CHAPTER 29Anesthesia for Transposition of the Great Arteries
Valentine Woodham, Mariepi Manolis, Lucy Hepburn, and Angus McEwan
Department of Anaesthesia, Great Ormond Street Hospital for Children NHS Foundation Trust, London, UK
Introduction
Transposition of the great arteries (TGA) refers to a spectrum of conditions of discordant ventriculoarterial (VA) connections. The term TGA is most commonly used to denote concordant atrioventricular (AV) connection and discordant VA connection, where the aorta arises from the morphological right ventricle and the pulmonary artery from the morphological left ventricle. This is the most common anatomic form encountered in clinical practice and is referred to as dextro‐TGA, or d‐TGA. The term TGA also encompasses the hearts with discordant AV and VA connections known as congenitally corrected TGA (or ccTGA, levo‐ or l‐TGA) and more rarely, other forms of anomalous AV connections.
TGA is the second most common congenital cardiac lesion, which accounts for approximately 6% of all congenital heart defects [1]. It is frequently an isolated lesion with no other associated extracardiac anomalies. Medical and surgical advances in the past four decades have spectacularly improved the outcome for individuals born with these lesions, from what was a universally lethal condition, into one of expected survival with the ability to lead near‐normal lives.
Initial attempts at anatomical repair were associated with poor outcome, leading to the development of physiological repair by Mustard and Senning. Today, the arterial switch operation (ASO) is the mainstay of management of these patients, with excellent results. Surgical intervention requires the cardiac anesthesiologist to fully understand the pathophysiology, aims of treatment, surgical procedures, and postoperative consequences to form the perioperative management plan.
This chapter will address two main subcategories of transposition: physiologically uncorrected TGA (referred to as TGA or d‐TGA) and congenitally corrected TGA (ccTGA or l‐TGA).
Physiologically uncorrected TGA
Incidence
TGA accounts for 5–7% of all congenital heart defects with a prevalence of 0.2 per 1,000 live births [1, 2]. There is a male preponderance of 2–3:1, and sibling recurrence rates of between 0.27 and 1.8% have been noted [3].
Anatomy
Physiologically uncorrected transposition of the great arteries is the most common anatomic form encountered in clinical practice and is referred to as TGA or d‐TGA. In other words, the normal connections of right atrium (RA) to right ventricle (RV) and left atrium (LA) to left ventricle (LV) are preserved, but there is VA discordance: the aorta arises from the RV rather than LV and the pulmonary artery (PA) from the LV (Figure 29.1).
This definition encompasses a spectrum of cardiac anomalies that can be further subdivided into either simple or complex TGA (Figure 29.2). In approximately 50–75% of cases children present with simple TGA in which there is isolated VA (VA) discordance with either a patent foramen ovale (PFO) or patent ductus arteriosus (PDA) or both. This is referred to as TGA with intact ventricular septum (TGA/IVS).
The lesion is considered a complex TGA when it has associated cardiac abnormalities. Complex TGA occurs in 25% of the patients with D‐TGA and requires alternative management and operative planning. Cardiac anomalies included in complex TGA are as follows:
- Ventricular septal defect (VSD), 40%
- Left ventricular outflow tract obstruction (LVOTO), 5%
- Double outlet right ventricle and the Taussig–Bing malformation
- Rarer abnormalities, which include TGA with VSD and coarctation of the aorta 5% or TGA with VSD and interrupted aortic arch.
The focus of this chapter will be on the most common and important clinical subsets:
- TGA with intact ventricular septum (TGA/IVS)
- TGA with VSD including the Taussig–Bing malformation
- TGA with LVOTO
- TGA and pulmonary vascular obstructive disease
In the normal heart, the position of the aorta is posterior and to the right of the pulmonary trunk. There is a subpulmonary muscular infundibulum, and the annulus of the aortic valve is in fibrous continuity with the mitral valve annulus. In the most common manifestation of TGA, a right sided right atrium connects via a right sided tricuspid valve and RV to a right sided and anterior aorta. There is a subaortic muscular infundibulum. On the left, a left‐sided atrium connects via the mitral valve and LV to a left‐sided and posterior PA. As a result, there is fibrous continuity between the mitral and pulmonary valves, but no fibrous continuity between the tricuspid and aortic valves [4, 5]. This anatomical manifestation is commonly referred to as d‐ or dextro‐TGA with the position of the aorta being anterior and to the right of the pulmonary trunk. However, in strict anatomical terms, d‐ or dextro‐TGA describes patients who have [S,D,D] segmental anatomy, meaning situs solitus [S], d‐loop ventricles [D], and d‐loop great arteries [D].

Figure 29.1 Dextro‐transposition of the great arteries (d‐TGA). (A) Normal anatomy. (B) d‐TGA anatomy. Ao, aorta; PA, pulmonary artery; RA, right atrium; LA, left atrium; RV, right ventricle; LV, left ventricle; DAo, descending aorta.

