James P. Spaeth1 and Andreas W. Loepke2 1 Division of Cardiac Anesthesia, Cincinnati Children’s Hospital Medical Center, Cincinnati, OH, USA 2 Cardiac Anesthesiology, Children’s Hospital of Philadelphia, Philadelphia, PA, USA Left‐sided obstructive lesions of the heart can occur at various anatomic levels and with varying degrees of severity, ranging from a bicuspid aortic valve with minimal hemodynamic compromise to aortic atresia and hypoplastic left heart syndrome (HLHS) with profound hemodynamic derangements. Imbalances in systemic blood flow may occur due to a circumscribed obstruction at a single level, such as in hypertrophic cardiomyopathy; coarctation of the aorta; interrupted aortic arch; and subvalvular, valvular, or supravalvular aortic stenosis, or from obstructions at multiple levels, such as seen in patients with Shone’s complex. Depending on the specific type of left‐sided obstruction, the child may also be at risk for pulmonary hypertension and/or impaired coronary perfusion during anesthetic care. A clear understanding of the level and degree of the obstruction is therefore important for optimal anesthetic management. A normal aortic valve has three leaflets and occupies an area of around 2 cm2/m2 of body surface area. Although by some estimates, isolated congenital valvular aortic stenosis occurs in only approximately 2 per 10,000 births [1], it is associated with other cardiac lesions in more than 5% of children suffering from congenital heart disease (CHD) and presents with three to four times higher prevalence in males [2]. Valvular aortic stenosis may be associated with aortic coarctation and/or hypoplasia of the ascending and transverse arch, due to decreased antegrade blood flow in the fetus, which prevents adequate development of these structures. Aortic stenosis (AS) has been described as part of Hunter’s, Hurler’s, and Turner’s syndromes. Structural variations from the customary tricuspid contour of the aortic valve are common and result in a bicuspid or monocuspid appearance of the valvular apparatus, whose formation starts during weeks 5–7 of embryonic development [3, 4]. The diseased valve leaflets are gelatinously or myxomatously malformed, and the commissures are often partially fused, leaving a diminished aortic orifice, which can be located eccentrically. Left ventricular myocardial hypertrophy is commonly observed, but non‐hypertrophied left ventricular dilation has also been reported [5]. The age of diagnosis, clinical presentation, treatment modality, and timing of intervention depend not only on the degree of valvular obstruction but also on concomitant cardiac lesions and left ventricular size and function. Only a small minority (approximately 2%) of asymptomatic patients will develop aortic valve dysfunction by adolescence, and progression of the lesion is usually slow [6, 7]. Conversely, neonates with ductal‐dependent critical AS may present in shock, requiring aggressive resuscitation and emergent intervention. The diagnosis is typically made using transthoracic echocardiography (TTE), which provides comprehensive information regarding valve anatomy, annular size, aortic root diameter, and ventricular function (Table 27.1) [8, 9]. Continuous‐wave mean Doppler gradients adequately predict the peak‐to‐peak transvalvular gradient as measured during cardiac catheterization, whereas peak instantaneous Doppler gradients slightly overestimate the transvalvular gradient [10, 11]. However, correcting the peak Doppler gradient for pressure recovery, a phenomenon describing the kinetic energy being transformed back to pressure energy as the velocity decreases distal to the stenosis, significantly improves agreement with subsequently measured catheter gradients [12]. Depressed left ventricular function, however, can lead to a misleadingly low gradient. In the most severe form of critical AS, presenting in the neonatal period, systemic blood flow and coronary perfusion can often be dependent on retrograde blood flow via a patent ductus arteriosus (PDA). After spontaneous ductal closure, the affected neonates frequently present with symptoms of significant congestive heart failure, metabolic acidosis, and mitral valve insufficiency, quickly progressing to cardiogenic shock. Aggressive hemodynamic, respiratory, and metabolic resuscitation as well as immediate treatment with prostaglandin E1 are essential to re‐establish adequate systemic perfusion. In infants with a less dramatic presentation, left ventricular stroke volume is often maintained for extended periods of time. Normal ventricular wall