as 30% of patients [105]. Unfortunately, this diagnosis is rarely made definitively in the OR or cardiac ICU due to the logistical challenges of blood sampling from the pulmonary veins. Intraoperatively, expectant measures directed at expansion of the lungs and maintenance of normal functional residual capacity (FRC) usually suffice to avoid pulmonary vein desaturation. When systemic hypoxemia is suspected to be due to low Qp: Qs, other manifestations can provide supporting evidence. A trial opening of the systemic‐to‐pulmonary artery shunt during the latter phases of rewarming on CPB may have failed to demonstrate a significant drop in the mean systemic arterial pressure, and early post‐CPB hemodynamics may have revealed a relatively narrow pulse pressure and/or high diastolic pressure. A substantial discrepancy may exist between arterial and end‐tidal CO2 measurements. These suggestive pieces of inferential evidence can be confirmed by aortic Doppler flow analysis or calculation of a Fick ratio using oxygen saturation determinations. Most commonly, diminished PBF may reflect a subtle technical aspect of the arch reconstruction, innominate artery dimension, or shunt placement. However, specific patient subsets can exhibit profound abnormalities in the pulmonary vasculature that result in excessive PVR elevations. Neonates born with HLHS and severe pulmonary venous obstruction due to intact atrial septum without alternative decompressing veins routinely demonstrate extremely high and volatile PVR. Even the typical HLHS anatomic constellation is associated with marked abnormalities in the number and muscularization of the pulmonary vasculature by pathologic examination. Hypotheses attribute these changes to chronic fetal pulmonary venous obstruction [106, 107]. One can speculate that these changes become more extreme in the context of the significant obstruction caused by HLHS with an intact atrial septum. Fetal echocardiography has confirmed alteration in pulmonary venous flow pattern in relation to the magnitude of restriction at the atrial septum [108]. In the context of hypoxemia due to low Qp:Qs, interventions fall into three categories: surgical modification, pulmonary vasodilation measures, and systemic vasoconstriction. In the subgroup of patients expected to have unusually elevated PVR, surgical modifications might entail placement of a larger shunt or placement of a central shunt (PTFE shunt from the aorta to the pulmonary artery). Potential pulmonary vasodilator therapies include the strategies one might employ in any patient demonstrating elevated PVR, such as supplemental oxygen, moderate hyperventilation, normothermia, alkali, and inhaled nitric oxide (iNO) [109–111]. Additionally, the use of higher doses of inotropic infusions or even vasoconstrictors to increase the driving pressure across the shunt may be considered. The latter necessitates careful monitoring to avoid jeopardizing perfusion to other vital organs. The depressed myocardial function represents another potential problem in the early post‐CPB period. Some degree of myocardial dysfunction typically occurs following stage I palliation, as no hemodynamic benefit is achieved that offsets the cost of CPB and an ischemic interval. When this dysfunction becomes more significant than usual, specific causes should be sought. Even in the context of the typical conduct of stage I reconstruction, the presence of AA can make routine myocardial protection measures, such as the infusion of cardioplegia solutions, challenging, and thus inadequate myocardial preservation represents one potential cause for persistent or excessive myocardial depression. Technical considerations represent the predominant cause of myocardial dysfunction following this complex intervention. One of the most complex aspects of this procedure is the reconstruction of an aortic arch so that the small ascending aorta, which principally serves to provide coronary flow, is not compromised. Problems with retrograde flow to the coronary arteries may not become evident until the cardiac volume is restored in anticipation of terminating CPB. Residual hemodynamic derangement represents another potential cause of myocardial dysfunction. Given that under the best circumstances, the Norwood operation does not provide appreciable hemodynamic benefit, one would expect that a surgical result with newly imposed volume or pressure loads would be poorly tolerated. Examples of such findings include residual aortic arch obstruction, AV valve dysfunction, and/or semilunar valve obstruction or regurgitation. Metabolic disturbances can also result in significant myocardial dysfunction. A fragile RV struggling to cope with significantly increased volume output demands at systemic pressure is perhaps more susceptible to what might otherwise be modest metabolic disturbances. As such, variables that can impact myocardial performance, such as ionized calcium levels and the presence of lactic acidosis, should be monitored closely and observed for trends. The rapid administration of blood products containing calcium‐binding