Figure 29.2 The spectrum of discordant ventriculoarterial connections that come under the “umbrella” of the term transposition of the great arteries (TGA). AV, atrioventricular; VA, ventriculoarterial; PA, pulmonary artery; VSD, ventricular septal defect; IVS, intact ventricular septum; ccTGA, congenitally corrected transposition of the great arteries; LVOTO, left ventricular outflow tract obstruction.
Although VSDs are detected in up to 30–40% of patients with TGA using angiography, only about one‐third of these are hemodynamically significant [6]. Approximately one‐third of the VSDs are perimembranous, and another one‐third are outlet VSDs with malalignment. From a surgical perspective, outlet VSDs with malalignment are more difficult to repair. In the presence of a VSD, the aorta is generally smaller than the PA; this discrepancy in size tends to be amplified in the presence of an anterior maligned VSD. Conversely, LVOTO is common when there is posterior deviation of the outlet septum.
The Taussig–Bing anomaly (TBA) represents a complex subset of double outlet right ventricle (DORV) with subpulmonary VSD that behaves as a physiological TGA. It is not theoretically a TGA, as the VA connection is double outlet, however, it is so similar to TGA from a physiological and management perspective that it is included within the TGA subset. In TBA, there is DORV with subpulmonary VSD, side‐by‐side vessels, and bilateral conus [7]. The aorta originates from the RV, and the PA arises from above the nonrestrictive VSD. It is frequently associated with subaortic right ventricular outflow tract obstruction (RVOTO) because of hypertrophy of the infundibular septum and aortic arch obstruction. Left ventricular oxygenated blood is preferentially streamed into the PA, thereby simulating TGA physiology.
Anatomic LVOTO can be found in approximately one‐third of patients with TGA and VSD, but it is rare in TGA/IVS, with an incidence of approximately 0.7%. Anatomic LVOTO can arise at the valvular or subvalvular level and can be caused by several anatomical structures. Valvular obstruction might be secondary to commissural fusion, annular hypoplasia, or leaflet immobility from a hypertrophic tissue [6, 8]. Pulmonary subvalvular obstruction may be attributable to posterior displacement of the conal septum, a subvalvular fibrous membrane, accessory leaflet tissue of the AV valve, or diffuse hypoplasia of the left ventricular outflow tract (LVOT). In TGA/IVS, mechanical LVOTO is uncommon, but 5% patients have functional LVOTO. This occurs due to the presence of a high systemic right ventricular pressure, displacing the interventricular septum into the LV during systole. This is uncommon in neonates as the septal shift required is prevented by their high pulmonary vascular resistance [9]. LVOTO limits pulmonary blood flow and therefore exacerbates the cyanosis in TGA.
Coronary artery anatomy
Coronary artery anatomy is extremely important in d‐TGA patients as transfer of the coronary arteries to the neo‐aorta without narrowing or distortion is critical to the success of an arterial switch operation (ASO). Mortality from distortion of the newly implanted coronary arteries arises from myocardial ischemia; necrosis of the supplied myocardial segment may cause fatal arrythmias, such as ventricular fibrillation [10]. The critical importance of unrestricted aortocoronary blood flow after ASO was established during the earliest unsuccessful ASOs in the 1950s by William T. Mustard [11, 12]. Initially the ASO was associated with 100% mortality. In 1954, three patients underwent ASO, a 6‐week‐old child died from aortic suture line bleeding, a 19‐day‐old died from left coronary artery occlusion, and another 19‐day‐old died from ventricular fibrillation [13].
In the normal heart, the coronary arteries arise from the aortic sinuses of Valsalva, which face the PA and are located on the anterior portion of the aorta (Figure 29.3). In TGA, the coronary arteries also arise from the aortic sinuses of Valsalva, but the location of the sinuses is posterior [4]. Considerable variability exists in the origin, distribution, and epicardial course of coronary artery anatomy in d‐TGA. The Leiden classification (Figure 29.4) system appears most commonly in surgical literature and is used to demonstrate the frequent coronary patterns that exist in TGA [16].
Although all types of coronary anatomy are suitable for the ASO, the less common coronary patterns present potential technical difficulties for an ASO and a moderately increased risk [17]. Single right coronary artery, inverted coronary artery, and coronary arteries with an intramural course have been identified as carrying increased risk [17, 18], but recent studies have shown that with increased surgical experience, coronary artery pattern may not be a risk factor for increased morbidity following the ASO [19, 20].
Natural history and pathophysiology
In the normal circulation, blood circulates in series; deoxygenated blood from the systemic circulation enters the right heart and proceeds to the lungs via the PA. Oxygenated blood then returns from the lungs via the pulmonary veins to the LA and is pumped through the LV to the systemic circulation via the aorta. In TGA, there is AV concordance (RA→RV and LA→LV) but VA discordance (LV→PA and RV→ aorta) resulting in two parallel circulations (Figure 29.5). Blood flows in a closed circuit on both sides of the heart where deoxygenated blood is recirculated to the body without passing the lungs for oxygenation. Oxygenated blood from the left side of the heart returns back to the lungs via the PA without reaching the body. Without one or more communications that allow for intercirculatory mixing, survival would be impossible. If left untreated, death from hypoxia and congestive heart failure (CHF) will occur in 90% of patients within the first year of life.

Figure 29.3 Anatomy of normal coronary arteries is well shown by computed tomography. (A) The aortic root as viewed from above. Right and left coronary arteries arise from the aortic valvular sinuses adjacent to the subpulmonary infundibulum, which has been transected to provide this image. (B) Variant in an otherwise normal heart in which the circumflex coronary artery arises from the right coronary artery and then extends through the transverse sinus.
(Source: Chiu & Anderson [14]. Reproduced with permission of Elsevier.)
Shunting and effective pulmonary and systemic blood flow in TGA
In TGA, the parallel circulations mean that, without shunting, no oxygenated blood would reach the tissues or deoxygenated blood the lungs. Anatomical shunting occurs due to a communication at the level of the cardiac chambers or great vessels allowing blood to move from one circulation to the other. A physiological shunt occurs when blood is recirculated and is considered “surplus” and noneffective. For example, a physiological left to right shunt is all the blood that is recirculated through the pulmonary vascular bed (the LA to the PA avoiding the systemic circulation). Physiological right‐to‐left shunt occurs through the recirculation of systemic venous blood (RA to the aorta, avoiding the pulmonary circulation). Therefore, in TGA, the majority of the blood flow is physiological shunt (recirculated blood that is ineffective) with a small proportion of blood that travels across the anatomical shunt (in effect, making up the effective blood flow) (Figure 29.6) [21]. The effective blood flow is the flow necessary for survival and comprises blood from the venous system of one circulation that reaches the arterial system of the other.
- Effective pulmonary blood flow is the amount of systemic venous blood that reaches the pulmonary arterial bed (e.g., in TGA, an anatomic R‐to‐L shunt)
- Effective systemic blood flow is the amount of pulmonary venous blood that reaches the arterial systemic circulation (e.g., in TGA, an anatomic L‐to‐R shunt)
Note: When bidirectional shunting occurs, the anatomic and effective blood flows are not equal. This is because some of the anatomic shunted blood may be redirected back to its original venous side prior to reach the arterial side of the other circulation. [21]
Effective pulmonary blood flow and effective systemic blood flow must be equal or one of the circulations would become deplete. Total pulmonary circulation is the volume of recirculated pulmonary venous blood and effective pulmonary blood flow. Total systemic blood flow is the volume of recirculated systemic blood and effective systemic blood flow. The greater the volume of the effective systemic blood flow relative to the recirculated flow, the greater is the arterial oxygen saturation. In contrast to effective blood flow, total pulmonary blood and total systemic blood flow do not have to be equal. This is most evident in the pulmonary circulation, where the total pulmonary blood flow is 2 to 3 times that of the total systemic blood flow (Qp:Qs 2–3:1). When there is adequate mixing of pulmonary and systemic venous blood, the arterial oxygen saturation is influenced by the Qp:Qs ratio. A high Qp will result in a pulmonary artery saturation that is higher than the aortic oxygen saturation, and systemic hypoxia will not occur. If there is effective mixing but pulmonary blood flow is restricted (due to LVOTO or PAH), the Qp:Qs ratio will drop below 2, significantly lowering the systemic arterial saturation and causing severe hypoxia [22]. These concepts are vital in understanding the physiology in TGA circulation.