tension is maintained by concentric ventricular hypertrophy; however, increased left ventricular systolic and end‐diastolic pressures can result in impaired subendocardial perfusion and ischemia. In severe cases, this malperfusion leads to the replacement of the myocardium with a fibrous tissue, termed endocardial fibroelastosis, further impairing ventricular performance and diminishing survival [13–15]. Table 27.1 Grading of aortic stenosis severity Treatment options and timing for intervention depend on the acuity of the clinical presentation and the concomitant cardiac abnormalities, as valvular aortic stenosis can cover a wide anatomical and pathophysiological spectrum. Patients with mild AS can be followed conservatively, and if aortic valve repairs or replacements are performed, outcomes are generally excellent [16], especially if achieved without patches [17]. Neonates suffering from critical AS, on the other hand, require immediate intervention. In this population, important consideration is given to the size and adequacy of the left ventricle to tolerate a one‐ or two‐ventricle repair [18]. In order to predict a successful outcome with either biventricular or univentricular palliation (the latter is discussed in Chapter 30) in neonates with critical AS, several scoring systems have been developed (Table 27.2) [19]. Unfortunately, a recent single‐center, retrospective study in 68 neonates did not find any of the binary score cutoffs to be predictive of successful biventricular vs. univentricular repair. In particular, the Rhodes and Discriminant scores predicted the need for a univentricular approach in most patients who successfully maintained biventricular circulation after neonatal aortic valve intervention. Table 27.2 Predictive scores for successful biventricular repair of critical aortic stenosis (Source: Sachdeva et al. [19]. Reproduced with permission from Cambridge University Press.) BSA, body surface area; CHSS, Congenital Heart Surgeons’ Society; LV, left ventricular; LVOT, left ventricular outflow tract; 4Ch, four‐chamber; PSLA, parasternal long axis; BiV: biventricular; EFE: endocardial fibroelastosis; 2 V, two‐ventricle. Given an adequate left ventricular size, the majority of pediatric cardiac centers currently favor percutaneous balloon valvuloplasty as the first treatment option for severe, ductal‐dependent congenital AS. Using a retrograde approach, the balloon sheath is most commonly advanced from the femoral, umbilical, or carotid arteries. However, femoral vascular complications are not uncommon using this technique [20]. An alternative, antegrade approach can be used, in which the balloon is usually inserted from the femoral or umbilical veins, via the foramen ovale, into the left atrium and ventricle. If left ventricular function is adequate, administration of a bolus of adenosine (0.3–0.5 mg/kg), rapid right ventricular overdrive pacing (rate of 220–240/min), or an infusion of esmolol (200 μg/kg bolus followed by titrated infusion) during balloon dilation prevents forceful ventricular contraction against the balloon‐occluded left ventricular outflow tract (LVOT) and ejection of the balloon from the aortic position with potential injury to the valve apparatus. In order to avoid post‐interventional aortic valve insufficiency, the selected balloon size usually should not exceed the diameter of the aortic root. Using these techniques, severe aortic valve insufficiency can be avoided in greater than 80% of patients immediately after intervention, and the 5‐year mortality typically remains below 20% [20–24]. If the left ventricle is of borderline size, significant aortic insufficiency would disqualify patients from single‐ventricle palliation. See Chapter 34 for further discussion of percutaneous balloon aortic valvuloplasty. Surgical options include primary surgical valvotomy, valvular repair, or replacement and depend on severity of the lesion and patient age. Advantages of an open approach include direct inspection and commissurotomy of the valve and the ability to address concomitant cardiac lesions such as PDA, ventricular septal defect (VSD), or aortic coarctation during the same procedure. However, the need for cardiopulmonary bypass (CPB) can increase morbidity for the patient. Historical case series using this approach have demonstrated an operative mortality of 0–18%, 5‐year survival of 60–100%, aortic valve competency in greater than 95% of patients, and a 10‐year freedom from reintervention of greater than 70% following surgical valvuloplasty [22, 25–28]. The Ross procedure, an aortic