drugs, high levels of potassium, and lactic acid, along with other vasoactive mediators can result in an acute, profound deterioration in cardiac performance in the early postoperative period. When the arterial pH falls below 7.3, myocardial performance may deteriorate and contribute to a further reduction in Qs. The administration of IV bicarbonate, calculated to eliminate the base deficit completely, often exerts a beneficial effect on both myocardial performance and Qs. In addition to the inherent cardiac sensitivity, certain anatomic factors accentuate this vulnerability to metabolic disturbances. Blood carrying the transfused products from the systemic venous circulation enters the RV and is directed immediately to the reconstructed aorta, which has as its first branch the coronary arterial circulation. Thus, constituents of the transfused blood (e.g., citrate, potassium, lactate) infused into the venous circulation arrive at the coronary arteries with greater speed and more concentration than might have occurred had they been dissipated throughout the pulmonary vasculature before entering the aorta. This effect is further accentuated if central venous catheters are employed to infuse the blood product. It is best to utilize either washed packed cells or blood that is <7 days of age. Arrhythmias most commonly occur as manifestations of the problems described previously. When they occur early in the rewarming process on CPB, coronary insufficiency represents the most common cause, particularly if the arrhythmia is ventricular in origin. Metabolic disturbances produce the same qualitative rhythm changes seen in normal hearts, although the manifestations might be more extreme. Given the predominantly extracardiac nature of the Norwood procedure, acquired heart block rarely follows this operation unless it existed preoperatively. On rare occasions, a patient presents with HLHS and a primary arrhythmia, such as Wolff–Parkinson–White syndrome. A 2021 retrospective review of 120 patients with HLHS after the Norwood procedure demonstrated a 26% incidence of postoperative arrhythmias. Of these, the majority (32.4%) were ectopic atrial tachycardia [112]. Excessive PBF may complicate the early postoperative period; however, this diagnosis should be entertained cautiously. In many instances, the apparent excess PBF reflects a relative imbalance with respect to significantly diminished systemic CO (Qs). The latter should be specifically excluded and addressed before invoking extreme measures to restrict PBF. Of course, subtle technical differences in the conduct of the operation can result in an anatomic propensity to an excessive Qp: Qs, which can, in turn, jeopardize systemic perfusion. Such patients typically exhibit an extremely wide pulse pressure or low diastolic pressure, reflecting pulmonary “run‐off.” If myocardial performance otherwise appears robust, specific measures employed to increase PVR are appropriate in this setting. In most patients, this condition dissipates as the infant recovers from surgery. Should the problem persist beyond the first postoperative day, cardiac catheterization should be considered to evaluate the need for further surgical intervention to diminish PBF. The subset of patients who demonstrate either an inability to separate from CPB or refractory cardiac dysfunction postoperatively may benefit from ECMO utilization as a support strategy. Ungerleider et al. have advocated the routine use of mechanical ventricular assistance in all patients following stage I reconstruction, reporting 89% survival to hospital discharge [113]. According to data from the National Pediatric Cardiology Quality Improvement Initiative database, 66 of 931 (7.1%) HLHS patients required ECMO during admission for stage I palliation. Patients requiring ECMO had a significantly higher risk of death or transplant (18%) compared to those who did not require ECMO (9%) [114]. Risk factors described for post‐Norwood ECMO requirement include birth weight <2.5 kg, longer CPB time, peak serum lactate >9 mmol/L, and the vasoactive inotropic score of >27 [115]. An analysis from the Extracorporeal Life Support Organization of 738 HLHS patients requiring ECMO after stage I palliation demonstrated a 31% survival rate. Longer duration of mechanical ventilation before ECMO, longer duration of ECMO support, and ECMO complications such as renal failure or neurologic injury, all increased the risk of mortality [116, 117]. The risk of cardiac arrest in neonates with HLHS remains a concern in stage I postoperatively and the inter‐stage period until SCPC is performed [118]. According to the Society of Thoracic Surgeons Congenital Heart Surgery Database, there was a 12.7% incidence of cardiac arrest among stage I palliation patients between 2007 and 2012. CPR in this population is also difficult, with a 62.3% mortality rate compared to 12.5% mortality in patients who did not experience an arrest [119]. The 2020 American Heart Association Pediatric Advanced Life Support guidelines include a specific section regarding the resuscitation of patients with SV