Figure 29.4 The most common patterns of coronary anatomy in patients with D‐loop transposition of the great arteries (TGA). (A) Usual coronary anatomy with the left coronary artery (LCA) arising from sinus 1 and the right coronary artery (RCA) from sinus 2. (B) Left circumflex coronary artery (LCx) arising from the RCA (sinus 2) and the left anterior descending (LAD) from sinus 1. (C) Single coronary from sinus 2. (D) Single coronary artery arising from sinus 1. (E) Inverted origin with the RCA arising from sinus 1 and the LCA from sinus 2. (F) The LAD and RCA arising from sinus 1 and the LCx from sinus 2. (G) Intramural LCA with origin of the LCA and RCA both from sinus 2 and intramural course of the LCA. (H) Intramural RCA with origin of the RCA and LCA from sinus 1 and intramural course of the RCA.
(Source: Mertens et al. [15]. Reproduced with permission of John Wiley & Sons.)
Intercirculatory mixing
Intercirculatory mixing can occur at one or more intracardiac (PFO/ASD or VSD) or extra‐cardiac (PDA or bronchopulmonary collaterals) sites. The degree of intercirculatory mixing is one of the factors that influences hemoglobin oxygen saturation and hence tissue oxygen delivery. In the first few days of life, the degree of cyanosis and the stability of the child will be largely determined based on the degree of mixing that occurs between the two parallel circulations, which in turn will determine the neonate’s course, medical management, and surgical timing.
Intercirculatory mixing is dependent on multiple factors in the neonatal period, namely the number, size, and position of the anatomic communications (Table 29.1). Larger, unrestrictive, and multiple communications allow for improved intercirculatory mixing. Although there is significant interplay between these factors, one large communication will allow for better mixing than two or more restrictive ones and a large but unfavorably positioned VSD, namely an anterior muscular VSD, is not conducive to effective mixing. [23]
Physiological factors have a significant impact on mixing, including ventricular compliance, systemic vascular resistance (SVR), and pulmonary vascular resistance (PVR). Balancing the effective pulmonary and systemic blood flows allows for optimal intercirculatory mixing. At the level of the atria, shunting is mainly influenced by the size of the ASD and the pressure variations across it. Mixing here is the most efficient as lower pressures allow for bidirectional flow during the cardiac cycle; for this physiologic reason, balloon atrial septostomy (BAS – see below) is preferred for cyanotic d‐TGA patients. Conversely, mixing at a VSD or PDA is usually more restricted as larger pressure gradients exist, typically directing blood in one direction (usually from right to left).

Figure 29.5 Comparison of the normal circulation, which is in series, with that in transposition of the great arteries (TGA), which is in parallel. In the normal circulation (left), deoxygenated blood from the body enters the right‐sided circulation before being oxygenated by the lungs and then distributed to the body via the left side of the heart. In the transposition circulation (right), the potential sites of mixing are shown: atrial septal defect (ASD), ventricular septal defect (VSD), and patent ductus arteriosus (PDA). The circulation is in parallel; thus, deoxygenated blood from the body enters the right side of the heart, but because of the anomalous connection, it is recirculated to the body via the aorta. Similarly, the oxygenated blood from the lungs enters the left heart but is recirculated to the lungs via the PA. This condition would be incompatible with life, if there was not any mixing of the oxygenated and deoxygenated blood. RA, right atrium; RV, right ventricle; PA, pulmonary artery.