valve replacement with a pulmonary autograft combined with placement of a homograft in the pulmonary position, was introduced by Donald Ross in 1967 (Figure 27.1) [30]. The autograft can also be supported on the outside by a tube graft [31]. It can also be combined with enlargement of the LVOT, which is termed as the Ross–Konno procedure [29]. These procedures can be performed in neonates and infants, but have demonstrated better outcomes when performed in childhood or adolescence. Compared with prosthetic valve replacement, the Ross procedure uses autologous tissue and avoids the need for chronic anticoagulation. Long‐term outcomes have been favorable, with greater than 75% freedom from reoperation up to 10 years postoperatively [32–35]. Allograft or prosthetic valve replacement is usually reserved for adolescent patients after completion of much of their somatic growth, if they are candidates for chronic anticoagulation. More recently, reconstruction of the aortic valve with pericardial tissue has been described by Ozaki and colleagues [36] (Figure 27.2). The diseased aortic leaflets are excised and replaced with three pieces of glutaraldehyde‐treated autologous pericardium trimmed to the size of the original leaflets, which are then attached to each annulus. Initial long and medium‐term results in adults and children have been encouraging [37–39]. Table 27.3 presents the outcomes after aortic valve surgery in children. During the past two decades, transcatheter aortic valve replacement (TAVR) has evolved from compassionate use in high‐risk inoperable patients to an alternative modality to surgical valve replacement, even in lower risk patients [41]. A recent review in adult patients suggested a trend toward favoring a transcatheter over the surgical approach for aortic valve replacement, with a concomitant decline of in‐hospital mortality for TAVR. TAVR has also been utilized in pediatric patients down to 4 years of age who were suboptimal surgical candidates; however, data on valve longevity is not yet available [42]. Experimental treatment options for critical AS or aortic atresia have included fetal transcatheter valvuloplasty [43]. The rationale for intervention, despite the risks for both mother and fetus, is to avoid single‐ventricle palliation by preventing the natural progression of severe AS to HLHS. The treatment assumes that alleviating the restricted blood flow across the aortic valve will lead to improved growth and function of the left ventricle, thereby allowing a postnatal biventricular repair. However, fetal interventions for AS remain highly controversial and long‐term outcomes are unclear [44, 45]. See Chapter 19 for further discussion of emerging treatment options. Neonates with critical AS can present with significant metabolic derangement and hemodynamic instability. Anesthetic management is directed toward meeting the increased oxygen requirement of the hypertrophied left ventricular myocardium and optimizing cardiac output. Physiologic goals include maintenance of preload, afterload, and contractility. A normal or, to some extent, decreased heart rate is preferred. Bradycardia, however, is not well tolerated in neonates, whose cardiac output is heart rate‐dependent given their fixed stroke volume, even without AS. Anesthetics that decrease systemic vascular resistance and/or increase heart rate must be used with caution to avoid myocardial ischemia and rapid deterioration of the patient’s status. Vasoconstrictors such as phenylephrine can be used in this clinical scenario. Dysrhythmias are not uncommon during cardiac catheterization and should be treated aggressively. Inotropic support with a medication such as epinephrine may be required if myocardial function becomes depressed, but must be used with caution given its ability to increase heart rate and myocardial oxygen requirement. In unstable patients, a high‐dose opioid technique will facilitate both balloon valvuloplasty and surgical valvotomy. Occasionally, extracorporeal membrane oxygenation backup is arranged for selected high‐risk patients. Figure 27.1 The Ross procedure (pulmonary autograft). (A) Incisions on the aorta, pulmonary artery, and the coronary buttons. (B) Pulmonary autograft and reimplantation of the coronary arteries. (C) Completing operation with pulmonary artery conduit to replace the native pulmonary valve. (Source: Mavroudis et al. [29]. Reproduced with permission from Cambridge University Press.) Subvalvular AS (subAS) is a fixed obstruction occurring within the LVOT and accounts for approximately 1% of patients with