physiology [120]. In stage I palliated patients, chest compressions provide both pulmonary and systemic blood flow, while chest recoil increases filling to the SV. Due to the parallel circulation, systemic blood flow will be lower and may have a low oxygen content, leading to coronary ischemia, systemic hypoperfusion, and end‐organ damage [118]. Cardiac arrest and resuscitation are discussed in greater detail in Chapter 25. The European Association for Cardio‐Thoracic Surgery (EACTS) and the Association for European Paediatric and Congenital Cardiology (AEPC) recently developed a set of guidelines for managing neonates and infants with HLHS. These guidelines include suggested management for diagnosis, imaging, preoperative assessment, surgical and anesthetic management, and inter‐stage monitoring. These recommendations allow practice standardization and identify potential targets for practice improvement at individual institutions [121] TA is a cyanotic congenital heart defect characterized by total agenesis or complete obstruction of the AV valve associated with the morphologic RV. Tricuspid atresia accounts for approximately 1% of all cases of CHD. It is the fourth most common form of cyanotic CHD, following transposition of the great arteries, Tetralogy of Fallot, and HLHS. Its incidence, similar in males and females [122], is 1 per 10,000 individuals [123]. Tricuspid atresia occurs due to the disruption of tricuspid valvulogenesis, a multistep process, and genetic mutations in specific pathways (NFATC1 gene, Wnt/beta‐catenin, RASA1, and Ras/ERK cascade) have been proposed to underlie certain cases of TA [124, 125]. Tricuspid atresia results in the absence of the RV inlet and varying deficiency of the trabecular portion of the RV, leaving the RV composed mainly of an infundibular portion and resulting in a functionally single LV (Figure 30.7) [126]. Other anatomic characteristics of TA include the presence of an interatrial defect (either ASD or PFO) and an enlarged left‐sided AV valve. A ventricular septal defect (VSD) is nearly universally present, and abnormalities such as pulmonary stenosis (PS) may occur as well. In cases where a VSD is not present or pulmonary outflow obstruction is complete, the patient’s PBF will rely on the presence of a PDA. The amount of PBF is determined by the degree of pulmonary outflow tract obstruction, the presence and size of a VSD, the relationship of the great arteries, and the presence of a PDA. Figure 30.7 Blood flow in tricuspid atresia. (A) Normal cardiac anatomy. (B), Tricuspid atresia. *, patent foramen ovale or atrial septal defect. ASD, atrial septal defect; TA, tricuspid atresia. (Source: Sumal et al. [126] / John Wiley & Sons / CC BY 4.0.) The timing and presentation of TA are determined by the degree of PBF, with approximately 50% of patients presenting on the first day of life and 80% by the age of 1 month. Without intervention, TA is associated with 90% mortality by the end of the first year of life [127]. The majority of patients present with cyanosis due to decreased PBF secondary to pulmonary obstruction and/or a small VSD. Patients with increased PBF secondary to shunting across a VSD and unobstructed PBF may initially have minimal cyanosis and progress to develop signs of excessive PBF and congestive heart failure (CHF) as the PVR falls after birth. Patients with less severe PS generally have a milder degree of cyanosis and may not require immediate surgical intervention as they have relatively balanced systemic and pulmonary circulations (see Figure 30.8) [128]. The Fontan procedure was initially applied to patients with TA, and many long‐term adult survivors remain from the early Fontan era. Although different classifications have been proposed for TA, the most widely accepted schemes are based upon the relationship of the great arteries (Type I, II, or III) and the degree of pulmonary outflow obstruction (subclassification a, b, or c) (see Figure 30.9) [129]. Table 30.2 summarizes the anatomic classification, incidence, and pathophysiological features of TA. Type I: Great artery relationships are normal. This is the most common type of TA, affecting 70–80% of patients. In the absence of a PDA, PBF is primarily dependent upon the size of the VSD and the severity of PS. Type II: D‐transposition of the great arteries exists. Type II affects approximately 25% of patients. Due to the transposed great arteries, the systemic outflow is dependent on the size of the VSD. Therefore, a small or restrictive VSD often results in aortic stenosis, arch hypoplasia, or coarctation of the aorta. Systemic hypoperfusion occurs as the PDA narrows and PGE1 infusion may be necessary to maintain ductal patency. Obstruction to PBF is unusual in these patients, and they frequently develop pulmonary overcirculation and CHF in the first few weeks of life. Figure 30.8 Hemodynamics of Type I tricuspid atresia with (A) no pulmonic stenosis (PS); (B) severe PS; and (C) “well‐balanced” PS. Qp, pulmonary blood flow; Qs, systemic blood flow. Numbers with percentages are oxyhemoglobin saturation values. (Source: Taggart [128]. Reproduced with permission of John Wiley & Sons.) Type III: Malpositions of the great arteries other than d‐transposition . This type is less commonly seen (3–6% of patients) and is used to classify TA with complex associated malformations such as AV septal defect or truncus arteriosus. The pathophysiology of TA is determined by the degree of anatomic obstruction to PBF, the PVR, and the patency of the ductus arteriosus. The size of the VSD and the degree of PS (if present) will determine the amount of antegrade PBF that can occur left‐to‐right via the VSD, from the LV to the RV and the pulmonary arteries. Without communication between the RA and the RV, deoxygenated systemic venous return from the RA must travel to the LA through the ASD or PFO. Systemic arterial desaturation is present in all patients with TA due to the obligatory mixing of all venous returns (systemic, pulmonary, and coronary) in the LA. An unrestricted atrial defect is crucial to allow both venous mixing and adequate CO. If the ASD or PFO is restrictive after birth, an urgent atrial septostomy must be performed to enlarge it. Blood may also enter the PA from the aorta via the PDA. Figure 30.9 Classification of tricuspid atresia. (A) TA with normal great arteries (69%). (B) TA with D‐TGA (28%). (C) TA with great‐artery position other than dextro‐transposition. L‐TGA (subtype 1) is shown (3%). (D) TA with persistent truncus arteriosus. D‐TGA, dextro‐transposition of the great arteries; L‐TGA, levo‐transposition of the great arteries; TA, tricuspid atresia. (Source: Taggart [128]. Reproduced with permission of John Wiley & Sons.) The diagnosis of TA is generally made prenatally with obstetrical screening ultrasound or fetal echocardiography. Postnatal diagnosis is confirmed with history and physical examination, chest radiography, electrocardiogram (ECG), and echocardiography. Chest radiography can differentiate between patients with high or low PBF as those with increased PBF demonstrate cardiomegaly and prominent pulmonary vasculature. In contrast, patients with pulmonary oligemia have a normal‐sized heart and concavity in the region of the PA. The ECG demonstrates left axis deviation in the frontal plane. Echocardiography describes the anatomy and allows detailed physiological assessment and surgical planning. It aids in defining the size of the VSD and the RV, the relationship of the great vessels, degree of PS, presence and size of the PDA, and the amount of blood flow across these areas. The presence of other associated anomalies such as arch obstruction can also be assessed. Color‐flow and spectral Doppler echocardiography can evaluate the adequacy of the atrial level communication (ASD or PFO). Cardiac catheterization is rarely required in the neonatal period unless necessary for balloon atrial septostomy or stenting of the PDA. Cardiac MR and CT may be used occasionally to define unusual anatomy. Ultimately TA patients will undergo staged SV palliative surgeries, culminating in the Fontan procedure. However, the necessity and type of neonatal intervention differ according to the anatomical classification and pathophysiologic consequences. The need for neonatal surgical or catheter‐based intervention depends upon the degree of obstruction to PBF, the size of the VSD, and the resulting level of cyanosis. Subgroups of neonates with decreased PBF either due to pulmonary outflow obstruction and/or a restrictive VSD will require the provision of a stable source of PBF. Infants presenting with Type Ia or IIa (pulmonary atresia) are ductal‐dependent until they undergo either surgical placement of a mBTTS or stenting of the PDA in the cardiac catheterization laboratory. Patients with increased PBF due to an unrestrictive VSD and either mildly obstructed (Types Ib and IIb) or unobstructed pulmonary outflow (Types Ic and IIc) often develop CHF with signs of compromised systemic perfusion. Placement of a PAB for restriction of PBF may be necessary until a superior cavopulmonary connection (SCPC) can be performed at 3–6 months of age. Infants with relatively balanced systemic and pulmonary circulations (Types Ib and IIb), although cyanotic, may not require neonatal surgical intervention and may be followed clinically for increasing cyanosis. Most often, SCPC is their first palliative surgery. Type II TA patients with restrictive VSDs or significant subaortic obstruction require a PDA to provide systemic circulation and may undergo a “palliative” arterial switch operation (ASO) to place the aorta so that the unobstructed LV ejects into it; the VSD may need to be enlarged in this case to prevent profound cyanosis [130, 131] (See Chapter 29 for a detailed discussion of the ASO). If subaortic obstruction exists despite an unrestrictive VSD, patients may undergo a Damus–Kaye–Stansel (DKS) operation, which bypasses subaortic obstruction by creating an end‐to‐side anastomosis between the ascending aorta and the proximal stump of the divided PA. Either a mBTTS or RV‐PA shunt is placed to provide PBF. The operative and anesthetic considerations for this surgery are