Figure 29.6 Representation of intracardiac shunting in transposition of the great arteries with intact ventricular septum (TGA/IVS), unrestrictive atrial septum, and PDA. The majority of flow is recirculated, RA→aorta and LA→PA, and is therefore, ineffective. A small amount of blood shunts left‐to‐right via the ASD; this is effective systemic blood flow. Equally, a small amount of blood flows from the aorta to the PA via the PDA, this is effective pulmonary blood flow. The effective systemic and pulmonary blood flows are equal and constitute a small volume of flow that is necessary for life. Typically, the total pulmonary blood flow is 2 to 3 times greater than the total systemic blood flow. AO, aorta; PA, pulmonary artery; ASD, atrial septal defect; LA, left atrium; RA, right atrium; RV, right ventricle; LV, left ventricle; PDA, patent ductus arteriosus.
Table 29.1 Factors influencing intercirculatory mixing in patients with D‐TGA
Factor | Effect on mixing |
---|---|
Size |
|
Position |
|
Number |
|
Pulmonary blood flow |
|
Pulmonary blood flow
The efficiency of mixing is further influenced by total pulmonary blood flow (Qp) [23]. As the patient surpasses the neonatal period, pulmonary blood flow is increasingly important in determining intercirculatory mixing. As PVR continues to drop after the neonatal period, cyanosis tends to improve. This may occur as the decrease in PVR increases pulmonary blood flow, thus increasing mixing. The opposite may also to be true: as pulmonary blood flow is reduced (usually secondary to pulmonary stenosis or pulmonary vascular occlusive disease), a decrease in mixing occurs resulting in a drop in systemic arterial saturation [23, 24]. A reduction in pulmonary blood flow caused by a decrease in overall cardiac output (e.g., hypovolemia, depressed myocardial contractility from volatile anesthetics) will also exacerbate cyanosis.
TGA and pulmonary vascular occlusive disease
Children with TGA are at particular risk of developing pulmonary vascular occlusive disease compared with other congenital heart lesions, especially in TGA with VSD. Pulmonary vascular remodeling caused by an abnormal hemodynamic state [25] (i.e., high pulmonary blood flow) plays a significant role in the development of pulmonary vascular occlusive disease. Multiple other factors believed to contribute to this disease process also exist in these patients: hypoxemia, polycythemia, and high PA pressure [6].
As PVR increases and pulmonary hypertension develops, pulmonary blood flow is reduced, thereby decreasing intercirculatory mixing and worsening systemic hypoxemia. Late diagnosis of TGA may result in severe pulmonary vascular occlusive disease. Once pulmonary hypertension is established, the therapeutic surgical options are reduced, sometimes necessitating palliative rather than curative options.
Flow characteristics and intercirculatory mixing vary depending on the variations in anatomy of the TGA subsets.
TGA with intact ventricular septum
In TGA/IVS, intercirculatory mixing occurs at two main sites: ASD and PDA. In TGA/IVS without additional communications (i.e., no PDA), shunting at the atrium is predominantly dependent on the size and pressure gradients across the atrial communication. During systole blood shunts from the LA to the RA (effective systemic blood flow) due to a raised LAP from the high volume of recirculating pulmonary blood flow. During diastole blood shunts from the RA to the LA (effective pulmonary blood flow) due to reduced ventricular and vascular compliance of the systemic circuit [26]. Respiratory variation also effects shunting across the atrial communication.
When a PDA is also present, flow across the PDA is dependent on the size of the inter‐atrial communication and the PVR. With a non‐restrictive ASD and a low PVR, blood flows from the aorta to the PA across the PDA (effective pulmonary blood flow) and left to right across the ASD (effective systemic blood flow).
As the PVR increases, shunting across the PDA and ASD becomes bidirectional. A unique clinical scenario occurs when raised PVR results in the PAP exceeding aortic pressure, causing blood to shunt across the PDA from the PA to the descending aorta, creating a reverse differential cyanosis. In this scenario, post ductal saturations (lower limb) will be higher than preductal (right hand). This phenomenon only occurs in TGA with preductal coarctation/interruption or when PVR is elevated (usually due to persistent pulmonary hypertension). A restrictive ASD or small PFO can cause elevated PVR due to raised LAP, resulting in poor mixing and severe cyanosis. The PFO may even close due to the raised LAP – eliminating a source of atrial mixing. Smaller infants with inadequate atrial mixing are at a high risk of preoperative or sudden death at birth due to PFO closure [27]. A BAS may be lifesaving in this setting.
TGA with ventricular septal defect
Although VSDs occur in 40% of patients with TGA, approximately one‐third of these are too small and not functionally significant [28]. In TGA with non‐restrictive VSDs, mixing at the VSD is usually less efficient than at the atrial level. This is due to larger pressure gradients throughout the cardiac cycle at the level of the ventricles which typically shunt blood in one direction, in this case from right to left (effective pulmonary blood flow). Flow across the PDA is also predominantly effective pulmonary blood flow, with blood shunting from the RV to the aorta to the PA. Effective systemic blood flow originates from blood shunted from left to right across an ASD or PFO. The increased pulmonary blood flow will pass almost entirely through the LV, resulting in a volume‐overloaded LV and subsequent heart failure. This increased pulmonary blood flow, particularly in patients with a VSD, significantly increases the risk of developing PAH, as systemic pressures are transmitted to the pulmonary vasculature.
TGA with left ventricular outflow tract obstruction
TGA with LVOTO occurs in approximately 5–10% of patients with TGA and is more commonly associated with a VSD. In TGA with LVOTO and VSD, there is a large anatomical left to right shunt and reduced pulmonary blood flow. Effective pulmonary blood flow is carried via the PDA. Due to a low Qp, LA hypertension and cardiac failure do not occur, but severe systemic hypoxemia is present. Cyanosis after birth is a common clinical feature, the degree of which is dependent on the magnitude of the obstruction.
Dynamic LVOTO can occur in patients with TGA/IVS who do not undergo an early ASO. This happens when the LV pressure drops below the RV pressure in the postnatal period due to a corresponding drop in PVR. High systemic right ventricular pressure displaces the interventricular septum posteriorly into the left ventricular outflow tract during systole. Dynamic LVOTO should be resolved after ASO; however, if left untreated, this obstruction can become fixed over time necessitating alternative surgical strategies.
Fixed LVOTO requires detailed surgical planning with assessment of the degree of LVOTO and adequacy of the pulmonary valve. Selected patients with fixed LVOTO may still proceed to ASO with satisfactory short‐ and mid‐term results [29, 30]. Neo‐aortic valve regurgitation remains a concern in long term [29]. If LVOTO is severe and fixed, other surgical options than ASO are advised [29, 30].
TGA with right ventricular outflow tract obstruction
Obstruction to the systemic circulation is an infrequent but important variant of TGA and occurs in approximately 5–10% of cases [31]. Systemic saturations will be variable depending on the degree of RVOTO and significant systemic hypoperfusion may occur due to impedance of systemic blood flow (including absent or diminished femoral pulses) if the PDA becomes restrictive. As mentioned above, a finding of reverse differential cyanosis indicates significant anatomical left‐to‐right shunting at the PDA and should alert clinical suspicion of coarctation of the aorta. A detailed evaluation of the aortic arch anatomy and of any coarctation and/or aortic arch hypoplasia are important surgical considerations.
Clinical presentation and diagnostic features
With the evolution of fetal echocardiography, most diagnoses of TGA are likely to occur antenatally. As a result, a preliminary management plan can be formed aimed at postnatal stabilization before a more definitive surgical correction takes place. Antenatal diagnosis facilitates planned delivery and early administration of prostaglandin preventing the production of lactate and thus improving the neonates’ pre‐operative condition. In addition, it reduces the risk of cerebral damage which has been shown to correlate with high lactates [32]. A detailed echocardiogram is repeated at birth which allows for comprehensive assessment of cardiac anatomy and the associated anatomical defects and to aid preoperative management and surgical planning. The echo must be performed promptly as prolonged duration of severe cyanosis and tissue hypoxia are important factors in determining acidosis, ventricular function, and ultimately multiple organ failure.
Clinical features in TGA are difficult to distinguish from other causes of neonatal cyanosis:
- Cyanosis‐The degree of cyanosis is determined by the amount of intercirculatory mixing. In infants with mild cyanosis, the lesion may only be detected on the basis of a pulse oximetry screen.
TGA/IVS: If the ASD is restrictive, limited intercirculatory mixing occurs leading to profound central cyanosis and tachypnea presenting within the first few hours of life. Arterial oxygen saturation will usually range between 50 and 70% unresponsive to oxygen administration.
TGA LVOTO: Due to reduced pulmonary blood flow from LVOTO or pulmonary obstructive disease, these patients can present with significant cyanosis. Reverse differential cyanosis might be present.
TGA/VSD: In infants with a large VSD that allows for satisfactory mixing between the two circulations, cyanosis may only be perceived during episodes of crying or agitation. However, signs of congestive heart failure will prevail due to ventricular overload: tachypnea, tachycardia, failure to thrive, a gallop rhythm, and eventually hepatomegaly. Arterial oxygen saturations will range between 75 and 90%.
Table 29.2 ECG and Chest Radiograph (CXR) findings in various D‐TGA morphologies
TGA morphology ECG CXR TGA IVS Essentially normal “Egg on side” TGA VSD Normal or RVH Narrow superior mediastinal area, cardiomegaly, prominent vascular markings TGA LVOTO Normal or biventricular hypertrophy Cardiomegaly, increased pulmonary vascular markings - Tachypnea – Patients will usually have a respiratory rate of over 60 breaths per minute and appear comfortable without other signs of respiratory distress (grunting, nasal flaring, subcoastal, or tracheal recession). Infants with large VSDs may present with severe respiratory distress due to heart failure by three to 4 weeks of age.
- Heart murmurs – Heart murmurs are not a consistent finding in patients with TGA. An ejection systolic murmur is associated with left outflow tract obstruction (i.e., PS). A pansystolic murmur may be heard with a small to moderate VSD or PDA.
- Diminished pulses – Diminished femoral pulses may be seen in patients who also have coarctation of the aorta or interruption of the aortic arch.
Cardiac catheterization is not routinely performed unless it is in conjunction with BAS, when ECHO cannot establish coronary artery anatomy, or to quantify pulmonary vascular occlusive disease and PVR in the older child. Table 29.2 presents common ECG and chest radiograph findings in the various TGA morphologies.
Preoperative management
After birth, infants with TGA require early treatment; it is therefore recommended that they are delivered at or close to a tertiary care hospital that provides pediatric cardiology and cardiac surgery facilities [33]. Enabling early stabilization and optimization of these children can reduce cost and transport‐related complications [34]. Infants born outside of a tertiary center will need to be stabilized in NICU prior to transfer; some institutions will electively intubate these patients, although there is increasing evidence that rates of complications are higher in intubated neonates [35].
The initial management of newborns with TGA should focus on stabilization, optimization of the mixing of systemic and pulmonary circulations, oxygen delivery, maintenance of adequate systemic perfusion and correction of acidosis. To ensure sufficient mixing between the two parallel circulations, an intravenous infusion of prostaglandin E1 is recommended to maintain patency of the PDA until a comprehensive series of post‐natal echocardiograms has been completed to assess all sites of possible intercirculatory mixing. Prostaglandin infusions should be started at 0.05 mcg/kg/min, higher doses may be necessary to re‐open a closed PDA. Prostaglandin may cause fever, platelet dysfunction, hypotension and apnea, particularly with increased doses which may necessitate intubation. If PGE1 does not improve tissue oxygenation, then an urgent balloon atrial septostomy (BAS) should be performed as ductal shunting is often insufficient in the presence of a restrictive ASD.
Transcatheter approaches
A BAS can be performed in a catheterization lab under angiography or in NICU using echocardiography. Occasionally, BAS may be performed at the local hospital by a specialist cardiac retrieval team so that the patient can be stabilized prior to transport to the cardiac center for definitive surgery. Because BAS can be safely performed under echocardiographic guidance, preoperative diagnostic cardiac catheterization should be considered only in selected cases for diagnosis of complex lesions or if institutional experience does not permit performance of atrial septostomy under echocardiographic guidance. Exact coronary artery definition is generally not required by the majority of surgeons as it can be determined in the operating room, and ASO is performed for almost every coronary artery arrangement.
During BAS, a balloon is passed via the umbilical vein or the femoral vein, into the left atrium across the PFO, the balloon is inflated and pulled back briskly across the septum, to tear the inferior aspect of the foramen ovale (Figure 29.7). This is repeated at least once to ensure adequate enlargement of the ASD; echocardiogram is performed to assess the intercirculatory mixing.
Outcomes from balloon atrial septostomy
The introduction of balloon atrial septostomy (BAS) by Rashkind and Miller in 1966 has greatly improved the prognosis of patients with TGA, with 3‐month survival increasing from 20 to 85% [37]. The significant improvement in saturations must be weighed against the risk of vascular trauma, arrhythmias, and rare complications such as atrial perforation and avulsion of the inferior vena cava [38]. Mortality of an urgent BAS is around 3%, with increased risk of complications if performed out of hours [39]. Although there is no increased mortality between patients who received a BAS and patients who did not, some studies have shown a strong association between BAS and preoperative stroke in neonates with TGA [39, 40]. Multiple studies have described MRI changes in both white and grey matter locations, including focal ischemic changes and periventricular leukomalacia (PVL) in patients with TGA, with reported prevalence rates of 30% [41]. Although it is possible that this is due to embolic phenomenon from the BAS, there are likely to be multifactorial components, including the degree of hypoxemia and hemodynamic instability. Multiple papers have now refuted the association between BAS and stroke [42, 43].

