Michael L. Schmitz1, Destiny F. Chau2, R. Ryan Das3, Lorraine L. Thompson4, and Sana Ullah5 1 Chief, Pediatric Cardiac Anesthesiology, Log A Load for Kids of Arkansas Endowed Chair in Congenital Cardiac Disorders, Pediatric Cardiothoracic Surgery, Pediatric Anesthesiology and Pain Medicine, Arkansas Children’s Hospital, University of Arkansas for Medical Sciences, Little Rock, AR, USA 2 Professor of Anesthesiology, Pediatric Cardiothoracic Anesthesiology, Arkansas Children’s Hospital, University of Arkansas for Medical Sciences, Little Rock, AR, USA 3 Associate Professor of Anesthesiology, Arkansas Children’s Hospital, University of Arkansas for Medical Sciences, Little Rock, AR, USA 4 Pediatric Anesthesiologist, Ellensburg, WA, USA 5 Associate Professor of Anesthesiology and Pain Management, Children’s Medical Center Dallas & UTSW Medical Center, Dallas, TX, USA Right‐sided obstructive congenital heart disease (CHD) is extremely variable in presentation – both in severity and clinical signs and symptoms. In some cases, severe cyanosis and/or congestive heart failure (CHF) is immediately apparent in a neonate, whereas in other cases, a teenager or adult may present with mild complaints of fatigue or reduced exercise ability. Variations in the extent of malformations in the right heart and great vessels dictate the severity and timing of presentation. All right‐sided obstructive diseases can cause right‐to‐left shunting of blood flow. Obstruction to blood flow in the right heart can result from malformations at a single anatomical area, such as the right atrioventricular (AV) valve, or multiple structural sites, as found in the tetralogy of Fallot (TOF). Failure of tissue formation and coordinated growth leads to poor development of valvar structures and incomplete septation of the right heart from the left. Impairment of normal hemodynamic blood pressures and flows from structural anomalies adds further malformations such as chamber dilation or hypertrophy as well as abnormal blood vessel growth and formation. Right‐to‐left shunting is present in patients who manifest cyanotic right‐sided obstructive heart disease. Examples include deoxygenated blood passing to the left heart via a patent foramen ovale (PFO) or atrial septal defect (ASD) as might be found with severe Ebstein anomaly (EA) or a ventricular septal defect (VSD) in TOF. Those patients who do not present with neonatal cyanosis may manifest periodic cyanosis from variations in shunt direction, depending on the relative pressures between the right and left chambers of the heart. When right heart pressures are higher than left, the right‐sided hypertension can be partially relieved by the right‐to‐left blood flow when septal defects are present. With severely restrictive septal defects, inadequate relief of right‐sided hypertension manifests with CHF. The degree of neonatal systemic hypoxemia that accompanies right‐sided obstruction is also dependent on the patency of the ductus arteriosus. Pulmonary blood flow (PBF) in the presence of severe obstructions of the right heart, such as with critical pulmonary stenosis (PS) or pulmonary atresia (PA), might be entirely dependent on a patent ductus arteriosus (PDA). Affected neonates can rapidly show signs of increasing hypoxemia, such as respiratory distress and deepening cyanosis when the ductus arteriosus begins to close shortly after birth unless the ductal patency is stabilized with exogenous prostaglandin E1 (PGE1). Congenital malformations that impede blood flow through the right heart can occur at a single critical anatomical area or a combination of areas. These include the right AV valve, the outflow tract of the right ventricle (RV), the pulmonary valve (PV), and the main pulmonary artery (MPA) and/or branch pulmonary arteries (BPAs). Commonly, congenital malformations affect several of these critical areas simultaneously, such as in TOF. Malformations can occur directly as a result of aberrant movement of tissues during development or indirectly as a result of impaired flow hemodynamics due to misaligned structural anatomy. Often the resultant congenital heart deformity is a combination of both processes. The physiology of right‐sided obstructive defects and the changes that occur with palliative or reparative intervention in the context of perioperative anesthetic care and planning for such patients are described in this chapter for: Other right‐sided obstructions such as those that result in a single functional ventricle (e.g. tricuspid atresia) are covered elsewhere in this book. See Chapter 30. Although EA occurs in only 0.3–0.7% of CHD, it is the most common congenital malformation of the tricuspid valve (TV) [1]. The incidence is approximately 1/10,000 live births [2]. EA was first described by Ebstein in 1866 [3] from necropsy findings. Clinical diagnosis was not achieved until 1949 [4] because the clinical presentation of more severe cases with diminished PBF and cyanosis resembled TOF, and partial competence of the malformed TV often was enough to prevent signs of CHF until late in evolution of the disease [5]. EA is characterized by a malformed TV and RV [6] (Table 28.1). With advent of cardiac catheterization and echocardiography, cardiologists were much more able to diagnose EA with certainty. The highly varied range of dysplastic characteristics of EA causes extreme variations in clinical presentation from the neonate to the adult [7–9] (Figures 28.1–28.2, Table 28.1). The septal and posterior leaflets vary in the apical displacement of their attachments, and in approximately one third of cases the septal and/or posterior leaflets may remain adherent to the ventricular myocardium due to a failure of delamination. The anterior leaflet is attached at the functional annulus in a position superior to the other leaflets, but it is morphologically abnormal. Large with apparent redundant tissue, the leaflet frequently appears sail‐shaped, often with fenestrations, and with abnormal RV attachments at the boundary of the inlet and trabecular areas. The anterior leaflet and/or the shortened chordae/dysplastic papillary muscles can restrict blood flow from the atrium/atrialized RV to the trabecular RV. Slits or perforations in the abnormally fixed anterior leaflet can be a significant obstruction. The shortened chordae extend from dysplastic papillary muscles. The trabecular RV is small, and sometimes very small, lacking an inlet chamber. Trabecular RV myocardial function can be normal or impaired with thin walls. An interatrial shunt is almost invariably present as over one third will have an ASD and most of the remainder will have a PFO. Table 28.1 Characteristics of the malformed tricuspid valve and right ventricle in Ebstein anomaly The line formed at the site of the distally displaced tricuspid leaflets serves as a functional TV ring and is the boundary between the trabecular and atrialized portions of the RV (Figure 28.3). Above this line of the distally displaced TV leaflets, the inlet portion of the anatomical RV serves as part of the RA. The atrialized portion of the RV is often thin and dilated with an abnormal ventricular electrical conduction pattern. Extreme thinning of the atrialized or inlet RV wall causes it to exhibit paradoxical movement with ventricular systole and dilation with RA contraction. The RA is dilated and sometimes massively dilated. The dilation of the atrialized RV creates an abnormal position for the interventricular septum, compromising left ventricular (LV) geometry and reducing the size of the LV chamber. There is increased fibrosis in both ventricles. On prenatal ultrasound screening, the most severe forms of EA present with hydrops fetalis characterized by a massively enlarged heart and pulmonary hypoplasia. Lesser affected infants will have a neonatal course dependent on the severity of the EA defects, ranging from asymptomatic to cyanosis of varying degrees. Cyanotic neonates with severe obstruction will show signs of CHF that could lead to death. In general, cyanotic neonates with EA require intervention for survival. For lesser affected infants, the clinical presentation ranges from asymptomatic to progressive CHF depending on the degree of apical displacement of the TV. Diagnosis is sometimes delayed until symptoms present in older children or young adults, and less commonly in middle age or later. Generally, exercise tolerance is minimally impaired in the mildly affected unless the foramen ovale is not patent. Adults who present with CHF from long‐term effects of tricuspid regurgitation (TR) have a poor prognosis without definitive intervention. Severity cyanosis in children and young adults with EA is associated with a poor prognosis. Even milder forms of EA that present in late childhood, adolescence, or young adulthood often result in death from CHF in the second and third decades of life without surgical intervention. For those asymptomatic individuals who have not developed CHF, up to 20% may die of atrial and ventricular arrhythmias, sometimes as a sudden death. Paradoxical emboli, abscess formation, and less commonly endocarditis contribute to later fatalities [10]. Figure 28.1 (A) Normal tricuspid valve attachments at the atrioventricular junction (dotted line) and the direction of the hinge line (dashed line) of the valve in Ebstein malformation. (B) The location of the functional orifice of the abnormal valve (black ovals) as observed in the series of hearts examined by Schreiber et al. [7]. aRV, atrialized right ventricle; CS, coronary sinus; fRV, functional right ventricle; IVC, inferior vena cava; RA, right atrium; SVC, superior vena cava. (Source: Dearani et al. [8]. Reproduced with permission of Cambridge University Press.) Figure 28.2 Carpentier classifications of four anatomical types of Ebstein anomaly. (A) Small, contractile, atrialized chamber (the “atrialized right ventricle, aRV”) with mobile anterior leaflet with no or limited (inset drawing) obliteration of the interchordal space and moderate apical displacement of septal and posterior leaflets. (B) Large, noncontractile atrialized chamber with no or limited interchordal space obliteration and mobile anterior leaflet with marked apical displacement of septal and posterior leaflets. (C) Anterior leaflet with restricted motion due to obliteration of interchordal spaces and attachment of the inferior edge to the ventricle and fibrous bands attaching other leaflet areas to the infundibulum, marked displacement of septal and posterior leaflets that may be severely hypoplastic. Atrialized chamber is large with a thin, noncontractile wall. (D) Leaflet tissue forms a continuous sac adherent to the dilated right ventricle, creating a “tricuspid sac,” with obliterated interchordal spaces. RA: right atrium; RV: right ventricle. (Source: Carpentier et al. [9]. Reproduced with permission of Elsevier.) The TR of EA and the degree of downward displacement of the TV variably impair adequate development of the heart. Diminished flow through the RV in utero may play a role in the development of right ventricular outflow tract obstruction (RVOTO), PS, and even PA. Significant TR places a volume load on the RV that results in a largely dilated RV that impairs LV function. Severe TR produces a massively ballooned RA. As pulmonary vascular resistance (PVR) decreases and stabilizes to near adult values, forward flow in the neonatal right heart can cause EA signs to improve [11, 12]. A cyanotic neonate with an unrestrictive ASD may eventually become acyanotic with acceptable cardiac output (CO). However, an infant with a restrictive ASD can have a low CO due to LV impairment from malposition of the interventricular septum with paradoxical motion. Figure 28.3 (A) Normal heart, and (B) heart with Ebstein anomaly. Carpentier class A (dashed white line indicates level of atrioventricular junction). aRV, atrialized right ventricle; LA, left atrium; LV, left ventricle; RA, right atrium; RV, right