similar to those for the Norwood stage I palliation of HLHS. Figure 30.10 demonstrates surgical options for TA type II with significant subaortic stenosis. Neonates with pulmonary overcirculation and varying degrees of CHF may present for PAB placement to protect the pulmonary vascular bed from high pressure to facilitate successful progression to SCPC and Fontan physiology. Cyanosis is mild in these patients; they are generally tachypneic and often receive diuretic therapy. Preoperative evaluation should include a thorough history and physical examination, a review of the chest radiograph for evaluation of heart size and degree of pulmonary overcirculation, and baseline pulse oximetry. A current hemoglobin level will aid in the assessment of the degree of chronic cyanosis, and serum electrolyte values can evaluate abnormalities due to chronic diuretic therapy. A review of the most recent transthoracic echocardiogram should reveal precise details of the anatomy and functional characteristics, including any mitral valve regurgitation. Although cardiac rhythm disturbances are unusual at this stage, a 12‐lead ECG is helpful to exclude arrhythmias. Table 30.2 Anatomic and physiologic classification of tricuspid atresia ASO, arterial switch operation; CHF, congestive heart failure; DKS, Damus–Kaye–Stansel operation;mBTS, modified Blalock–Taussig shunt; PS, pulmonic stenosis; subAS, subaortic stenosis; VS, ventricular septum; VSD, ventricular septal defect; +, mild; ++, moderate; +++, severe; −, no effect. After placement of standard monitors, an IV induction of anesthesia is most commonly performed, utilizing fentanyl in combination with incremental doses of either ketamine or propofol. After administration of a neuromuscular blocking agent and tracheal intubation arterial access is achieved for monitoring. Institutional viewpoints vary regarding the need and best location for placement of central venous access. Some centers advocate the use of femoral venous cannulation, due to concern for potential thrombus formation in the SVC, as it will be necessary later for successful SCPC. Others may choose to place a transthoracic RA line. If appropriate peripheral IV access exists some centers elect not to use central venous access for this procedure. The patient’s preoperative condition, along with institutional preferences and the need for postoperative vascular access in the ICU, will assist in decision‐making. Steady‐state anesthetic and ventilatory conditions should be maintained during the procedure in order to optimize PAB adjustments. The FiO2 and PaCO2 at the time of PAB placement should approximate baseline conditions for the patient; communication between the surgeon and anesthesiologist regarding the desired conditions is essential. A FiO2 of 0.30 or less is generally utilized, and PaCO2 is often in the mid‐40s mmHg due to the metabolic alkalosis from diuretic therapy and the mild state of respiratory insufficiency from CHF. Many centers use TEE guidance during PAB placement. The operation is usually performed through a median sternotomy without CPB. After dissection, the surgeon places a thin cotton tape or strip of Gore‐Tex around the main PA, using care not to impinge on the branch PAs or pulmonary valve. A formula derived by Trusler advocated an initial band circumference of 24 mm + 1 mm/kg body weight for infants with bidirectional mixing disorders, but this was subsequently modified for patients with univentricular physiology, narrowing the circumference further to 22 mm + 1 mm/kg [132, 133]. Various methods may be used to assess and adjust PAB tightness. The primary method is to assess the change in pulse oximeter saturation and the measured arterial PaO2 saturation after band placement, with a SpO2 of 75–85% and a PaO2 of approximately 40–45 mmHg customarily targeted. Transesophageal echocardiography helps measure peak velocity (V) across the PA band; 3 m/s is a frequent goal and corresponds to a pressure gradient of 36 mmHg using the modified Bernoulli principle (peak velocity = 4V2). Additionally, echocardiography can reassure that no encroachment on the pulmonary valve or distortion of the pulmonary arteries has occurred. Finally, direct pressure measurement in the surgical field is utilized by some institutions; a PA pressure distal to the PAB that is about one‐third of systemic arterial pressure (range, 25–50%) is a frequent goal. The PAB is adjusted temporarily with surgical clips, blood gas measurements can be obtained after steady‐state conditions are achieved, and the band is then permanently secured with non‐absorbable sutures. An overly tight PAB will produce profound cyanosis and bradycardia, while one that is too loose will produce little change from baseline conditions. The final PAB circumference is often determined by surgical judgment, hence the importance of achieving and maintaining steady‐state anesthetic and ventilatory conditions. Although usually not necessary, inotropic support should be readily available as afterload will increase after placement of the PAB once the pulmonary circuit no longer provides