CHD. Patients with subAS usually become symptomatic after infancy, with a higher male predilection of 2:1 to 3:1 [46]. Most commonly, the anatomic correlate of the LVOT obstruction is either a thin, discrete membrane of endocardial or fibrous tissue or a fibromuscular ridge emanating from the crest of the interventricular septum. Less common manifestations include a circumferential, fibromuscular ring that originates from the anterior mitral valve leaflet and a diffuse, tunnel‐like fibromuscular obstruction. Other rare causes of subAS include anomalous attachments of mitral valve leaflets or mitral chordae. A variety of congenital heart lesions may exist in association with subAS and include bicuspid valves, AS, VSD, and aortic coarctation (Table 27.4). SubAS can occasionally present in infancy as part of Shone’s complex or following the surgical closure of a VSD, but more commonly, the diagnosis is not made before the end of the first year of life and severity usually increases with age. Figure 27.2 The Ozaki procedure. View of the coaptation of the three new aortic valve leaflets constructed using autologous pericardium. (Source: Ozaki et al. [36]. Reproduced with permission from Oxford University Press.) Table 27.3 Outcomes after aortic valve surgery in children Source: Buratto et al. [40]. Reproduced with permission from Elsevier. Table 27.4 Concurrent congenital anomalies in patients with subvalvular aortic stenosis Patients usually present with complaints of orthopnea, dyspnea on exertion, or exertional angina and syncope. A systolic ejection murmur is present, most notable in the left, second, and third parasternal spaces, and a carotid artery thrill can be palpated in a significant number of patients. The diagnosis is confirmed by color Doppler echocardiography, which allows assessment of the degree of stenosis, biventricular enlargement and function, and concomitant mitral and aortic regurgitation. Cardiac catheterization is usually only needed to assess patients with serial obstructions or a tunnel‐like stenosis. As the severity of subAS increases with age, progression of the LVOT obstruction may eventually lead to left ventricular diastolic dysfunction and pulmonary venous hypertension. Moreover, abnormal blood flow across the aortic valve can lead to thickening of the valve leaflets, causing valvular AS, left ventricular hypertrophy, damage to the aortic valve, and subsequent aortic insufficiency with progressive regurgitation. A higher LVOT gradient at diagnosis is an independent predictor for these adverse outcomes. Currently, surgery is favored as an early intervention for LVOT peak pressure gradients exceeding 40 mmHg and consists of fibromuscular resection with or without myectomy through an aortotomy (Figure 27.3), but timing remains controversial. A study comparing early and late surgery in 83 patients with subAS found that the LVOT gradient was successfully reduced in both groups, but that the late surgery group had a much higher recurrence rate at 5 and 10 years (28 and 57%, respectively) compared with the early surgery group (6 and 0%, respectively) [47]. Failure to intervene early also increases the risk of developing aortic regurgitation, which does not necessarily improve following subaortic resection. Transcutaneous balloon dilation is usually ineffective due to only temporary relief of the stenosis. Surgical complications include newly developed or worsened aortic insufficiency and postoperative atrioventricular heart block. In patients with tunnel‐type subAS, an aortoventriculoplasty (Konno procedure) may be required or in the presence of aortic insufficiency, an aortic root replacement with a prosthetic valve or a Ross–Konno procedure is now favored. The Konno operation is still favored in patients with very small aortic annulus and involves an aortic incision above the aortic commissures and extended to the annulus and carried through the left and right ventricular outflow tracts, placement of a mechanical or bioprosthetic stented aortic valve, followed by closure with a pericardial or synthetic patch. Compared with the Konno operation, the Ross–Konno procedure requires less of an incision into the interventricular septum, limits right ventricular outflow tract distortion and potential injury to the conduction system (see also section on Shone’s Complex later in this chapter). The operation involves harvesting the pulmonary valvular autograft, resection of the diseased aortic valve with coronary button mobilization, implantation of the pulmonary autograft and neoaortic reconstruction with