ventricle. (Source: Michael L. Schmitz, MD.) The distortion of atrial and ventricular chambers with EA is associated with arrhythmias that can further impair function and be life‐threatening. As many as 25–30% of individuals will have atrial flutter, atrial fibrillation and paroxysmal supraventricular tachycardia. Other electrophysiological abnormalities are also frequently present [1] (Table 28.2). Familial genetically inherited forms of EA are sometimes associated with LV noncompaction cardiomyopathy. In fact, the type of CHD most associated with this myopathy is EA. This combination is associated with mutations in the B‐myosin heavy chain gene MYH7. Although autosomal dominant, gene penetrance is variable as some individuals do not have cardiac abnormalities [13]. Characteristics of LV noncompaction include prominent trabeculations with deep intratrabecular recesses that give the LV a spongy appearance on cardiac ultrasonography. Added risks of CHF, arrhythmias, thromboemboli, and sudden cardiac death occur with this combination [14]. Recent genetic analyses of patients with EA suggest multiple genetic associations with genes involved with myocardial development or function other than MYH7 including NKX2‐5, TTN, MYH6 as well as a number of various single nucleotide alterations, genetic locus deletions/duplications and copy number variants [15] not infrequently associated with cardiomyopathies. Table 28.2 Major electrophysiologic abnormalities in Ebstein anomaly Progressive right heart failure resulting from TR, an inadequately sized RV pumping chamber, and other right‐sided obstruction combined with the EA TV are sometimes indications for surgery in the infant or child. Otherwise, moderate CHF can be treated with diuretic therapy, perhaps combined with digoxin. Medical therapy and perhaps catheter ablation can be used to control some types of arrhythmias. However, if deterioration of the TV and RV appears progressive, long‐term outcome will be improved with earlier surgical intervention [16]. The timing of intervention for EA is determined by the degree of apical displacement of the functional TV, the competency of the TV, the presence of PA or circular shunting, and the age of the patient (Figure 28.4). Depending on the size of the trabecular RV, patients may undergo two ventricle repair or be palliated with one‐and‐one‐half (1.5) or single ventricle surgical pathways. Timing of intervention in neonates also takes into account potential RVOTO and allowance for PVR to resolve to near adult pressures. Only the most severely compromised infants are generally considered for cardiac transplantation [11] such as those with poor biventricular function and/or uncontrollable arrhythmias. Decision‐making for the type of surgical repair or palliation depends first on the age of the patient and secondly on two key elements: the TV morphology and the size of the trabeculated RV (Figure 28.5). A number of infants with adequate trabecular RVs have successfully undergone two ventricular repairs. In 1958 after studying EA heart specimens, Hunter and Lillehei [17] suggested a potential repair for the TV but advances in valvuloplasty ultimately led to the first successful EA TV repair in 1963 [18]. From 1972 onward, further refinements in valvuloplasty by Danielson et al. [19] and others have improved survival for patients with EA (Table 28.3). Techniques for valvuloplasty of the EA TV have evolved for nearly 60 years. A technique introduced in 1972 altered the anterior leaflet of the TV such that it would approximate the ventricular septum or the small, malformed septal leaflet during systole. Coaptation of the TV was facilitated by plicating the TV annulus at the apical boundary of the atrialized RV to reduce annulus diameter [19]. Further modifications for mobilizing and fixing the anterior and posterior leaflets at the true tricuspid annulus while utilizing a valve ring ensued [9]. Some infants with adequately sized RVs have done well with an aggressive two‐ventricle repair that included reconstruction of a monocuspid TV from the anterior leaflet with or without translocation of the leaflets to the true annulus, ventriculorrhaphy, reduction atrioplasty, subtotal closure of the ASD, and repair of other associated defects [20]. Others have focused on a bicuspid TV repair without repositioning the valve to the true annulus [21]. Figure 28.4 Algorithm for treatment of neonatal Ebstein anomaly. CO, cardiac output; NO, nitric oxide; PGE1, prostaglandin E1; PVR, pulmonary vascular resistance. (Source: Michael L. Schmitz, MD.) Figure 28.5 Algorithm for neonatal Ebstein anomaly surgical intervention. PA, pulmonary artery; PV, pulmonary valve; RV, right ventricle; TV, tricuspid valve. (Source: Michael L. Schmitz, MD.) Table 28.3 Developments in the treatment of Ebstein anomaly Building on a previous technique, da Silva et al. [22] developed a method known as the cone reconstruction that enhances available valve tissue by surgically delaminating leaflets attached to the RV wall and septum. The cone reconstruction of the TV for EA began to be developed in 1989 and has evolved to become a successful option for patients with adequate available valvar tissue and trabecular RV chambers. The TV leaflets are mobilized and reattached to the true TV annulus. The anterior and posterior leaflets are sutured together into a cone shape, incorporating the septal leaflet into the cone when possible. The atrialized RV is longitudinally plicated. Flexible annuloplasty rings (partial rings for children to allow for growth) are sometimes used to add stability to the dilated TV annulus [23] (Figure 28.6). Although cone reconstruction has been successful in neonates [24, 25] and small infants [26], valve rings are not an option for these small patients. When tissue is found to be insufficient, leaflets can be augmented with patch material [27, 28] (Figure 28.7). The cone operation improves coaptation of the TV leaflets and allows for centralized flow. Figure 28.6 Key components of the cone repair. (A) Standard aortic and bicaval cannulation. Right atriotomy is parallel to the atrioventricular (AV) groove. Anatomic examination of the tricuspid valve (TV) and the atrialized right ventricle (RV). Membranous septum and AV node are marked by small vein (vein of D). (B) Delamination of the TV. First incision is made at 12 o’clock in the anterior leaflet a few millimetres away from the true annulus. Surgical delamination is the division of the fibrous and muscular attachments between the body of the leaflet(s) and the free wall of the RV. Delamination must continue to all attachments between the AV groove and the leading edge of the leaflets (close to the RV apex) while keeping the attachments at the leading edge of the leaflet intact. (C) Rotation of leaflet tissue. Following mobilization of all leaflet tissue, the inferior leaflet, or most medial aspect of the anterior leaflet, is rotated clockwise to meet the mobilized septal leaflet. These leaflets are approximated with suture. At completion, the neo‐TV orifice should be composed of 360° of leaflet tissue. Prior to reattachment, the atrialized RV is examined to determine need for RV plication. (D) RV plication. Internal triangular plication of portions of the atrialized RV reduces tension on the repair, reduces size of the TV annulus, and eliminates noncontractile RV. Plication is started close to the RV apex and proceeds to the AV groove. Care must be taken to avoid injury to the right coronary artery. (E) Attachment of neo‐TV to the true annulus. The septal leaflet is reattached to the ventricular septum (just caudal/ventricular side of the true annulus). (F) Repair can be reinforced with either a felt band (in younger children) or an annuloplasty band (older children and adults). CS, coronary sinus; IVC, inferior vena cava; LV, left ventricle; PFO, patent foramen ovale; RA, right atrium; RCA, right coronary artery; SVC, superior vena cava; TTA, true tricuspid annulus. Copyright © Mayo Foundation for Medical Education and Research. (Source: Holst et al. [23]. Reproduced with permission of Mayo Foundation for Medical Education and Research.) Figure 28.7 Tricuspid valve (TV) leaflet augmentations for the cone repair. Leaflet augmentation can be accomplished with either autologous pericardium or CorMatrix membrane to increase the height of the anterior TV leaflet (A) or to increase the TV diameter at the annulus (B). When there is a linear attachment of the leading edge of the anterior TV leaflet to the right ventricular endocardium, autologous neochordae can be fabricated with surgical fenestrations (C). (Source: Dearani et al. [29]. Reproduced with permission of Elsevier.) Utilization of a semi‐functional RV by combining RV output to the MPA from the inferior vena cava (IVC) with a bidirectional Glenn (BDG) anastomosis in a 1.5 ventricular repair can be beneficial. Compared to patients converted to single ventricle physiology, 1.5 ventricular pulsatile flow to the pulmonary arterial tree might reduce MAPCA development while inclusion of hepatic venous flow to the pulmonary arterial tree might reduce formation of pulmonary arteriovenous malformations. Even a hypoplastic RV may increase CO with increased cardiac demand beyond what could occur with a Fontan circulation. The BDG can be used to reduce RV preload to hypoplastic EA RVs or those with moderate degrees of TV stenosis, allowing for closure of a coexisting ASD or PFO. In some cases of severe EA, there may be no identifiable TV leaflet tissue available for reconstruction. In the absence of available leaflet tissue combined with a severely dilated dysfunctional RV and secondary LV compression, a fenestrated RV exclusion procedure with a systemic‐to‐pulmonary arterial shunt may be the best option for an infant [30]. The modified Starnes procedure is accomplished by placing a patch with a 5 mm fenestration at the level of the true tricuspid annulus while allowing the coronary sinus to remain on the RA side of the patch. The fenestration allows for thebesian venous drainage from the excluded RV to the RA. If there is pulmonary arterial insufficiency, the RV to pulmonary connection is interrupted. As with all single ventricle palliations, an unrestricted atrial communication is ensured. Later, the infant or child may advance to a BDG or Fontan palliation. A limited follow‐up for 12 patients has shown that the RV involutes sufficiently to allow improved geometry and function of the single LV [31]. The Sano RV exclusion technique [32] for EA is similar but employs an RV to pulmonary artery conduit rather than a systemic‐to‐pulmonary shunt to provide PBF. The advantage of the modified Starnes procedure over the Sano RV exclusion technique is that the already reduced functional RV myocardium is less compromised without the ventriculotomy required for the RV to pulmonary artery conduit. Complications of the cone procedure are similar to other TV repairs for EA. Inadvertent tricuspid stenosis or suture dehiscence are possible. Sutures too near the AV node can cause third‐degree heart block. Distortion caused by plication of the atrialized RV and TV annulus can impair right coronary artery blood flow. Outcomes are compromised when neonatal EA is associated with RVOTO, PA, small pulmonary arteries, or any congenital disease that causes LV dysfunction. Rhythm disturbances can occur at any time with EA. Temporary pacing wires should be placed on the RA and RV near the conclusion of surgery as postoperative pacing could be necessary. Generally, teenaged and adult patients who do not require postoperative pacemakers have fewer rhythm disturbances on follow up [33]. Wolff‐Parkinson‐white accessory pathways, often near the orifice of the TV, are present in 10–20% of EA. Outcome studies are limited for the cone reconstruction. A follow‐up of 52 patients ranging in age from 0.25 to 49 years (mean 18.5 ± 13.8 years) showed that the cone repair