a low resistance “pop‐off.” Placement of a PAB mechanically increases the resistance to PA flow, and therefore, more blood flow is directed out through the aorta. Systemic arterial pressure often increases after placement of a PAB, and occasionally a vasodilator such as sodium nitroprusside is necessary to control blood pressure. After surgery, tracheal extubation may occur in the OR or within the first few hours of ICU admission. Figure 30.10 Surgical methods for tricuspid atresia type II with significant subaortic stenosis. (A) Preoperative anatomy of tricuspid atresia type IIc. (B) Direct resection of subaortic myocardium with enlargement of the ventricular septal defect. (C) Damus–Kaye–Stansel operation: main pulmonary artery to aorta end‐to‐side anastomosis. (D) Palliative arterial switch operation. Ao, aorta, LA, left atrium; LV, left ventricle; PA, pulmonary artery; RA, right atrium; RV, right ventricle. As the patient emerges from anesthesia, CO changes, along with the relative tightness of the band. As infants are in a period of rapid somatic and cardiac growth, they often “outgrow” the PAB, and cyanosis increases. As nearly all of these infants undergo an SCPC within a few months, this issue is not usually problematic in the infant SV population. Patients with TA and either pulmonary atresia or significant PS who require PGE1 and maintenance of ductal patency for PBF may present for either surgical placement of a mBTTS or placement of a ductal stent in the cardiac catheterization laboratory to establish a stable source of PBF. Occasionally an older infant with profound cyanosis and a closed ductus arteriosus may also present for placement of an mBTTS. Although an mBTTS can be performed via right thoracotomy, the median sternotomy approach is most commonly utilized. Unless necessary due to hemodynamic instability or significant hemoglobin‐oxygen saturations during the procedure, CPB is not utilized. The anesthetic considerations for placement of an mBTTS are presented in detail in Chapter 28. Percutaneous transcatheter stenting of the ductus arteriosus was first described in 1992 and has continued to increase in prevalence as an alternative palliative approach to secure PBF in patients with duct‐dependent lesions [134]. Ductal stenting (DS) has proven to be a reasonable alternative to the mBTTS, as it is associated with similar or improved symmetrical PA growth, fewer procedural complications, a shorter ICU and hospital length of stay, and improved survival compared to surgical shunt [135, 136]. In comparing the two procedures, cardiac arrest, the need for extracorporeal circulatory support, arrhythmias requiring therapy, and bleeding were observed only in the mBTTS group, while access site injury was observed in the DS group [137]. As the experience continues to grow and early technical challenges are overcome, DS is emerging as a preferred alternative to the mBTTS. Further detail about ductal stenting is presented in Chapter 34. Unplanned reinterventions to treat cyanosis before SCPC may be required in patients with either mBTTS or DS. These interventions most commonly involve either balloon or stent angioplasty of the PDA stent or mBTTS, or surgical revision [136]. Planned reinterventions may also occur in DS patients, with reexpansion of the stented ductus allowing adjustment of PBF over time. A study comparing pre‐SCPC hemodynamics and PA growth in SV patients palliated with either DS or mBTTS found no significant differences between the two [138]. Management of patients with unrestrictive PBF and systemic ventricular outflow tract obstruction (TA with associated TGA) remains challenging. Systemic blood flow must pass through the VSD, or bulboventricular foramen, to a rudimentary subaortic outlet chamber. The VSD may become restrictive, limiting blood flow, or ventricular hypertrophy after placement of a PAB may further increase outflow obstruction. While early relief of obstruction is essential, surgical management of this issue has not been uniform. Although subaortic stenosis may be relieved directly by means of VSD and/or subaortic chamber enlargement, this incurs the risks of heart block, recurrent stenosis, and ventricular dysfunction due to the ventriculotomy. As noted earlier, the DKS operation may be performed for TA variants with significant subaortic or aortic obstruction or with a restrictive VSD. The anesthetic considerations are very similar to those for Norwood stage I palliation, as discussed earlier. Because the single functional ventricle is an LV, and because the aortic valve is not atretic and the coronary artery circulation not as tenuous as in HLHS, these patients are generally expected to have a more stable pathophysiologic condition after DKS, compared with the Norwood stage I palliation. The palliative ASO has many anesthetic considerations in common with the standard ASO for d‐TGA with two ventricles (discussed in Chapter 29). The major difference is that TA remains, and adequate PBF needs to be assured after the ASO, often requiring enlargement of the VSD or possibly placement of an mBTTS.