coronary button anastomoses, followed by implantation of a pulmonary homograft into the right ventricular outflow tract. The use of an autograft provides growth potential and freedom from anticoagulation regimens [29]. Figure 27.3 Surgical repair of subaortic stenosis. (Source: Adapted from Tworetzky et al. [43].) Anesthetic management of subvalvular stenosis aims at maintaining the oxygen requirements of the myocardium and other end organs. Accordingly, the goal is to decrease myocardial oxygen demand and preserve both preload and afterload while maintaining or allowing a slightly decreased heart rate. In this regard, the anesthetic management of the patient with subAS is very similar to that for valvular AS. Congenital supravalvular AS is the least common fixed obstruction of the LVOT, accounting for less than 0.05% of CHD. However, caused by abnormalities in the elastin gene (ELN), supravalvular AS occurs in up to two‐thirds of patients with Williams–Beuren syndrome, also known as Williams syndrome, and as part of familial supravalvular AS [48–51]. The outflow tract obstruction frequently arises as a concentric narrowing of the ascending aorta at the superior margin of the sinuses of Valsalva, creating a typical hourglass deformity of the aorta [52]. Other manifestations include a diffuse narrowing along the entire ascending aorta or a fibrous semicircular membrane at the sinotubular junction. Supravalvular AS is a progressive disease, usually diagnosed after infancy, but may present earlier in life in patients with Williams syndrome. Coronary artery involvement, which is common in children with Williams syndrome, may include a reduction of the left coronary artery ostial size, ostial obstruction by fusion of the aortic cusp to the supravalvular ridge, or diffuse narrowing of the left coronary artery (Figure 27.4). In cases of mild supravalvular AS, one case series found spontaneous improvement in the obstruction with time in 16% of patients and complete resolution in another 12% [53]. Figure 27.4 Representative angiogram of a patient with Williams syndrome, demonstrating supravalvular aortic stenosis (black arrow) and left coronary artery ostial stenosis (white arrow). (A) Anteroposterior view. (B) Lateral view. Peak systolic gradient during catheterization was 70 mmHg. (Source: Robert Beekman, MD. Reproduced with permission.) The underlying mechanism for supravalvular AS in Williams syndrome is an elastin arteriopathy, leading to a lack of elastic tissue in the walls of large arteries, increased amounts of collagen, and hypertrophy of smooth muscle cells [54]. Accordingly, pulmonary artery stenosis is also observed in over 30% of these patients, which can lead to biventricular hypertrophy [50]. Supravalvular AS may also be associated with a bicuspid aortic valve and aortic valvular stenosis in up to 50% of patients [55]. Concentric left ventricular hypertrophy can lead to myocardial ischemia, which is exacerbated by concomitant coronary artery stenosis. The risk of sudden death may only be slightly raised in patients with sporadic supravalvular AS, but is significantly increased in patients suffering from Williams syndrome, compared with the general population [56, 57]. A low‐pitched, crescendo–decrescendo, systolic murmur is commonly heard at the base of the heart, radiating to the right carotid artery. The diagnosis of supravalvular AS is routinely made using two‐dimensional echocardiography, and Doppler echocardiography can be utilized to determine the pressure gradient across the ascending aorta. Electrocardiograms usually show left ventricular hypertrophy with special attention given to potential ischemic ECG changes. Patients with Williams syndrome, occurring in approximately 1 in 20,000 live births, are easily recognized due to their characteristic facial dysmorphisms; common features include a wide mouth with full lips, micrognathia, dental malocclusion, widely spaced teeth, a short nose with a flat nasal bridge, and a long philtrum. They may also suffer from arterial hypertension, hypercalcemia with nephrolithiasis, esotropia, a hoarse voice, joint hyperelasticity, and sensorineural hearing loss. Although patients often exhibit cognitive impairment, they commonly have a characteristic desire for conversation and company with a lack of social inhibition [50, 58]. The suspected diagnosis of Williams syndrome can be verified even in neonates who do not yet exhibit the characteristic features, using genetic testing for karyotype and the fluorescent in situ hybridization (FISH) test for the 7q11.23 elastin gene