maintained its integrity and there was an increase in RV function relative to body surface area indexing. Two of the 52 died postoperatively in‐hospital and one was lost to follow up resulting in a statistical survival of 86.2 ± 8%f at 7 years. Of the followed 49, four underwent TV revision (3, 4, 5, and 10 years postoperatively), one received a pacemaker for intermittent episodes of complete AV block 1 year after surgery, three had atrial arrhythmias (one successfully ablated and the other two medically controlled). Eleven had Wolff‐Parkinson‐White syndrome and had the anomalous bundle surgically sectioned; ten were successful and one had repeated ablations, developed TR, and then underwent TV revision 5 years postoperatively [34]. A single institution review of 151 EA patients with a mean age of 28 years (56 patients less than 18 years of age) who underwent either cone reconstruction (n = 38, age 31 ± 19 years), conventional repairs (n = 94, age 26 ± 18 years), or TV replacement (n = 19, age 36 ± 21 years) presented mid‐term follow up comparisons for cone repair, conventional repairs, and TV replacement. Cardiopulmonary bypass (CPB) times as well as aortic cross clamp times were longer for cone repairs than either conventional repairs or TV replacement. Cone repair was more successful than conventional repairs at mid‐term follow up (8 years) with moderate or less TR (p = 0.031) and a reduced incidence of post‐repair severe TR that did not reach clinical significance. Of note, most of the cone repairs were performed more recently than many of the conventional repairs [35]. In the largest series to date from the Mayo Clinic [28], 235 consecutive cone repairs for patients with EA and a median age of 15 years (range 6 months to 73 years) showed only one early death of a 50‐year‐old from low CO syndrome related to endocarditis from pacemaker leads. Extracorporeal membrane oxygenation (ECMO) was needed for the one adult early mortality patient and for 5 pediatric patients with severe preoperative RV dysfunction and dilation. Cone repair was the primary correction in 80% and a re‐repair in 12%. Early reoperation for recurrent TR occurred in 5.9% (7 children and 7 adults). TV replacement was ultimately required for 6/7 adults, but 6/7 children had a successful reoperation. Of those requiring reoperation, 11 of the 14 were in the first third of the series, suggesting a learning curve for a successful cone repair. Supplemental techniques were used in many, including the BDG anastomosis in approximately 20% (41 children and 5 adults), annuloplasty bands, leaflet augmentation, and use of neochordae. In general, TV replacement is reserved for irreparable TV and RV anatomy as reoperation and repeat cardiotomy for TV replacements will likely be necessary for the patient’s lifetime. In addition, options for prosthetic valves are limited or non‐available for small infants and children. Some teenaged or adult patients with late diagnoses might have incurred TV leaflet damage from turbulent blood flow or endocarditis that preclude successful repair, and TV replacement may be the best option. For select patients such as the elderly, TV replacement might be considered if advantages of lessened CPB time outweigh the longer time needed for TV repair. Bioprosthetic valves are preferable in the tricuspid position due to their lower thrombogenicity over mechanical valves. When placing the prosthetic TV, care is exercised in avoiding the conduction system on the septal side and the right coronary artery on the anterior and inferior sides of the implanted TV as well as avoiding the right ventricular outflow tract (RVOT) and impingement of the valve struts on the AV node. Similar considerations will apply to future development of percutaneous TV replacement. Older children and adults with preoperative paroxysmal atrial fibrillation or atrial flutter might be candidates for a modified right‐sided maze procedure or cavotricuspid isthmus ablation using either radiofrequency or cryoablation [23] (Figure 28.8). Those with continuous atrial fibrillation may do better with a biatrial maze [36]. For neonatal presentation of EA, signs of right heart failure and systemic hypoxemia are most critical. Dyspnea, cyanosis, and hepatomegaly suggest that the infant should be supported such that demands on CO and oxygen consumption are minimized. The most severely affected infants benefit from intubation, mechanical ventilation, paralysis, and sedation to reduce work of breathing and demands on CO. Oxygen, inhaled nitric oxide (iNO, if pulmonary hypertension is suspected), inotropic support, and possibly PGE1 may increase oxygen delivery. For the less affected infant, signs of fatigue with feeding, feeding intolerance, irritability, diaphoresis, dyspnea, and episodic cyanosis with distress suggest a poorly functioning heart. Oxygen, milrinone, and possible PGE1 may be beneficial prior to anesthesia. Some neonates will manifest a gradual improvement in right heart forward flow and a reduction in right‐to‐left shunting and cyanosis as TR declines. Neonatal surgical intervention, when possible, is delayed until PVR has declined significantly. Figure 28.8 Cryoablation for atrial flutter/fibrillation in Ebstein anomaly. AVN, atrioventricular node; CS, coronary sinus; FO, foramen ovale; IVC, inferior vena cava; SAN, sinoatrial node; SVC, superior vena cava; TV, tricuspid valve. Copyright © Mayo Foundation for Medical Education and Research. (Source: Holst et al. [23]. Reproduced with permission of Mayo Foundation for Medical Education and Research.) For the toddler, older child, adolescent, or even young adult, functional symptoms of dyspnea with exertion, excessive fatigue with daily activities or as compared to peers, and certainly signs of cyanosis as well as the trends in symptomatology in recent months give some insight into the severity of EA. In the older child and young adult, syncope, chest pain, and palpitations should be investigated for the presence of arrhythmias. Physical examination often reveals a soft, high‐pitched systolic murmur, sometimes accompanied by triple or even quadruple heart sounds. A mid‐diastolic murmur is sometimes found at the left sternal border and apical areas and has a soft, scratchy sound. A persistently split‐second heart sound due to delayed emptying of the RV is usually present and varies little with respiration. Pediatric patients with myocardial failure are diaphoretic, tachypneic, and irritable. Chest auscultation can be notable for rales if LV function is impaired by a dilated RV. Abdominal examination often reveals hepatomegaly on palpation. Moderate to severe cardiomegaly is found on the chest radiograph, and pulmonary vascularity is reduced. The silhouette of the heart is globular due to the enlarged RA. In fact, the electrocardiogram (ECG) usually shows an increased duration for the P‐wave suggesting right atrial enlargement, an increased PR interval, and not unusually a complete or incomplete right bundle branch block. In at least 10–15% of patients, the pre‐excitation patterns of Wolff‐Parkinson‐White syndrome are present. An echocardiogram is usually diagnostic for EA, showing a large TV orifice with apical displacement of the septal leaflet [29] (Figure 28.9). Cardiac catheterization is seldom useful, even for the most commonly associated defects, and place the patient at risk for development of tachyarrhythmias. Magnetic resonance imaging (MRI) can be valuable in estimation of the size of the functional RV and accurately measuring the displacement of the TV leaflets (Figure 28.10). Though both MRI and echocardiography are useful in detecting associated defects, echocardiography may underestimate the degree of TR but is more effective in detecting small shunts from associated defects than MRI [37]. Figure 28.9 Preoperative four‐chamber view echocardiogram evaluation for potential cone repair. (A) Multiple attachments between the anterior leaflet and the RV free wall from base to apex (arrows). (B) The undelaminated septal leaflet (arrows) suggests that this leaflet tissue can be recovered increasing the chances for a successful repair. aRV, atrialized right ventricle; LV, left ventricle; RA, right atrium. (Source: Dearani et al. [29]. Reproduced with permission of Elsevier.) Figure 28.10 Cardiac magnetic resonance imaging of Ebstein anomaly. (A) Short‐axis plan at mid‐ventricular level. (B) Four‐chamber view. (C) Right ventricular inflow‐outflow view. The atrioventricular junction (dotted line) and the functional tricuspid valve orifice (dashed line) define the basal and apical boundaries (respectively) of the atrialized right ventricle (aRV). The functional right ventricle (fRV) lies between the dashed line and the pulmonary valve. The anatomic RV includes both the aRV and fRV. The tricuspid valve functional orifice is rotated apically and superiorly towards the RV outflow tract. Ao, aorta; LV, left ventricle; PA, pulmonary artery; RA, right atrium. (Source: Holst et al. [23]. Reproduced with permission of Mayo Foundation for Medical Education and Research.) Hypoxemic neonates should be managed with interventions aimed to decrease and avoid increasing PVR. For the mechanically ventilated neonate, adequate tidal volumes and minute ventilation must be achieved with minimum airway pressures. Those infants with marginal heart function may benefit from iNO by reducing PVR and enhancing right heart to pulmonary artery flow. In addition, functional and anatomical obstructive components to PBF may be further defined by the addition of iNO as poor right heart function is anticipated to improve with iNO while anatomical obstructions in the RVOT, PV, and PAs will remain. In the first couple weeks after birth, pulmonary arterial blood flow may require augmentation with a PDA maintained with PGE1. However, too much flow through a large PDA can result in cardiac failure and loss of systemic and pulmonary perfusion with a phenomenon known as “circular shunt” [38] (Figure 28.11). Circular shunt results when generous flow to the pulmonary arterial system from the aorta via the PDA follows a retrograde path of least resistance when the PV and TV are incompetent and there is an interatrial communication. Sequentially, flow proceeds from the PDA, BPA, MPA, PV, RV, RA, ASD or PFO, LA, LV, and finally the aorta. Perfusion of the pulmonary capillary bed is diminished and CO to the vital organs is reduced. PGE1 should be discontinued so that the PDA becomes smaller and more resistant to flow, reducing the circular shunt and high output failure [12]. Figure 28.11 Circular shunt: aortic blood enters the lower pressure pulmonary artery (PA) via the patent ductus arteriosus (PDA). Pulmonary insufficiency allows blood to continue retrograde into the right ventricle (RV), right atrium (RA), left atrium (LA), left ventricle (LV), and then again to the aorta (Ao). (Source: Schmitz et al. [38]. Reproduced with permission of John Wiley & Sons.) Most neonates and young infants may not require anxiolytic premedication. Older infants, those who have developed stranger anxiety (approximately 9 months of age), and children often benefit from anxiolytic administration of midazolam. Midazolam may be given orally (0.5–0.75 mg/kg up to 15–20 mg) or intravenously (IV) (0.1–0.15 mg/kg up to 2–4 mg). Anxiolysis can be helpful in reducing or avoiding decreases in PBF that can result with crying or breath‐holding during application of the mask anesthetic. In some cases, a sleeping child who has received dexmedetomidine can be safely transported and undergo a safe anesthetic induction, but this technique requires planning. Loading with 0.5 to 1.0 μg/kg IV over 10 minutes is often sufficient but requires monitored attention for potential bradycardia. For patients who do not yet have IV access and are to undergo a mask anesthetic induction or merely have a non‐stimulating procedure (such as a sedated transthoracic echocardiogram), intranasal