CHAPTER 30
Anesthesia for the Patient with a Single Ventricle
Introduction
Hypoplastic left heart syndrome
Incidence, anatomy, and natural history
Pathophysiology of HLHS
Surgical and transcatheter approaches and outcomes
Stage I palliation
Systemic‐to‐pulmonary and RV‐to‐pulmonary shunts
Hybrid procedure
Biventricular repair in borderline HLHS
Anesthetic considerations
Preoperative considerations
Mitral stenosis/aortic atresia
Hypoplastic ascending aorta (<2 mm)
Restrictive atrial septum
Obstructed pulmonary venous return
Greater than moderate tricuspid valve regurgitation
Pulmonary valve regurgitation
Age >14 days
Pre‐maturity
Low birth weight (<2.5 kg)
Non‐cardiac congenital abnormalities
Metabolic acidosis
Chromosomal abnormalities
Inotropic support
Right ventricular dysfunction
Intraoperative considerations
Postoperative considerations
Tricuspid atresia
Incidence, anatomy, and natural history
Pathophysiology of TA
Surgical and transcatheter approaches and outcomes
Anesthetic considerations
Pulmonary artery banding
Major pathophysiologic features
Type
Major anatomic features
Incidence
Cyanosis
CHF
Decreased systemic perfusion
Neonatal surgical approach
Type I: Normally related great arteries
70–80%
Ia
Intact VS with pulmonary atresia
+++
−
−
mBTS
Ib
Small VSD with PS
++
−
−
None
Ic
Large VSD, no PS
+
++
−
PA band
Type II: Transposition of the great arteries
12–25%
IIa
VSD with PA
+++
−
Yes if VSD restrictive
mBTS
IIb
VSD with PS
++
−
Yes if VSD restrictive
None
IIc
VSD no PS
+
++
Yes if VSD restrictive
PA band
Type II: Transposition of the great arteries with restrictive VSD or subAS
++
+++
++
DKS; or subAS resection, +/− enlarge VSD; or palliative ASO, +/− enlarge VSD
Type III: Normally related or transposed great arteries with other complex cardiac lesions
3–6%
+ to +++
− to ++
Depends on associated anomalies
Depends on associated anomalies
Systemic‐PA shunt and ductal stenting
Damus‐Kaye‐Stansel operation and palliative ASO
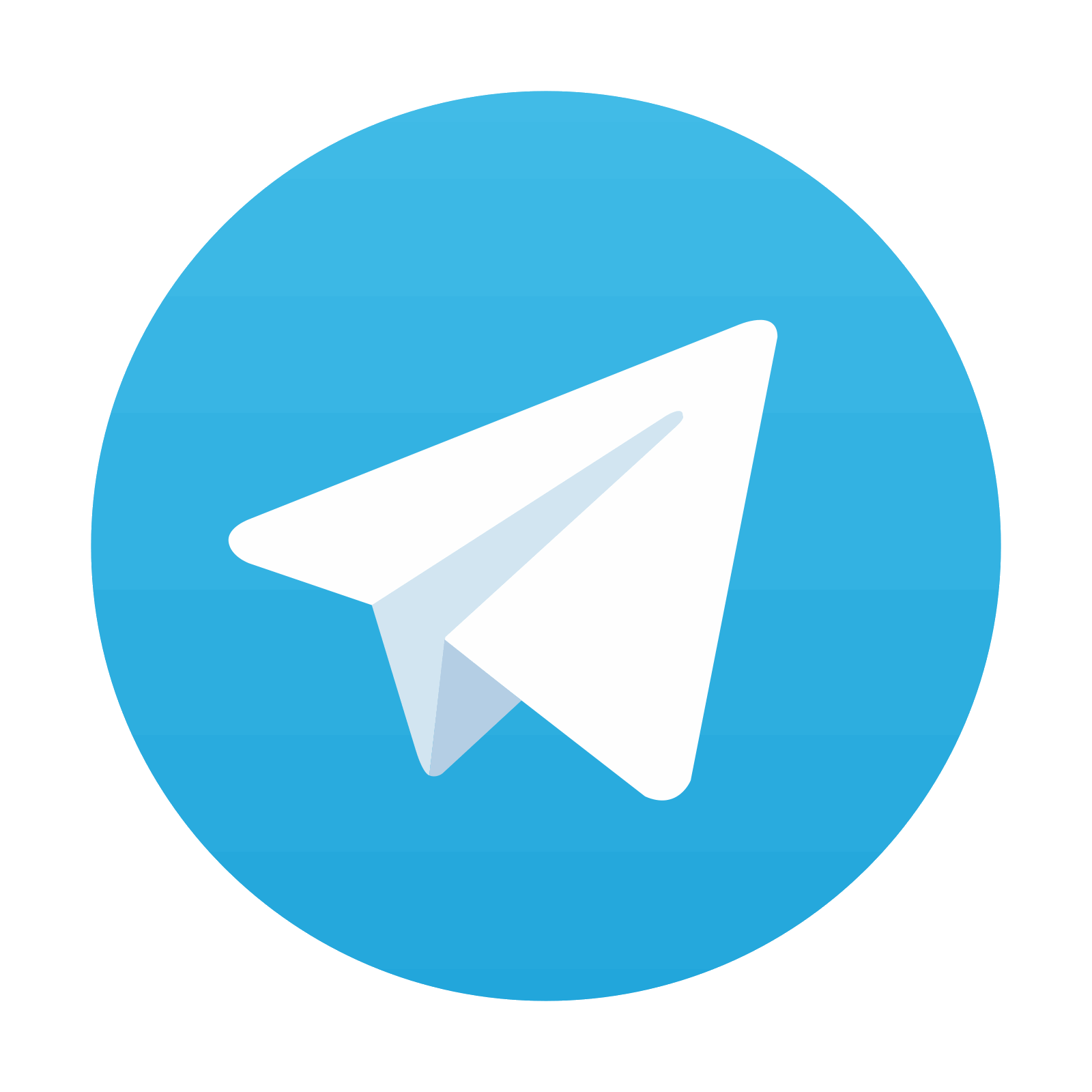
Stay updated, free articles. Join our Telegram channel
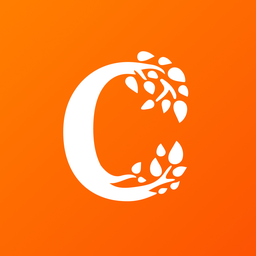
Full access? Get Clinical Tree
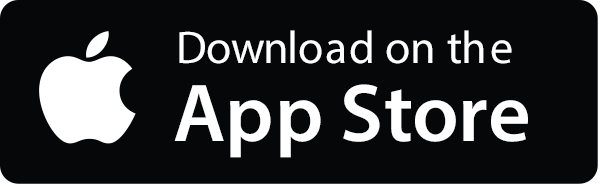
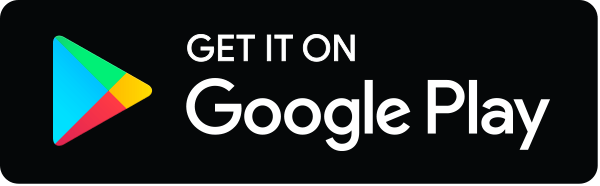