deletion [54]. See Chapter 8 for futher discussion of Williams Syndrome. Infants with a peak pressure gradient of less than 20 mmHg across the supravalvular stenosis often remain stable and do not require intervention; however, a peak instantaneous pressure gradient of greater than 75 mmHg is usually an indication for surgical intervention [40]. Surgical techniques for repair of supravalvular stenosis include patch aortoplasty with one (Figure 27.5) or multiple patches [59, 60], complete excision of the stenotic ring with end‐to‐end anastomosis, or the Ross or Ross–Konno procedures [55, 61]. Significant coronary ostial obstruction may be relieved by a patch enlargement of the coronary os, excision of the obstructing aortic leaflet, or coronary artery bypass grafting [62]. Concomitant pulmonary artery stenosis in many patients with Williams syndrome is frequently addressed by balloon dilation of the right ventricular outflow tract stenosis prior to surgical repair of the LVOT obstruction. Figure 27.5 Repair of supravalvular aortic stenosis. (A) External appearance. (B) Coronal plane view of the defect. (C) Inverted Y incision in the ascending aorta. (D) Placement of an autologous pericardial patch. (Source: Chang et al. [59]. Reproduced with permission from Wolters Kluwer Health, Inc.) Supravalvular AS shares many of the pathophysiological characteristics and anesthetic management requirements of valvular and subvalvular stenosis. However, due to the potential combination of supravalvular AS, left ventricular hypertrophy, right ventricular outflow tract obstruction, and coronary artery disease, patients with Williams syndrome can be at significantly increased risk for anesthesia‐related complications. Accordingly, several reports have described perioperative fatalities in these patients, ranging from events during anesthesia induction or intubation to postoperative complications [56, 63–67]. The majority of these reported events seemed to be caused by myocardial ischemia. Maintaining afterload at slightly elevated levels is therefore paramount to preserve coronary perfusion pressure. Volatile anesthetics must be used with caution due to their myocardial depressive and vasodilatory effects. Intramuscular premedication with ketamine may facilitate the establishment of intravenous access, and high‐dose opioid techniques may accomplish the anesthetic goals. However, significant increases in heart rate, which can occur following administration of ketamine or vagolytic agents, are not well tolerated and need to be treated aggressively. Also, arrhythmias, such as those caused by catheter manipulation during cardiac catheterizations, are frequently poorly tolerated. It must be emphasized that the coronary arteries are frequently significantly involved, being partially obstructed or “hooded” from the abnormal surrounding connective tissue in the aorta, and the degree of coronary obstruction often does not correlate with the severity of the supravalvular AS. Other anesthetic complications, such as difficult mask ventilation or difficult tracheal intubation, may arise due to the concomitant facial deformities in this syndrome. Moreover, the potential development of abnormal skeletal muscle tissue with lipid deposits may lead to an increased sensitivity to muscle relaxants, thus warranting close monitoring of neuromuscular blockade [68]. Additional sources of perioperative morbidity include their predisposition to renal insufficiency and arterial hypertension, which need to be appropriately addressed in the anesthetic plan. Preoperative risk assessment is difficult, because adequacy of coronary blood flow is difficult to verify and the more reliably quantifiable degree of supraaortic stenosis does not necessarily correlate with the degree of coronary abnormalities. In particularly, for critical patients, extracorporeal membrane oxygenation (ECMO) or CPB backup may be advised as cardiopulmonary rescuscitation can be challenging in these patients. Hypertrophic cardiomyopathy (HCM) is a cardiac disorder with significant heterogeneity of etiology, anatomic findings, and clinical presentation; in adults, it occurs with an estimated prevalence of 1:500 [69, 70]. This disease is diagnosed less commonly in children, but when identified, it carries a significant risk for adverse outcomes, with an overall survival of 97% at 5 years and 94% at 10 years [71]. Over the past decade, the increased availability of genetic and metabolic testing has improved the ability to identify the underlying etiology of HCM. While in both adults and children, the most