dexmedetomidine is administered with a nasal atomizer (2–3 μg/kg, 100 μg/mL) often results in adequate sedation. The time required for mean onset of sufficient sedation is 10–24 minutes for 3 μg/kg [39]. Reducing ambient light and background noise while ensuring that the child is warm and comfortable facilitates the sedative effect. Relative contraindications for dexmedetomidine include poor CO, hypovolemia, bradycardia or risk for bradycardia, AV nodal block, or medications that delay AV conduction or increase vagal tone [40]. An inhalational induction with nitrous oxide and sevoflurane is appropriate for infants and children with mild to moderate EA. Inhalational induction is slowed by interatrial left‐to‐right shunting as well as by decreased CO. Ketamine (1–4 mg/kg) or propofol (1–2 mg/kg) are IV induction alternatives for mild to moderate disease. For those with severe EA, hemodynamic stability is maintained in a euvolemic patient in most instances with IV ketamine (1–4 mg/kg) or etomidate (0.2–0.3 mg/kg) without inducing detrimental myocardial depression or afterload reduction. Sufficient right heart preload must be maintained to maximize the EA right heart forward flow. As the anesthetic progresses, increases in vascular compliance are accommodated with replacement of intravascular volume, such as 5% albumin, crystalloid solutions, or in the anemic patient, packed red blood cells (PRBC). Muscle relaxants are selected based on the anticipated duration of the procedure and the occasional need for a modified rapid‐sequence induction. Rocuronium offers quick action for induction and intubation (0.6–1.2 mg/kg) as well as subsequent maintenance of muscle relaxation (0.1–0.2 mg/kg every 20–30 minutes). Pancuronium is another option with a longer duration of action (0.06–0.1 mg/kg for induction and approximately 0.01–0.02 mg/kg for redosing). Both muscle relaxants induce ganglionic blockade and vagolysis, effecting a higher baseline heart rate. For CPB cases, opioid‐based anesthetics combined with modest levels of potent inhalation agents such as sevoflurane or isoflurane are common. The relative balance of opioids and inhalation anesthetic is based on the patient’s ability to tolerate the myocardial depressant effects of higher ranges of inhaled halogenated agents and the plan for early versus delayed liberation from mechanical ventilation. For patients that will remain intubated with mechanical ventilation after arrival to the intensive care unit (ICU), fentanyl 10–15 μg/kg and 0.5–1.0 minimum alveolar concentrations (MAC) of halogenated agent may be used prior to CPB with a total fentanyl of perhaps 20–30 μg/kg combined with a morphine infusion of 30–35 μg/kg/hour started at the time of incision. If extubation in the operating room is anticipated, total fentanyl administration is approximately 10–20 μg/kg and halogenated agents are utilized at higher MAC as tolerated. A morphine infusion of 25 μg/kg/hour is started by the time of incision. For infants beyond the neonatal period, sedation with dexmedetomidine at 0.5 μg/kg/hour is added for sedation management in the postoperative period whether extubated in the operating room or later in the ICU. Plasmin–binding inhibitor antifibrinolytic drugs such as epsilon‐aminocaproic acid or tranexamic acid infused during surgery and in the immediate postoperative period can reduce overall blood loss. Monitoring for rhythm changes, especially tachyarrhythmias in EA, can be accomplished with a five‐lead ECG and monitoring capable of displaying multiple tracings to facilitate identification of the arrhythmia. ST monitoring is helpful in identifying EA post‐repair right coronary artery compromise which may be identified by ST elevation in leads II, III, or AVF or reciprocal depression in I or AVL. Sometimes, temporary AV pacing may be necessary when complete heart block, junctional rhythms, or sinus node dysfunction present after removal of the aortic cross clamp in order to achieve hemodynamic function sufficient to separate from CPB. Depending upon the etiology of the rhythm disturbance, temporary pacing could be needed for a brief period after cessation of CPB, several hours, several days, or until a permanent pacemaker is placed. Other useful monitoring includes near‐infrared spectroscopy (NIRS) for monitoring brain and somatic oxygenation, especially during the non‐pulsatile flow of CPB. Near the end of CPB, intraoperative transesophageal echocardiography (TEE) is used to assess clearance of air bubbles in the systemic heart chambers, reconstructed TV function, ventricular contractility, and residual shunts in the immediate post‐CPB period. Transcranial Doppler (TCD) flow velocity is not commonly utilized due to the technical difficulty in maintaining its positioning on the patient, but it has the ability to follow changes in cerebral blood flow velocities in unilateral or bilateral anterior and/or middle cerebral arteries during surgery. The TCD can also be used to detect gaseous or particulate emboli that enter the cerebral circulation. Patients with good biventricular function and mild TR can be candidates for immediate extubation at the end of surgery provided that the rhythm is normal sinus or stable in a paced mode and there are no concerns for postoperative surgical bleeding. Inhaled halogenated anesthetics are utilized primarily after CPB, and muscle relaxant and opioids employed sparingly. After the sternum is closed, reversal of muscle relaxation and initiation of pressure‐supported ventilation can begin. Adjunctive IV acetaminophen can be used to decrease opioid requirements at the end of surgery. Additional opioids can be titrated to the respiratory rate as surgical stimulation decreases and inhaled halogenated agents are weaned. Local analgesia administered by the surgery team decreases narcotic requirements at the end of the case. Dexmedetomidine sedation (0.3–0.5 μg/kg/hour) and morphine infusion (25 μg/kg/hour for extubated patients and 30–35 μg/kg/hour for mechanically ventilated patients) are continued upon transfer of the extubated patient to the ICU. High risk patients, such as those who presented with severely dilated right hearts and compressed LVs, are not candidates for extubation in the OR. In addition to higher needs for inotropic support, such patients are at high risk for lethal ventricular arrhythmias in the post‐repair period, especially if they have undergone RV plication. Antiarrhythmic medications such as lidocaine and amiodarone need to remain readily available. Patients who manifest runs of ventricular tachycardia or other ventricular arrhythmias should be placed on amiodarone (3–5 mg/kg over 30 minutes, then 2 mg/kg/day, maximum 20 mg/kg/day or 2.1 g/day) or lidocaine (20–50 μg/kg/min) infusions in the early postoperative period. Prophylactic amiodarone infusions may be considered for patients who present with severe right heart cardiomegaly. Right heart forward flow can be augmented with infusions of milrinone (0.2–0.5 μg/kg/min) and low‐dose epinephrine 0.02–0.04 μg/kg/min. Preload to the right heart must be fastidiously maintained for a poorly functioning RV. In cases with elevated pulmonary vascular resistance, iNO can be instituted. Leaving the sternum open in highest risk patients facilitates access for ECMO in the case of cardiovascular collapse due to arrythmias, low cardiac output, or pulmonary hypertension. In the ICU, continuous monitoring of the heart rhythm and arterial blood pressure is essential. Intravascular volume expansion options need to be readily available. Often, there may be post‐CPB papillary muscle dysfunction, possibly of ischemic etiology. Early ICU echocardiography can show poor coaptation of the TV leaflets, but as the papillary muscle bundle dysfunction resolves, later echocardiographic examinations can show improving TV leaflet coaptation. Pain is controlled with morphine infusions of 25–30 μg/kg/hour for extubated opioid‐naïve patients and 30–40 μg/kg/hour for mechanically ventilated opioid‐naïve patients. Dexmedetomidine 0.3–0.5 μg/kg/hour is used for sedation rather than relying on narcotic infusions for sedation. For the patients with poorly functioning RVs, gradual recovery from sedation is appropriate to allow for recovery of myocardial function prior to increased CO demands of the fully awake patient. Morphine boluses (20 μg/kg) can be added for breakthrough pain, and dexmedetomidine boluses of 0.5 μg/kg over 10 minutes or lorazepam (0.05–0.1 mg/kg IV every 6 hours) can be added for periodic needs of additional sedation, especially for patients who remain intubated and mechanically ventilated. Rhythm disturbances are common in the early post‐repair periods and in some instances extend as a late complication. Operative recovery can be complicated by supraventricular tachycardia, AV block (intermittent or persistent), and junctional rhythm. Temporary pacing enhances CO for patients with AV block or junctional rhythm in the early postoperative period. A return of normal sinus rhythm can result as myocardial edema resolves and cardiac conduction pathways recover. Ventricular arrhythmias and the risk for sudden death is greatest in the first month after repair with those patients who had perioperative ventricular tachycardia or ventricular fibrillation being at enhanced risk [41]. High‐risk patients who have had intermittent ventricular arrhythmias during their early ICU recovery and were treated with IV lidocaine or amiodarone infusions might benefit from continuing oral amiodarone for several months after surgery. TOF is the most common cyanotic congenital heart disease with an estimated incidence of 1 in 2,500–3,000 live births [2]. Although reports of this abnormality can be dated back to 1671, it was Etienne Fallot in 1888 who reported the four cardinal distinguishing features and proposed clinicopathophysiologic correlations derived from extensive clinical and postmortem studies. This “blue malady or cardiac cyanosis” as Fallot referred it, was named “tetralogy of Fallot” in 1924 by Maude Abbott [42]. The first surgical treatment for TOF occurred in 1945, when the first Blalock‐Taussig shunt (BTS) was performed. The tetrad of TOF consists of (Figure 28.12): One embryologic theory states that TOF results from uneven conotruncal spiral septation leading to a smaller MPA and a larger aorta. Another theory, van Praagh’s, suggests that the TOF stems from one abnormality – the underdevelopment of the subpulmonary infundibulum (infundibulum means “funnel” in latin). The hypoplastic infundibulum, in turn, affects the surrounding structures as follows: (i) the conoseptum is deviated anterior and leftward contributing to obstruction to flow, (ii) the aorta is shifted rightward over the ventricular septum facing the VSD and facilitates flow of the ejected RV blood into the systemic circulation, (iii) a VSD results from the malaligned conoseptal tissue failing to join the remainder of the ventricular septum, and (iv) postnatal RV muscle hypertrophy develops from cumulative work against the higher pressure load imposed by a stenotic outflow tract and systemic pressure transmitted across the VSD. The phenotype of TOF with PS varies from patient to patient. Although no two TOF cardiac anatomies are the same, physiologically they follow similar guiding precepts for management. Of note, the normal RV of a newborn infant has similar thickness to the LV because of exposure to systemic pressures. As normal cardiac development progresses, the RV becomes thinner relative to the LV as a result of their markedly different afterloads. Figure 28.12 Schematic illustration of tetralogy of Fallot. (A) Normal heart. (B) Tetralogy of Fallot demonstrating right ventricle infundibular stenosis (Stenosis), ventricular septal defect (VSD), overriding aorta (Ao), and right ventricular hypertrophy (RVH). LA, left atrium; LV, left ventricle; PA, pulmonary artery; RA, right atrium; RV, right ventricle. (Source: Michael L. Schmitz, MD.) In TOF, the RVOTO often occurs at several levels and has fixed and dynamic components. The fixed obstructions occur at the valvar and supravalvar levels. At the valvar level, the pulmonary annulus is frequently hypoplastic with a bicuspid valve that contains dysplastic leaflets. At the supravalvar level, hypoplasia of the MPA can be localized at the sinotubular ridge or be diffuse and extend into the BPAs. The RVOTO dynamic component comes from the hypertrophied subvalvar muscle bundles. The hypertrophy develops over time in response to the increased pressure load. This dynamic component is thought to play a major role during hypercyanotic episodes associated with TOF. The VSD is usually large and unrestrictive. The bundle of His courses at the posteroinferior edge of the defect, near the anteroseptal leaflet of the tricuspid valve, and can be inadvertently injured during VSD closure [43, 44] (Figures 28.12–28.14). Other cardiac anomalies associated with TOF are found in about 40% of patients, and they have implications for the optimal surgical repair strategy [44–56] (Table 28.4). Also, anatomical variations influence surgical conduct, e.g. degree of aortic override and location of conoseptum determine the patch size to close the VSD and baffle the LV flow to the aorta [57]. Unique TOF variants include TOF with PA/VSD/MAPCAS (7%) and TOF with absent PV (5%) [58, 59]: Typically, TOF presents as an isolated lesion, although in 15–30% of patients TOF can present with other abnormalities or as part of a syndrome [60–62]. The most common include the 22q11 microdeletion‐associated syndromes called DiGeorge, velocardiofacial syndrome, and conotruncal anomaly face syndrome. Other conditions include trisomy 21 and VACTERL association, among others [60–71] (Table 28.5). There is a mild association of familial inheritance of TOF. Other genetic bases for TOF have been linked to mutations on NKX2‐5, JAG1, chromosome 20p12, chromosome 5q35, the GATA4 gene on chromosome 8p23, ZFPM2, TBX1, and GATA6 [72]. Figure 28.13 Transthoracic echocardiography images of tetralogy of Fallot. (A) Left image shows ventricular septal defect (VSD), overriding aorta (Ao), and right ventricular hypertrophy (RVH). Right image shows Doppler color flow of left to right shunting through the VSD. (B) Left image reveals anterior deviation of the conal septum with narrowed right ventricular outflow tract (RVOT). Right image Doppler image shows turbulent flow seen through the narrow RVOT. (Source: Destiny F. Chau, MD.) Figure 28.14 Computed tomographic images of tetralogy of Fallot. (A) Sagittal view, demonstrating the overriding aorta (Ao) in brighter contrast. (B) Axial view, showing right ventricular hypertrophy (RVH), pulmonary stenosis (PS), and right pulmonary artery (RPA) hypoplasia. LPA, left pulmonary artery. (Source: Destiny F. Chau, MD.) Table 28.4 Cardiac anomalies associated with tetralogy of Fallot and their implications ASD, atrial septal defect; AV, atrioventricular; CPB, cardiopulmonary bypass; IVC, inferior vena cava; LAD, left anterior descending artery; LSVC, left superior vena cava; PA, pulmonary artery; PDA, patent ductus arteriosus; PFO, patent foramen ovale; RCA, right coronary artery; RV, right ventricle; RVOT, right ventricular outflow tract; TTE, transthoracic echocardiogram; VSD, ventriculoseptal defect. The natural course of uncorrected TOF reveals about 50% mortality in the first 5–10 years of life. Prolonged survival is enabled by the persistence of pulmonary flow via the PDA and /or collaterals, and by compensatory hypoxic mechanisms such as erythrocythemia. Uncorrected, it is rare for survival beyond the 4th decade of life. The morbidity and mortality are secondary to chronic hypoxemic ramifications such as thromboembolism, brain abscesses and endocarditis [73]. Modern operative mortality is low with an overall rate of less than 2% in developed countries [74], and less than 4% in low‐to‐middle income countries [75]. A report analyzing the only collaborative database of congenital heart surgery in low‐to‐middle income countries found that over half of the children undergoing primary TOF repair were over 1 year of age. Interestingly, age at primary repair was not a risk factor for mortality. Poor nutritional status and hypoxemia were associated with increased infection rates and mortality [75]. Athanasiadis et al. found that syndromic patients with TOF had an all‐cause mortality rate of 9.8% [62]. Long‐term survival 40 years after TOF repair has been quoted to be over 70% with late mortality from ventricular fibrillation and heart failure [76]. Table 28.5 Syndromes associated with tetralogy of Fallot AVC, atrioventricular canal; CNS, central nervous system; GI, gastrointestinal; IAA, interrupted aortic arch; PA, pulmonary atresia; TEF, tracheoesophageal fistula; TOF, tetralogy of Fallot; VSD, ventriculoseptal defect. Patients with TOF manifest a wide spectrum of clinical presentations depending on the severity of RVOTO and degree of right‐to‐left shunting of venous blood into the systemic circulation. The unrestrictive VSD allows a near equalization of RV and LV pressures. Shunting direction will be determined by the balance of systemic vascular resistance (SVR) and RVOTO. Most patients are initially asymptomatic and acyanotic – the “pink tets.” Some of them have near‐normal sized pulmonary valvar and supravalvar areas with minimal RVOTO. As PVR drops, the increasing left‐to‐right shunting through the unrestrictive VSD leads to pulmonary overcirculation and even CHF. Cyanosis eventually presents at about 6–12 months when infundibular muscles become hypertrophied and RVOTO increases over time. In contrast, some babies present with severe cyanosis shortly after birth and need immediate medical management for stabilization of PBF while preparing for surgical intervention. In these patients, the main obstruction is usually at the level of the PV. A number of patients develop acute hypercyanotic events or “tet spells,” a sudden and profound hypoxemic event that can result in loss of consciousness, stroke, or death. Typically, hypercyanotic episodes occur in association to periods of increased sympathetic activity such as crying, pain and fright. Other predisposing conditions include dehydration, acidosis, and fever – events which decrease SVR and promote right‐to‐left shunting with resulting arterial desaturation. Additionally, it is postulated that infundibular muscle spasms with acute worsening of RVOTO lead to right‐to‐left shunting and drastic PBF reduction. The resulting acidosis and hypoxemia decrease SVR, furthering the right‐to‐left shunting. Prompt aggressive treatment to halt the vicious cycle, reverse the shunt, and restore pulmonary flow is imperative. Treatment for hypercyanotic episodes is described later in the chapter. During these events, awake patients often hyperventilate, likely to mitigate the hypoxia and acidosis. Squatting behavior during the spell is also observed in older children. Squatting increases SVR from compression of the iliac arteries, increasing RV preload with enhanced venous return generated by elevating intraabdominal pressure and compressing veins in the leg musculature. The result of squatting is reduced right‐to‐left intracardiac shunting and increased PBF. Progression of infundibular and RV hypertrophy from exposure to high pressure loads results in anatomical changes that complicate the perioperative course and contribute to postoperative RV dysfunction: (i) the RV becomes less compliant with abnormal diastolic relaxation, requiring high filling pressures for adequate CO, (ii) the hypertrophied RV and muscle bands increase surgical complexity, (iii) the increased RV muscle mass may reduce the myocardial protection of cardioplegia, a reduction in protection that in some instances is compounded by abnormal coronary artery distributions, and (iv) muscle resection or division is often required to relieve the RVOTO. Presently, complete surgical correction is commonly performed in early infancy for most patients. Early repair is thought to prevent the development of RV hypertrophy, minimize the effects of cyanosis and polycythemia, allow improved pulmonary vascular development via greater PBF, and avoid the occurrence of hypercyanotic episodes. Fetal ultrasonography can diagnose TOF prenatally. After birth, most neonates with TOF are acyanotic and present with non‐specific physical examination findings. Auscultation shows a crescendo–decrescendo systolic murmur best heard at the left upper sternal border prompting further investigation. Clubbing is not a feature at this stage; it only becomes apparent after prolonged periods of chronic hypoxemia. Ductal closure will uncover cyanosis in those with severe PS and ductal‐dependent PBF. The ECG reveals persistent RV hypertrophy and right axis deviation beyond the neonatal period. The chest radiograph shows a “boot‐shaped” cardiac silhouette, resulting from the RV hypertrophy displacing the apex upwards and the hypoplastic MPA creating a concave upper left heart border. Transthoracic echocardiography is effective at establishing the TOF diagnosis and demonstrating related important features: (i) extent and anatomical characteristics of RVOTO, (ii) VSD size, number and locations, (iii) coronary artery distribution, (iv) additional cardiac abnormalities including ASD and aortic arch‐sidedness, and (v) biventricular function. Most children are diagnosed during infancy in countries with routine access to modern medicine. In regions of the world with limited access to care, it is common to see children with uncorrected TOF present at much older ages with advanced pathology. All infants born with TOF eventually require intervention as hypoxemia develops. Most patients can be monitored until meeting indications for surgery that occurs when the oxygen saturation declines below 75–80% or with the advent of hypercyanotic episodes. If delaying surgery is necessary, patients manifesting hypercyanotic episodes can sometimes be temporized with propranolol. For patients with significant cyanosis who have contraindications to surgery, a palliative balloon dilation and/or stenting of the PDA or RVOT can be performed in the cardiac catheterization laboratory [77]. Surgical palliation via a modified BTS (mBTS) is also an option for patients who are deemed too high risk or have unfavorable anatomy to undergo early primary repair. A palliative shunt allows time for optimization of surgical conditions including time for corporal growth; complete repair is facilitated when performed on a larger‐sized heart and infant. This two‐stage repair (palliative systemic‐to‐pulmonary artery shunt followed by later full repair) comes with the risks associated with an additional thoracic surgery as well as risks of loss of shunt patency, imbalance of PBF to systemic blood flow ratio (Qp:Qs), and distortion of pulmonary arterial anatomy. Factors influencing the surgical approach and timing are based on the patient’s symptomatology, cardiac anatomy, concomitant risk factors, the center’s capability and preferences, and contraindications to early complete repair. Elective complete repair in the neonate is currently performed in some centers. The smaller anatomy makes the operation more challenging; many use a ventriculotomy to facilitate surgical exposure as opposed to a transatrial or transpulmonary approach. Additionally, the neonate’s immature organ systems are subject to the negative effects of CPB. Other concerns of neonatal full repair are associated with increased longitudinal morbidity [78, 79]. For elective cases, some centers prefer the staged approach while other centers favor elective complete primary repair between 3 and 6 months of age [78]. Current survival rates after complete repair of TOF are over 98%. Although the optimal TOF repair strategy is unclear, the preservation of the PV and supporting structures while reducing