Figure 29.7 Balloon atrial septostomy. (A) Schematic representation of the maneuver. A balloon‐tipped catheter is introduced via the inferior vena cava into the right atrium. After passing through the foramen ovale, the balloon is inflated into the left atrium and then pulled across the septum to enlarge the opening. Finally, the balloon is rapidly deflated, and the catheter removed. (B) Angiographic images showing the different steps of the maneuver.
(Source: Seguela et al. [36]. Reproduced with permission of Elsevier.)
Anesthesia for balloon atrial septostomy
A BAS can be performed in the cardiac catheterization laboratory under angiographic guidance or in the neonatal intensive care unit under echo guidance, with the latter being more common.
The procedure is performed by a pediatric interventional cardiologist, with a neonatologist or an anesthetist present to manage sedation and mechanical ventilation.
A stable airway must be guaranteed, although not all neonates need to be intubated. Our center advocates routine intubation, this permits optimal sedation and analgesia, as well as permitting the use of paralysis to facilitate BAS. Prior to the procedure, an ABG, baseline blood tests, and IV access are required. A unit of red blood cells is essential and ideally crossmatched if time permits. Baseline observations including HR, RR, pre‐ and post‐ductal saturations, BP, blood glucose, and urine output should be documented prior to and throughout the procedure.
Intubated neonates will usually be sedated with a morphine infusion, with additional boluses of fentanyl, ketamine or midazolam given as required. In spontaneous ventilating neonates, small incremental boluses of appropriate sedation should be used, including opioids (morphine or fentanyl), midazolam, and ketamine [44]. Dexmedetomidine is a safe drug in children with congenital heart disease with no significant respiratory depressive effect; thus, it may be useful in this setting [45]. Acetaminophen can be a useful adjunct in these patients.
If a BAS is performed successfully, systemic oxygen saturations should begin to increase immediately; if adequate mixing is seen at the atrial level, then the PGE1 infusion can be gradually discontinued (as long as there are no concerns regarding aortic arch obstruction). The aim is now to ensure hemodynamic stability and optimize preoperative condition. Once the patient is stable, they can be fed, and all further required investigations should be performed.
Associated medical problems (such as respiratory distress, necrotizing enterocolitis (NEC), and intracranial hemorrhage) should be diagnosed and treated appropriately. If the patient is extremely premature or other associated conditions are present, an early ASO should be postponed. If intracranial hemorrhage occurs, surgical repair is usually delayed by 2 weeks. Due to this delay, the left ventricular mass will decrease and will need to be “re‐trained” to support a high SVR after the ASO (see below).
A small proportion of patients may remain cyanotic after a BAS, requiring further detailed echocardiography to confirm the ASD is unrestrictive, assess the patency of the PDA, and assess for pulmonary hypertension if present. Incidence of persistent pulmonary hypertension in neonates with TGA is 12.5%, occurring more commonly in patients with TGA /IVS [46]. Multiple treatment strategies have been used with variable success, including sedation, paralysis and hyperventilation, inhaled nitric oxide (NO) [47], sildenafil, bosentan [48], and extracorporeal life support (ECLS) [49]. The prognosis in these patients remains poor [50].
Surgical options
The ASO, first performed successfully in 1975 by Adib Jatene, has become the standard corrective procedure for patients with D‐TGA and has revolutionized their survival (Figure 29.8). Mortality has decreased from >90% to <1% in patients with simple TGA and 4% with complex TGA [51]. The ASO has been used as the preferred procedure for patients with D‐TGA since the late 1980s and has replaced the earlier atrial switch techniques by Mustard and Senning.
Indication and timing of ASO
Significant uncertainty exists regarding the optimal timing of surgical repair. For ASO to be successful, the LV must have significant myocardial mass to support the systemic circulation. At birth, the LV mass is equal to that of the RV. In TGA/IVS, as the PVR drops postnatally the left ventricle involutes, losing muscle mass and thus becoming unable to support the systemic circulation [52]. Due to this, the majority of ASOs for TGA/IVS are performed within the first 2 weeks of life [53]. In older infants with TGA/IVS, there is a concern that the LV will decondition and therefore fail when exposed to higher systemic pressures after ASO, resulting in low cardiac output syndrome. There is no clear upper age limit for ASO, with most surgeons performing a primary ASO up to 4 weeks of age (Box 29.1). Beyond 4 weeks, a primary ASO becomes controversial, and it is likely that patients will require post‐op mechanical circulatory support and a prolonged ICU admission [54]. A study from Lo Rito et al, however, has suggested that primary ASO can be safely performed in TGA/IVS patients who present late with a severely deconditioned LV. They showed no significant differences in early mortality or LV recovery, although an increase in neurological injury was noted, which was thought to be due to the 3‐ to 5‐fold increase in ECMO use [55]. For infants >2 months old, a rapid two stage ASO is recommended. A two‐stage ASO involves pulmonary artery banding (+/− an aortopulmonary shunt in cyanotic patients) to “re‐train” the left ventricle by inducing LV hypertrophy before performing an ASO within 2 weeks. The PA band must be sufficiently tight to increase pressure in the LV to approximately one‐half to two‐thirds of the systemic ventricle without compromising pulmonary blood flow.