RVOTO are key determinants for reducing long‐term morbidity. Morales et al. have proposed a surgical decision algorithm with the goal of preserving the RV and infundibulum [80, 81] (Figure 28.15). Results for this approach appear promising [81, 82]. Palliation, meaning “lessening the intensity of disease,” comes from the late Latin word “palliatus,” meaning to cloak or to mask. A palliative intervention does not offer correction but rather a relief for a condition, generally only temporary. For TOF, the first operative procedure was palliative, the classic BTS by Blalock in 1945, an end‐to‐side anastomosis of the subclavian artery to the right pulmonary artery branch, to provide a source of pulmonary flow. The anastomosis is usually performed on the side ipsilateral to the innominate artery. Direct anastomotic aorto‐pulmonary shunts, such as the now nearly obsolete Potts and Waterston shunts, are difficult to take down due to their anatomical locations. The mBTS is the most commonly used systemic‐to‐pulmonary shunt (Figure 28.16). It consists of prosthetic graft placed between the innominate artery or the subclavian artery and the ipsilateral BPA. When anatomy is unfavorable for a mBTS, a central shunt, comprising a graft placed between the ascending aorta and the MPA, may be placed. In TOF, the shunt augments PBF until complete primary repair can be achieved. The mBTS allows early palliation without sacrificing the subclavian artery. Resistance from the graft diameter (usually 3.5–4 mm) and length regulates PBF. The locations of the mBTS and central shunts allow exposure and maneuverability during subsequent repairs. A right‐sided mBTS is most common due to more favorable surgical exposure during take down and less risk of phrenic nerve injury compared to a left‐sided mBTS. Although mBTS was traditionally done via a thoracotomy, many centers are performing mBTS via median sternotomy. This approach also facilitates patch augmentation of narrowed BPAs with bypass support. A downside of a mBTS is that the shunt can frequently lead to stenosis and distortion of the BPA [44]. Figure 28.15 Algorithm for treatment of TOF as described by Morales et al. [80]. (Source: Schmitz et al. [38]. Reproduced with permission of John Wiley & Sons.) Figure 28.16 Diagram showing the various types of shunts used to increase pulmonary blood flow. The modified Blalock–Taussig shunt and the central shunt are the only shunts used in the modern era. (Source: Michael L. Schmitz, MD.) Patients who are unsuitable candidates for surgical palliative shunts, often small and critically ill neonates or infants, can undergo the less invasive transcatheter palliative interventions such as pulmonary balloon valvuloplasty and stent placement in the RVOT or PDA to maintain PBF. Candidates for ductal stenting have an anatomically suitable ductus as well as an additional source of PBF. Pulmonary balloon valvuloplasty alone seldom produces long‐lasting improvement, as the dilated RVOT often returns to its original stenotic state. Cutting balloons or primary stent implants are associated with longer‐lasting improvement in relief of obstruction. Application of stents should be done in consultation with the cardiac surgeons as the stents impact the operative conduct of the full repair [52, 83, 84]. See Chapters 19 and 34 for further discussion of RVOT and PDA stenting. Preoperative knowledge of pertinent anatomic TOF details is important for surgical planning and successful repair, including size and nature of RVOTO at the subvalvular, valvular, and supravalvular levels, coronary artery distribution, type and number of VSDs, and any other associated cardiac anomalies. The first successful full repair of TOF was performed on a 10‐year‐old boy by Walton Lillehei in 1954. In the early era of TOF repair, the goals of surgery were relief of RVOTO and VSD closure via ventriculotomy while preserving RV function. In the 1960s, full repair was reserved for children 4–6 years of age because surgical mortality was too high for younger patients. Later, use of deep hypothermic circulatory arrest improved survival and opened the door for full primary repair during infancy. Early repair has hemodynamic and anatomic advantages for the developing heart. Although some centers offer neonatal elective full repair, most centers prefer to perform elective full primary repair between 3 and 6 months of age and prior to 12 months for those with a palliative shunt. The increased duration of longitudinal postoperative follow‐up reveals that adults with repaired TOF exhibit medical and surgical sequelae such as impaired exercise capacity, atrial and ventricular arrhythmias, RV and LV dysfunction, cardiac failure, and sudden death. The pathophysiology of these late complications appears related to the effect of extensive ventriculotomy, coronary artery compromise and PV destruction. Findings from outcomes have led to changes in the surgical approach by emphasizing the preservation of the RVOT apparatus, sparing PV injury, minimizing ventriculotomy incisions, and reducing residual VSDs. Hence, transatrial and transpulmonary approaches with valve‐sparing techniques are emphasized with only selective use of transannular patches (Figure 28.17). The surgical approach is via median sternotomy with CPB‐assist. The thymus size is noted prior to subtotal excision due to association to 22q11.2 microdeletion syndromes with TOF. Upon cardiac exposure, the coronary artery distribution is assessed. After CPB commencement and cardioplegia, the RVOT is examined through a right atriotomy via the TV, and the PV annulus is sized with calibrated dilators. Muscle bundles are divided as needed. A PV commissurotomy can be performed transatrially if exposure is adequate; otherwise, it can be accessed via a transpulmonary approach by a longitudinal incision in the MPA. If preservation of the PV is not feasible, a right ventriculotomy with placement of transannular patch will be done to augment the RVOT size. The VSD is closed using a patch, avoiding the AV node or bundle of His that passes adjacent to the VSD margin. The atrial level shunt is closed if present. If postoperative RV dysfunction is expected, a small atrial communication (“pop‐off”) is left to allow LV filling and preload with a degree of right‐to‐left shunting. Patch augmentation of BPA stenosis is performed as needed. In some patients, a RV‐to‐pulmonary artery conduit may be necessary to avoid injuring a coronary artery crossing the RVOT (Figure 28.18). TEE is used after separation of CPB to assess for adequacy of repair, residual shunts and defects, and ventricular function. An RV:LV pressure ratio greater than 0.7 usually indicates inadequate relief of RVOT obstruction, with the caveat that early post‐CPB pressure measurements do not always correlate to later measurements and might prompt unnecessary revisions. If residual shunts are suspected, a comparison of PaO2 from simultaneous blood gas measurements taken from the superior vena cava and the MPA can be helpful. Temporary atrial and ventricular pacing wires are placed prophylactically for the management of postoperative arrhythmias such as heart block and junctional ectopic tachycardia (JET) following TOF repair. Most patients with repaired TOF will live to adulthood; however, many will require reintervention before 30 years of age to address residual disease. The most common sequela is PI. Other sequelae include BPA stenosis, residual VSDs, and RVOT aneurysmal dilation. Chronic PI leads to RV volume overload, RV dilation and dysfunction, and ventricular arrhythmias. Eventually, these sequalae affect the LV and cause biventricular dysfunction and heart failure. Monitoring for symptomatology and progression of RV dilation is essential. An increasingly used monitoring modality is cardiac MRI for the longitudinal evaluation of volumetric ventricular measurement, PI fraction quantification, and RV functional status [85]. Ideally, a PV replacement is performed prior to irreversible RV dysfunction from the effects of PI but as late as possible to allow placement of an adult‐sized valve to decrease the number of re‐interventions needed in a patient’s lifetime. Bioprosthetic valves deteriorate over time, and most patients will require another replacement within a decade. For symptomatic patients, PV replacement is indicated. For asymptomatic patients, the timing and indications for PV replacement is unclear. Professional organizations have published criteria to guide these decisions [86]. Figure 28.17 Surgically repaired hearts with tetralogy of Fallot, showing resection of right ventricular outflow tract (RVOT) muscle (dashed lines), patch closure of the ventricular septal defect (VSD), and patch arterioplasty: (A) supravalvular main pulmonary patch arterioplasty, RVOT muscle resection, VSD patch; (B) RVOT patch, RVOT muscle resection, VSD patch; (C) supravalvular main pulmonary patch arterioplasty, RVOT patch, RVOT muscle resection, VSD patch. (Source: Michael L. Schmitz, MD.) Figure 28.18 (A) Normal coronary artery pattern, (B) Normal variant conal artery (first branch of right coronary artery) crossing the right ventricular outflow tract, (C) Aberrant left anterior descending artery arising from right coronary artery and crossing the right ventricular outflow tract. (Source: Michael L. Schmitz, MD.) Multiple PV interventions are needed over the lifetime of the TOF patient. The most common late re‐intervention in the patient with repaired TOF is PV replacement. Nonsurgical options for PV replacement are currently available as transcatheter valve implants for patients with dysfunctional RV‐to‐pulmonary artery conduits, such as the Medtronic Melody valve (Medtronic, Inc., Minneapolis, MN), a stent‐mounted bovine jugular valve and the Edwards SAPIEN XT valve (Edwards Lifesciences Corporation, Irvine, CA), a stent‐mounted bovine pericardial valve. Pre‐stenting a RV‐to‐pulmonary artery conduit to prepare a landing zone for the PV to anchor is often necessary (Figure 28.19). Transcutaneous valves for the native RVOT without a circumferential conduit are under development. Knowledge of coronary artery location is necessary to avoid coronary artery compression during and after valve implantation. Other than coronary artery compression, complications include stent fracture, RV‐to‐pulmonary artery conduit tear, device dislodgement and infective endocarditis. This field continues to grow, and more transcutaneous device options will become available [87]. See Chapter 34 for additional discussion of transcatheter PV implantation. Figure 28.19 Transcatheter Pulmonary Valve Implantation. (A) Contrast injection of right ventricle‐to‐pulmonary artery conduit showing pulmonary insufficiency and irregular luminal surface, lateral view. (B) Pre‐stenting of right ventricle‐to‐pulmonary artery conduit for improved landing surface for the pulmonary valve to anchor, lateral view. (C) Pulmonary valve deployment inside of right ventricle–pulmonary artery conduit pre‐stent, lateral view. (D) Contrast injection showing a competent pulmonary valve, lateral view. (E) Final pulmonary valve position, anterior to posterior view. (F) Final pulmonary valve position, lateral view. (Source: Destiny F. Chau, MD.) Hypoxemia becomes progressive over time as PBF diminishes concordant to the increasing obstruction by the RVOT. Some patients experience hypercyanotic episodes when the oxygen saturation acutely drop to 20–30% or less. Since systolic pressure in the RV reflects the pressure in the LV and aorta, a decrease in systemic arterial pressure (as may occur with peripheral vasodilatation caused by anesthetic agents and sedative drugs, or prolonged fasting) results in decreased RV pressure and PBF. An increase in LV pressures, as induced by vasoactive agents and volume administration, can reverse shunting of blood from the RV into the LV and promote PBF. Close monitoring of blood pressure changes from anesthetic interventions can provide early physiological guidance for optimizing PBF and preventing hypercyanotic episodes. Preoperative assessment should include the history of hypercyanotic episodes in patients who are not palliated or repaired. Patients with severe cyanosis present with failure to thrive and polycythemia unless they are anemic. Polycythemia actually enhances the “blueness” during hypercyanotic episodes due to higher levels of deoxygenated hemoglobin. Patients with stable and mild cyanosis often have had generous weight gain from frequent feedings given to prevent agitation and worsened cyanosis. Anticipation, early recognition, and management of potential hypercyanotic episodes are essential for avoiding cardiovascular collapse. Preoperatively, the following factors are considered for preventing hypercyanotic episodes: Even under anesthesia, increased stimulation can incite infundibular spasm that enhances right‐to‐left shunting, precipitating a hypercyanotic episode. Anesthetic induction and times of surgical manipulation are especially vulnerable periods for occurrence of a hypercyanotic episode. Infants with unrepaired TOF may present for anesthetic care for non‐cardiac interventions such as cardiac catheterizations, MRI, and CT, or for surgical procedures that cannot be delayed until cardiac palliation or repair (Table 28.6). The primary goal is to improve PBF so that the hypoxemia can be reversed. PBF is increased by reducing acute RVOT obstruction promoted by the infundibular spasms, enhancing RV preload, and reversing right‐to‐left intracardiac shunting by increasing SVR relative to RV pressure. The objectives are to increase afterload to the left heart, reduce RV infundibular spasm, and augment RV preload (Table 28.7). Table 28.6 Overview of considerations and recommendations for the anesthetic management of patients with unrepaired tetralogy of Fallot (TOF), acyanotic or cyanotic, for non‐cardiac surgery ASA, American Society of Anesthesiologists; CHF, congestive heart failure; ECG, electrocardiogram; IM, intramuscular; IV, intravenous; PBF, pulmonary blood flow; PVR, pulmonary vascular resistance; Qp, pulmonary blood flow; Qs, systemic blood flow; TOF, tetralogy of Fallot; RV, right ventricle; RVOTO, right ventricle outflow tract obstruction; SBE, subacute bacterial endocarditis; SVR, systemic vascular resistance. Table 28.7 Treatment for a “tet spell” or hypercyanotic episode for patients with tetralogy of Fallot RV, right ventricle; IV, intravenous; SVR, systemic vascular resistance; ECMO, extracorporeal membrane oxygenation; CPB, cardiopulmonary bypass. If the patient remains cyanotic despite these measures, ECMO should be considered as a lifesaving therapy. Sternotomy with manual compression of the aorta can be used to temporarily increase SVR and reverse right‐to‐left shunting while rapidly instituting CPB. Most neonates or infants needing surgical palliation are critically ill and dependent on PGE1 infusion to maintain ductal patency for augmenting PBF and managing hypoxemia. Some other infants become symptomatic shortly after discharge from the hospital as they develop cyanosis and fail to thrive. Anesthetic goals are to maintain PBF by limiting right‐to‐left intracardiac shunting and preserving adequate SVR. Induction of anesthesia can be successful with ketamine and/or fentanyl with an inhaled agent for maintenance. General anesthesia and potent inhaled agents have been demonstrated to depress ventricular function [88, 89]. Rivenes et al. showed that both sevoflurane and isoflurane have a dose‐dependent, albeit not statistically significant, decrease in CO and shortening fraction at 1 and 1.5 MAC. Isoflurane led to larger dose‐dependent increases in heart rate and decreases in SVR index compared to sevoflurane; thus, sevoflurane would be the better choice [90]. IV fluids and phenylephrine are useful to increase SVR. Inotropic drugs can increase heart rate and contractility and worsen infundibular spasms. In the absence of reliable IV access, inhalational induction with sevoflurane, and intramuscular ketamine and rocuronium as needed can provide adequate conditions for IV access and tracheal intubation. Central venous access is obtained for the infusion of fluids and vasoactive agents. An arterial line is placed for close hemodynamic monitoring and blood gas sampling. If placing a radial arterial line, it is recommended on the contralateral side of the anticipated mBTS, so that blood pressure assessment is unbiased by the run‐off from the ipsilateral innominate artery or by possible stenosis at the anastomotic site. Although mBTS was traditionally done via a thoracotomy, the mBTS is now often performed via median sternotomy [47]. Median sternotomy permits exposure for conversion to a central shunt, institution of CPB in the event of severe hemodynamic instability or need to patch augment the BPAs, inspection of the coronary anatomy, division of the PDA while confirming adequacy of the shunt flow, and avoidance of a separate incision site after full repair. Median sternotomy also avoids the lung retraction required for exposure with thoracotomy. Heparin at 100 units/kg is given prior to shunt placement. Hypoxemia and hemodynamic instability can occur during the partial clamping for the graft anastomosis and is managed with vasoactive support, fluids, and ventilatory adjustment. Once the shunt is open, oxygen saturation acutely improves while blood pressure decreases. A widened pulse pressure gradient results due to low diastolic pressure secondary to blood run‐off to the lungs; insufficient diastolic blood pressure can compromise coronary perfusion. Management with normoventilation, low FiO2, volume, and vasopressors can temporize this problem. If a mBTS was done via a right thoracotomy and a PDA was present, the excessive diastolic run‐off may continue until the PDA closes. PGE1 infusion is discontinued when alternative PBF is established. A shunt flow approaching Qp:Qs of 1:1 under normal physiological conditions of normocarbia while breathing spontaneously in room air is ideal, resulting in an SpO2 of 80–85% in the absence of parenchymal lung disease. Intraoperatively, simulating normal physiological conditions as closely as possible is important for accurate clinical assessment of Qp:Qs. If the ductus is still open, the additional PBF must be considered in Qp:Qs. Otherwise, saturations too high or too low would indicate an imbalanced Qp:Qs. Lower than expected saturations, after ruling out reversible ventilatory impairments such as atelectasis or endobronchial intubation, may indicate that the shunt is inadequate. Some surgeons prefer the larger diameter shunts if physiologically tolerated to accommodate patient growth and associated increased PBF needs prior to the full repair. Platelet transfusions are avoided whenever possible due to an increased risk of shunt thrombosis. In the ICU, the patient is supported with mechanical ventilation for the next 12–24 hours for stabilization, monitoring and management of ongoing physiologic changes. Potential complications include unilateral or bilateral pulmonary edema or hemorrhage from increased PBF. Also, low systemic diastolic blood pressure can compromise coronary artery flow during diastole, causing myocardial ischemia. Shunt thrombosis is always a potential risk, and heparin infusion at 6–10 units/kg/hour is commenced to maintain shunt patency after the risk of surgical bleeding subsides. A shunt murmur, reflecting presence of shunt blood flow, can be easily auscultated by pausing ventilation and placing the stethoscope at the end of the endotracheal tube; the murmur is transmitted via the endotracheal tube because the shunt is near the bronchus. Antiplatelet therapy is initiated with aspirin when enteral intake is started and continued until the time of complete repair. Other perioperative complications include recurrent laryngeal nerve and phrenic nerve damage and development of chylothorax from thoracic duct injury. The pre‐CPB goal is the promotion of PBF, and post‐CPB goals are preservation of ventricular function and CO optimization. Monitors include central venous pressure and invasive arterial blood pressure. Cerebral oximetry is helpful. TEE is recommended post‐CPB to assess the surgical repair, but epicardial echocardiography can be used if TEE is not feasible. Induction of anesthesia for complete repair can be similarly accomplished as for the palliative shunt placement. For patients at a low risk of hypercyanotic episodes, inhalational induction of anesthesia is generally safe. There are several described techniques for maintenance of anesthesia. If immediate extubation in the operating room or early extubation in the ICU (within 6 hours after surgery) is anticipated, then a multimodal low‐dose opioid technique is recommended. At the author’s institution, a technique of total fentanyl dose of 10–15 μg/kg supplemented with an inhalational agent, dexmedetomidine infusion 0.3–0.5 μg/kg/hour, morphine infusion 20–25 μg/kg/hour, local anesthetic wound infiltration and IV acetaminophen provide for adequate immediate extubation conditions after surgical closure. The extubated patient is transported to the cardiac ICU with continuation of the dexmedetomidine and morphine infusions, as well as a milrinone infusion. A report compared three anesthetic techniques in patients 30 days‐old to 3 years‐old undergoing TOF, AV canal or ASD repair: fentanyl 10 μg/kg vs. fentanyl 25 μg/kg vs. fentanyl 10 μg/kg plus dexmedetomidine at 1 μg/kg loading dose followed by 0.5 μg/kg/hour infusion, until separation from CPB. Adjuvant isoflurane was used in all three groups. It showed that the stress response, coagulopathy, transfusion requirements and postoperative opioid use were highest in the fentanyl 10 μg/kg group while the stress response was most blunted with the 25 μg/kg fentanyl dose. Adding dexmedetomidine to the low‐dose fentanyl group improved stress response blunting and provided better postoperative pain control. No difference in timing for fast track extubation was found amongst all three groups [91]. One institution described using a ketamine infusion and found more hemodynamic stability when compared with isoflurane due to preservation of SVR in the pre‐CPB period [92]. Considerations for separation from CPB include: Indications for returning to CPB include coronary compromise by excessive traction or injury, residual VSDs, residual RVOT obstruction and TR. An STS database report found that early extubation (within 6 hours after surgery) was performed in 31.5% (478 of 1,519) of children undergoing TOF repair between 2010–2013, in an aggregate of 92 centers [102]. An updated report from 2010–2017 found that 17.15% (705/4126,120 programs) of TOF repair patients were extubated before leaving the operating room [103]. Although early tracheal extubation has associated advantages such as avoidance of positive pressure ventilation and shorter lengths of ICU and hospital stay, patient selection and the implementation of strong strategies for managing postextubation pain, sedation, RV dysfunction, and pulmonary status are essential for successful early extubation and good outcomes. Reintubation after extubation failure is associated with increased morbidity, hospital stay and mortality. A factor that might contribute to extubation failure is delirium, a condition that often is treated with additional sedation and sometimes leading to inadequate ventilation [104, 105]. After chest closure, the patient is prepared for planned immediate extubation after meeting extubation criteria. Otherwise, the patient remains intubated and ventilated while receiving adequate sedation during transport to the ICU. Regardless of whether the patient continues mechanical ventilation or is extubated, analgesia and adequate sedation can be provided with morphine (20–40 μg/kg/hour) and dexmedetomidine (0.3–0.7 μg/kg/hour) infusions at titrated doses.