Figure 29.8 The arterial switch operation (ASO). Schematic representation of d‐transposition of the great arteries anatomy before (A) and after (B) the ASO. Ao, aorta; PA, pulmonary artery; LA, left atrium; RA, right atrium; RV, right ventricle; LV, left ventricle.
The majority of the left ventricular mass increase occurs in the first seven days after banding, with the ASO therefore typically performed within a week [57]. Although primary ASOs have better outcomes, a rapid two‐stage repair may be unavoidable in patients where a primary ASO would expose the infant to excessive risk. Primary ASO may not be possible due to prematurity, sepsis (e.g., NEC), low birth weight (<1.5 kg) or late presentation.
Timing of surgery may also have an impact on neurodevelopmental outcome with some evidence to suggest that delaying surgery beyond 2 weeks of age is associated with impaired brain growth and slower language development at 18 months of age [58]. This may be due to the effects of prolonged hypoxia on the developing brain, with the risk of PVL increasing with lower arterial oxygenation and longer time to surgical repair [43].
Some evidence supports day 3 of life as the optimal time for an ASO, with increasing morbidity and healthcare cost beyond this timeframe. Currently, in Europe, 30% of ASOs are performed within the first week of life [59], with the majority of ASOs performed on Day 6 or 7 in the United States [60]. Preliminary reports have shown that performing an ASO in the first few hours of life is safe, thus avoiding the need for a BAS and theoretically reducing neurological impacts of prolonged hypoxia. This technique remains very controversial having been carried out in a limited number of cases [61, 62].
In the subsets of patients where the LV is exposed to systemic pressures (such as LVOTO, a large VSD, or PDA), LV mass is maintained after birth, preventing ventricular deconditioning beyond the age of 2–3 weeks. In these patients, there is less urgency to perform the surgery, and it can be done up to 6 weeks of life. In reality, they are usually operated on within the first month, before intractable cardiac failure or pulmonary vascular occlusive disease can occur.
TGA with left ventricular outflow tract obstruction
Surgical intervention in TGA with LVOTO varies with the type of LVOTO obstruction. Dynamic LVOTO obstruction is amenable to ASO as the dynamic nature of the LVOTO will be fully reversed, with no residual LVOT gradient.
Challenging decision‐making is required in TGA with mechanical LVOT obstruction. The surgical decision is dependent on the complexity of LVOT obstruction, the size of the pulmonary valve, and the size of the VSD (Figure 29.9). ASO and muscle resection may be possible in certain patients with a normally aligned LVOT where complete or near complete relief of the LVOTO is to be expected. There is an increased risk of reintervention of the LVOT or neo‐aortic valve [30]. For complex TGA with severe or fixed LVOTO (due to subvalvular, valvular or multilevel obstruction) and a VSD, an ASO is generally not performed. There are three main surgical options: the Rastelli, the REV and the Nikaidoh procedure (see below).
Aortic arch obstruction (coarctation with hypoplastic arch or interruption) is present in only 5–9% of TGA patients, but it is much more frequent in patients with TBA (more than 50%). Previously a two‐stage repair was advocated in these patients that included a primary coarctation reconstruction +/− PA band, followed by an ASO or intracardiac repair months later; however, mortality rates were high. Now a one‐stage ASO is usually undertaken [63].
Arterial switch operation
The ASO is performed through a median sternotomy on cardiopulmonary bypass (CPB). Both of the great vessels are transected distal to their respective valves, translocated, and re‐attached to the opposite root. This will result in the right ventricle attaching to the pulmonary artery and the left ventricle to the aorta. When CPB is established, the ductus arteriosus is divided, the ascending aorta is clamped, and cardioplegia is administered. The ascending aorta is transected above the commissures and the left and right coronary arteries are excised with 3–4 mm of the surrounding tissue. These aortic explant sites are repaired with a pericardial patch. The main pulmonary artery is transected, and the coronary arteries are reimplanted into the remaining root of the PA which will become the neo‐aorta (Figure 29.10). This clarifies why delineating coronary artery anatomy is important prior to surgical repair. The LeCompte maneuver (Figure 29.11) is then performed; this involves the PA being brought anterior to the aorta before it is re‐anastomosed to the native aortic root whilst the distal aorta is re‐anastomosed to the proximal cuff of the native pulmonary artery (now the neo‐aorta). This results in the aorta lying behind the bifurcation of the PA, which reduces the risk of subsequent PA stenosis.

Figure 29.9 Flowchart illustrating anatomical factors determining surgical decision‐making in patients with transposition of the great arteries (TGA) with left ventricular outflow tract obstruction (LVOTO). VSD, ventricular septal defect; IVS, intraventricular septum; ASO, arterial switch operation; LV‐PA conduit, left ventricle to pulmonary artery conduit; REV, Réparation à l’Etage Ventriculaire.
An ASD is usually closed by direct suture, while patients with a VSD require a pericardial patch. In children with TBA, the VSD is closed with a patch placed diagonally to achieve continuity of the LV with the neo‐aorta, while the neo‐PA remains connected to the RV.
Once the aortic cross‐clamp is removed, the myocardium is carefully observed for perfusion, and ECG and LAP are closely monitored. Ischemia of the myocardium is reflected by lack of reactive hyperemia or a rise in LAP, even before the ECG changes become apparent. A rise of LAP above 10 mmHg requires careful re‐examination of the myocardium and coronary vessels to ensure adequate coronary flow. During rewarming, the aortic sinus defect is repaired, the anastomosis is completed, and temporary atrial and ventricular pacing wires are placed (Figure 29.12).
Other surgical procedures
Rastelli procedure
The Rastelli procedure is used to anatomically correct TGA with VSD and fixed LVOTO. A right ventriculotomy is performed, and a patch is placed to diagonally direct blood from the LV through the VSD toward the aorta. The PA is ligated at the level of the pulmonary valve, and a valved RV‐to‐PA conduit is placed [65]. The conduit can be an aortic or pulmonary homograft with a polytetrafluoroethylene graft extension. This achieves the LV to the aorta and the RV to the PA continuity, whilst bypassing the LVOTO (Figure 29.13). It is advantageous as it allows the LV to act as the systemic ventricle. The Rastelli procedure requires a large subaortic VSD, although restrictive VSDs can be enlarged to allow precise patch placement. Enlargement of the VSD has been shown to reduce the risk of developing post‐operative LVOTO but may increase the risk of heart block from damage to the conduction pathway [66].
In patients with severe LVOTO, where the pulmonary blood flow is low and inter‐arterial mixing is limited, a systemic‐to‐pulmonary shunt (usually a modified Blalock– Taussig shunt) is inserted in the neonatal period; these children are too young to be considered for a primary Rastelli procedure. This is used to increase pulmonary blood flow until the child has outgrown the BT shunt and a Rastelli operation will then be performed. This usually occurs before two years of age.
Senning and mustard procedures
The first surgical treatments of TGA were the atrial switch operations, performed by Senning and Mustard for the first time in 1958 and 1964, respectively. Senning and Mustard procedures (also known as atrial switch procedures) provide physiological, but not anatomic correction of the TGA. During the Senning procedure, an autologous tissue from the right atrial wall and intra‐atrial septum is used to create an intracardiac baffle redirecting systemic venous blood via the mitral valve to the LV and pulmonary venous blood via the tricuspid valve to the RV [67]. The Mustard procedure was developed as a modification of the Senning technique, where the interatrial septum is excised to create a large ASD and a baffle, made of either autologous pericardium or a synthetic material, redirects systemic and pulmonary venous blood flow to the LV and RV, respectively (Figures 29.14 and 29.15). Despite the Mustard procedure being a simplified technique, many deem the Senning procedure to be superior due its use of an autologous atrial tissue, thus allowing atrial growth and preserving contractility.

Figure 29.10 Technique for the arterial switch operation. (1) The great arteries are transected above the sinuses of Valsalva. (2) The coronary arteries are excised from the aorta, transposed posteriorly, and reanastomosed to the pulmonary artery trunk: the neo‐aorta. (3) The distal aorta is brought behind the pulmonary artery (LeCompte maneuver) and anastomosed to the neoaorta. (4) Completed repair. Ao, aorta; PA, pulmonary artery; LCA, left coronary artery; RCA, right coronary artery; RV, right ventricle; LV, left ventricle; NPA, neo‐pulmonary artery; NAo, neo‐aorta.
Réparation à l’Etage Ventriculaire (REV) and Nikaidoh procedures
Réparation à l’Etage Ventriculaire (REV) involves radical excision of the outlet septum to enlarge the VSD, then a patch closure to direct the LV flow to the aorta. The PA is transected with the distal end brought anterior to the aorta (LeCompte) and the proximal stump ligated. The distal PA is then augmented with an autologous patch and anastomosed directly to the RV creating RV‐PA continuity [69] (Figure 29.16). REV avoids the use of an extra‐cardiac prosthetic conduit and reduces the risk of intracardiac tunnel obstruction. It is feasible in patients with anatomic contraindications to the Rastelli operation, and it may reduce the need for re‐operation and prevalence of residual pulmonary outflow tract obstruction. Disadvantages are the lifelong implications of pulmonary regurgitation and tension of the pulmonary artery reconstruction leading to early RVOTO [71].
During the Nikaidoh procedure, the aortic root is completely mobilized and translocated posteriorly to overlie the LV. The VSD is closed, and the PA is translocated anteriorly (LeCompte) and anastomosed to the RV using an autologous pericardial patch to reconstruct the RVOT (Figure 29.17). Patients with a hypoplastic right ventricle benefit the most from the Nikaidoh procedure. It creates a more normally aligned right and left outflow tracts, reducing the risk of recurrent LVOT obstruction and RV‐PA compression by the sternum.

Figure 29.11 Three‐dimensional gadolinium‐enhanced magnetic resonance angiography in a patient with transposition of the great arteries showing the bifurcation of the pulmonary arteries arising from the right ventricle (blue) in front of the aorta arising from the left ventricle (red) after arterial switch operation with the LeCompte procedure.
(Source: Voges et al. [64]. Reproduced with permission of Wolters Kluwer Health, Inc.)
Outcomes for ASO
Long‐term outcomes after ASO are excellent, with over 95% survival at 25 years after discharge [72]. ASO also provides very good functional status and little limitation in physical activity, with over 95% of patients in NYHA functional class I [51]. These outcomes are particularly favorable in comparison to atrial switch operations, Mustard or Senning, where long‐term survival is 80% at 20 years and 75% at 25 years respectively [73]. Despite excellent survival, patients are at risk for morbidity that may require further surgical intervention into adulthood (Table 29.3). Overall freedom from catheter or surgical re‐intervention is 75% at 20 years, with re‐intervention being significantly more common in the TGA/VSD cohort post ASO [75, 76]. The most common reason for re‐ intervention post ASO is pulmonary artery stenosis, possibly due to inadequate growth at the neo‐pulmonary anastomotic site [76]. Performing ASOs in the neonatal period reduces the need for a PA band, which, alongside the introduction of the LeCompte maneuver, has likely reduced the incidence of PA stenosis, resulting in <5% now requiring reintervention [51, 77, 78].
Coronary artery insufficiency has been a long‐held concern after ASO and led to high early hospital mortality when ASO was first introduced. As surgical techniques and experience have improved, early mortality from coronary artery complications has dropped significantly. Coronary artery complications are the primary cause of early mortality with ischemia or ventricular dysfunction most commonly presenting in the first 3 months postoperatively [79]. This is likely due to anatomical kinking, torsion, or external compression, thus reducing coronary perfusion. Long‐term outcomes are also dependent on the patency of the coronary arteries, although late deaths due to coronary insufficiency are rare. It is thought that late coronary artery insufficiency is caused by stretching of the coronary arteries as they grow or progressive fibrocellular intimal thickening [80]. This may also predispose to early atherosclerotic disease, which is screened for during long‐term follow up. Coronary lesions occur in around 3 to 6.8% of patients, with coronary angiography being the gold standard as lesions are often subclinical [75, 79, 81]. This may be due to collateral circulation, which may mask ischemia on noninvasive screening modalities (ECG, echocardiography, stress tests, etc.) [79].

Figure 29.12 Layout of the chest before sternotomy closure in the arterial switch operation. PA, pulmonary artery catheter; LA, left atrial catheter.

Figure 29.13 Rastelli procedure for repair of transposition of the great arteries with ventricular septal defect (VSD) and left ventricular outflow tract obstruction (LVOTO). (A) Baffle closure of the VSD with a patch redirecting left ventricular outflow across the aortic valve. (B) In the presence of LVOTO, the proximal pulmonary artery (PA) is ligated, and a valved conduit is placed from the RV to the main PA. Ao, aorta; PA, pulmonary artery; RA, aright atrium; LA, left atrium; RV, right ventricle; LV, left ventricle; DAo, descending aorta.