CHAPTER 28
Anesthesia for Right‐Sided Obstructive Lesions
Introduction
Ebstein anomaly
Incidence, anatomy, and natural history
Pathophysiology
Surgical and transcatheter approaches and outcomes
Anesthetic considerations
Preoperative
Intraoperative
Postoperative
Tetralogy of Fallot
Incidence, anatomy, and natural history
Description
Frequency
Surgical Significance
Other Considerations
Coronary artery anomalies
Anomalous and variant coronary artery anatomy can limit options for RVOT patch placement
4.3% anomalous coronary artery (usually the LAD),
6% have a variant infundibular artery originating from RCA crossing the RVOT
(Total 10.3%)
Presence of coronary artery over RVOT limits options for RVOT patch and may require RV to PA conduit placement
Direct visual inspection prior to RVOT incision. TTE is usually an adequate primary survey. CT scan can be definitive.
Pulmonary Valve
Frequently bicuspid with thickened leaflets tethered to the pulmonary artery wall
Over 50% are bicuspid
Pulmonary surgical valvotomy for relief of stenosis
Usually, valve will re‐stenose after balloon valvuloplasty
Multiple VSDs
Can occur in multiple locations, generally in the muscular septum
5–14%
Suture placement can damage the conduction system. When multiple VSDs are present some may be missed, leading to repeat CPB run to close hemodynamically significant VSDs.
Residual VSDs can result in continued postoperative pulmonary overcirculation.
Most muscular VSDs close spontaneously.
Discontinuous Pulmonary Arteries
The proximal LPA near the site of ductal insertion is a frequent location for stenosis. The LPA has ductal tissue that, once regressed, can lead to stenosis or discontinuity of LPA.
Restoration of continuity and/or augmentation of the branch pulmonary arteries is required.
ASD/PFO
Potential for postoperative atrial level shunting if not closed surgically
Almost always PFO or ASD is present
Often a small opening is left if anticipating postoperative RV dysfunction.
Occasionally included as the pentalogy of Fallot
Right Aortic Arch
Often present with associated abnormal branching of head and neck vessels
25%
Placement of palliative shunts depend on spatial location of aortic branches to pulmonary artery
Others
All might require modifications to the surgical repair, such as an additional venous drainage cannula for a LSVC
*Nearly 1 of 4 of patients with syndromic TOF undergo palliation prior to full repair
Syndrome
Frequency
Associated findings
22q11.2 microdeletion, also known as DiGeorge syndrome, velocardiofacial syndrome and others
7.4–10.5% of all TOF patients
or
40–47.8% of the syndromic TOF patients
Trisomies: 21, 18, 13
Trisomy 21
Trisomy 18
Trisomy 13
VACTERL association
2.6% of the syndromic TOF patients
CHARGE Syndrome
0.9% of all TOF patients
Alagille Syndrome
0.4–1.3% of all TOF patients
Holt‐Oram syndrome
0.2% of the syndromic TOF patients
Pathophysiology
Surgical and transcatheter approaches and outcomes
Initial stabilization and planning for intervention
Surgical and transcatheter palliation
Surgical repair
Long‐term surgical complications
Transcatheter pulmonary valve implantation
Anesthetic considerations
Initial assessment and planning
Management of hypercyanotic episodes
Anesthesia considerations and recommendations for patients with unrepaired TOF undergoing non‐cardiac surgery
Acyanotic, “Pink Tets,” at risk for pulmonary overcirculation
Cyanotic, “Blue Tets,” at risk for hypercyanotic spells
Hemodynamic goal:
Maintain Qp:Qs near 1:1
Hemodynamic goal:
Preserve PBF and avoid hypercyanotic episodes
FiO 2 restrictions‐ Avoid unnecessary hyperoxia as it increases PBF
FiO 2 restrictions‐ None
PREOPERATIVE
PREOPERATIVE
INTRAOPERATIVE
Monitors/Lines
Anesthesia induction
**SBE prophylaxis as indicated
Anesthesia maintenance
Anesthesia emergence
INTRAOPERATIVE
Monitors/Lines
Anesthesia induction
**SBE prophylaxis as indicated
Anesthesia maintenance
Anesthesia emergence
Postoperative care
Postoperative care
Perioperative anesthetic management for surgical palliation
Postoperative care for surgical palliation
Perioperative anesthetic management for surgical repair
Postoperative care for surgical repair
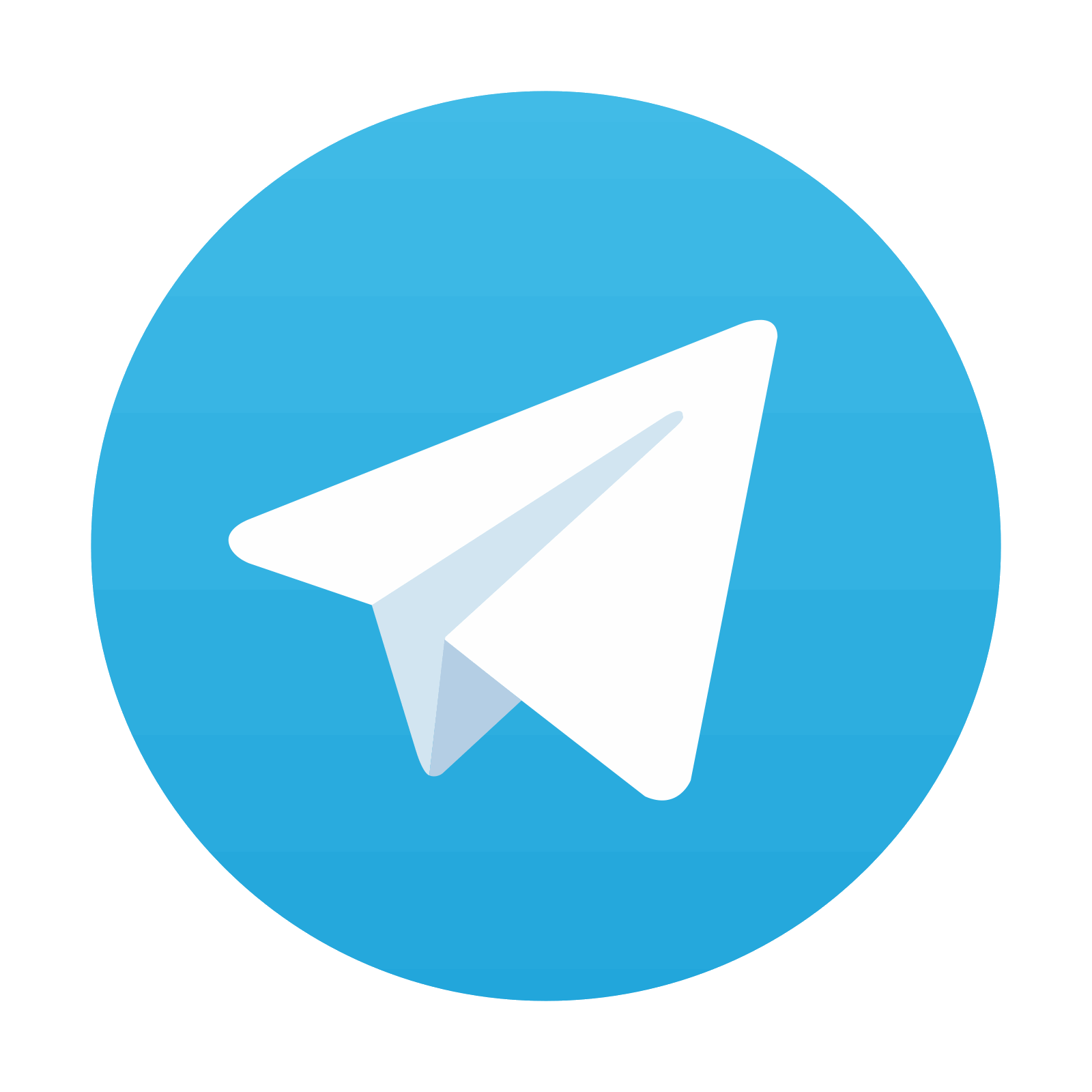
Stay updated, free articles. Join our Telegram channel
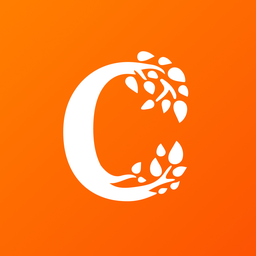
Full access? Get Clinical Tree
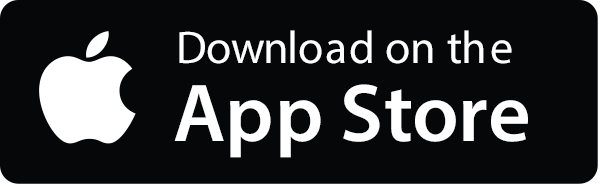
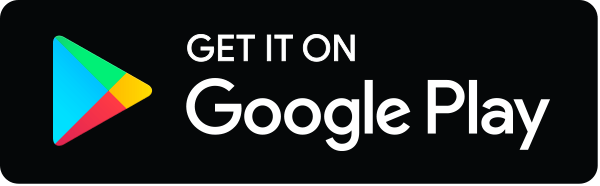