Figure 29.14 Postoperative specimen of transposition of the great arteries after the Mustard operation. The tomographic cut is comparable to an apical four‐chamber echocardiographic projection. The patch within the atrium (black arrowheads) directs pulmonary venous blood to the tricuspid valve and into the right ventricle (RV). LA, left atrium; PV, pulmonary vein; RA, right atrium; VS, ventricular septum.
(Source: Warnes [68]. Reproduced with permission of Wolters Kluwer Health, Inc.)
In the immediate post‐op period after ASO, there is a high incidence of chronotropic impairment due to sympathetic denervation, despite this over 96% of patients are in sinus rhythm [51, 79]. Arrythmias present after the immediate post‐op period require urgent investigation to rule out coronary artery abnormalities or residual lesions resulting in hemodynamic compromise. Late arrythmias are a relative frequent complication occurring in around 2 to 10% of patients [51, 79, 82, 83].

Figure 29.15 The Mustard procedure. An intra‐atrial baffle diverts blood from both the superior vena cava (SVC) and the inferior vena cava (IVC) across to the mitral valve and left ventricle, which ejects blood to the pulmonary artery. Pulmonary venous blood is returned via the tricuspid valve and RV, which ejects blood into the aorta. The baffle is made from pericardium or a synthetic material. Ao, aorta; RV, right ventricle; LV, left ventricle; DAo, descending aorta.
Neo‐aortic valve regurgitation is a frequent finding after ASO as the native pulmonary valve is exposed to systemic pressures. Up to 20% of patients develop some degree of aortic regurgitation, with 5% of patients requiring neo‐aortic root or valve reoperation at 20 years, increasing to 8% at 25 years. This demonstrates the significant attrition of the neo‐aortic valve over time and the need for ongoing close surveillance of ASO survivors. It is not yet known how well the neo‐aortic root will cope with systemic pressure as these patients reach middle age [84, 85].

Figure 29.16 This figure shows the innovations of the REV procedure relative to the Rastelli procedure, (A) preoperative anatomy, with resection of the muscular outlet septum (B) and use of LeCompte maneuver (C) which avoids the use of an extacardiac conduit.
(Source: Martins et al. [70]. Reproduced with permission of Cambridge University Press.)

Figure 29.17 The illustration shows the major steps of Nikaidoh’s procedure. (A) Shows the areas for harvesting the aortic root and transection of the pulmonary trunk. In (B), the aorta and pulmonary trunk have already been disconnected from their previous insertion, and the line of division of the outlet septum is shown. (C and D) show aortic translocation and reconstruction of the left outflow tract, with a pericardial patch used to close the ventricular septal defect. In (E) and (F), the pulmonary trunk is sutured to the right ventricular outflow tract, and the reconstruction is completed with a pericardial patch.
(Source: Martins et al. [70]. Reproduced with permission of Cambridge University Press.)
With increased survival after TGA repair, attention has turned toward quality of life and functional outcomes. There is increasing evidence that neurodevelopmental impairment is more prevalent in children after TGA repair than in the general population [86]. Neurodevelopmental impairment in these children ranges from motor delay, cognitive impairment, poorer academic achievements, speech and language dysfunction and psychosocial impairment. Multiple studies have described MRI changes in both white and grey matter locations, including focal ischemic changes and PVL in patients with TGA, with reported prevalence rates of 30% [41]. However, MRI abnormalities poorly predict adverse neurodevelopmental outcomes in these patients [58]. Poorer neurodevelopmental outcomes are likely to be multifactorial and strongly associated with the infant’s prenatal, neonatal, and perioperative course. Risk factors include post‐natal diagnosis of TGA, greater degree of hemodynamic instability and hypoxemia, underlying genetic abnormality, inadequate nutrition, complex TGA, prolonged bypass times, peri‐operative complications (e.g., NEC), use of DHCA, and need for ECMO [86]. This highlights the importance of fastidious attention to neuroprotection in the perioperative period, with optimal timing of surgery, anesthetic and surgical techniques, and monitoring to improve neurodevelopmental outcomes and quality of life in ASO neonates.
Table 29.3 Postoperative sequelae following the arterial switch operation
Source: Wernovsky et al. [74]. Reproduced with permission of John Wiley & Sons.
Postoperative sequalae | Incidence |
---|---|
Supravalvar pulmonary stenosisa | ~10% |
Supravalvar aortic stenosisa | <5% |
Neoaortic root dilatation | Essentially 100% |
Neoaortic regurgitation | ~50%b |
Significant arrythmia | Rare |
Asymptomatic coronary occlusion | 2–3% |
Pulmonary hypertension | Rare |
Hypertrophied bronchial collateralsa | <5% |
Abnormal bronchial collateralsa | Unknown |
Sudden death | Rare |
a Requiring intervention.
b Increase with increasing duration of follow‐up.
Outcomes for Rastelli
Overall survival after Rastelli operation has improved drastically in the last few decades from 52 to 93% at 20 years [87, 88]. Despite this, the re‐intervention rate remains high, with approximately 40% of patients requiring re‐intervention for RVOTO at 20 years [88]. The conduits do not grow with the child, become stenosed overtime, and are at a risk of calcification; therefore, serial conduit replacements are inevitable. However, extension of the VSD at the time of operation has nearly eliminated the need for reintervention for LVOTO. Atrial and ventricular arrythmias occur commonly and sudden death from arrythmia is a problematic long‐term complication. Although RV and LV failure may also occur, the Rastelli operation protects the RV from chronic regurgitation, unlike the REV and Nikaidoh procedures.
Outcomes for Senning and Mustard procedures
Mustard and Senning procedures have low operative mortality, and overall survival for all patients is approximately 75% after 25 years [73]. Long‐term sequelae, however, are common and are largely related to RV failure, arrythmias, and baffle complications. Mustard and Senning procedures were the original mainstay for surgical correction of TGA, but as a result of the morphological RV remaining as the systemic ventricle, they have been largely superseded by the ASO. All adults develop some degree of RV dysfunction, with 10% of adults requiring hospital admission for CHF after 20 years [73, 89]. Common baffle‐associated complications include leak, stenosis, and/or intractable atrial arrhythmias [90]. Scarring around the sinus node and intra‐atrial baffle sutures results in sick sinus syndrome and atrial flutter, respectively. Sudden, presumed arrhythmogenic death and myocardial failure are the most frequent causes of late death in these patients [91].
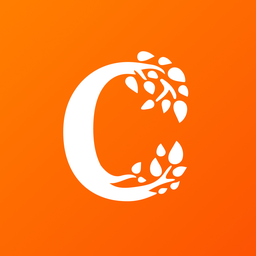
Full access? Get Clinical Tree
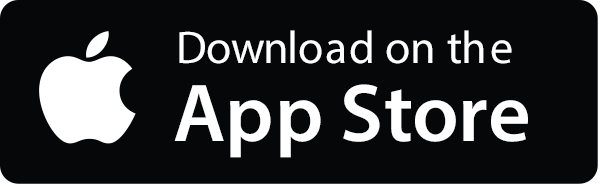
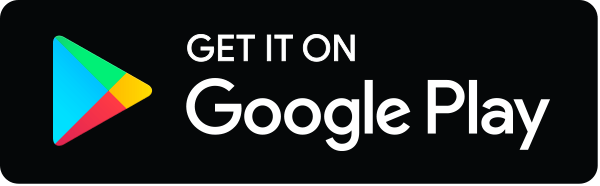