common cause of HCM is a mutation in sarcomeric genes, in the pediatric population, the etiology is more heterogeneous and non‐sarcomeric causes represent up to 35% of cases of HCM [72] (Table 27.5). In one single‐center pediatric study, an affected family member, positive HCM gene panel mutation, metabolic disease, or genetic syndrome were identified in over 80% of patients with HCM. [61] Children presenting with HCM at less than 1 year of age carry a worse prognosis, and an inherited error of metabolism or malformation syndrome (e.g., RASopathies) is likely present [72, 73]. Genetics evaluation and testing is now recommended in all children diagnosed with HCM [71]. The characteristic gross morphologic feature in HCM is a hypertrophied and non‐dilated left ventricle, and the absence of other disease processes capable of causing this degree of hypertrophy (e.g., AS). There is significant heterogeneity with regard to the location and degree of cardiac hypertrophy, and in fact, even first‐degree relatives with familial HCM often exhibit different patterns of hypertrophy [74, 75]. Myocardial hypertrophy may occur in the anterior, posterior, and/or basal regions of the ventricular septum, and in the ventricular free wall. The hemodynamic severity is dependent on the particular region where hypertrophy is most prominent: significant hypertrophy of the anterior region of the ventricular septum compared with the posterior septum more commonly causes subaortic outflow obstruction [76]. Echocardiography is extremely helpful in determining the extent, location, and severity of the disease. Quantitative echocardiographic findings in patients with HCM include increased left ventricular wall thickness, decreased left ventricular end‐diastolic cavity size, and increased left ventricular fractional shortening [77]. Other important findings include the presence and severity of LVOT obstruction, systolic anterior motion of the mitral valve, and mitral regurgitation [78]. Cardiac Magnetic Resonance Imaging (CMR) is also commonly used for diagnosis, risk prediction, and pre‐surgical planning. This imaging modality provides extremely accurate information regarding LV wall thickness, ventricular chamber sizes, and the degree of myocardial fibrosis which has been shown to be a marker for increased risk for ventricular tachyarrhythmias and heart failure progression [79]. Table 27.5 Non‐sarcomeric causes of hypertrophic cardiomyopathy in children Source: Monda et al. [72] / Frontiers Media S.A. / CC BY 4.0. The natural history of this disease is dependent on multiple factors, including the underlying etiology, age at presentation, clinical signs and symptoms, and the presence of other co‐morbidities. Most patients with HCM, and neonates in particular, often remain asymptomatic for a long period of time. Many patients are not diagnosed until they present with symptoms of congestive heart failure, cardiac rhythm disturbances, or syncope or following a near‐death cardiac event [70]. HCM has become the leading cause of sudden cardiac death in young athletes [74, 75]. Even when the diagnosis is made prior to a catastrophic event, the clinical course of the disease is inconsistent and dependent on whether the HCM is obstructive or non‐obstructive. If obstructive, more than 25% of infants will develop symptoms of congestive heart failure manifested by feeding intolerance or failure to thrive [80]. Hypertrophic cardiomyopathy is most commonly caused by mutations in genes encoding proteins of the myocardial sarcomere, and in fact over 50 mutations causing the disease have been identified, making it one of the most widespread genetic diseases of the myocardium. Depending on the specific mutation, abnormalities in the protein may lead to ineffective contraction of the sarcomere and the development of myocyte hypertrophy [70]. The histology of the left ventricle is remarkable for disarray of the hypertrophied cardiac muscle cells, myocardial scarring and fibrosis, and thickened walls in small intramural coronary arteries causing luminal narrowing [76, 81]. Although fiber disarray is present even in normal hearts, the extent to which this happens in the heart with HCM is significantly increased [82]. A minority of patients may progress to a secondary phase characterized by wall thinning in areas of previous hypertrophy, enlargement of cavity size, and impairment of ventricular function, a phenomenon likely related to myocardial ischemia and necrosis [76]. Hypertrophic cardiomyopathy also presents in infancy without sarcomeric protein gene mutations, such as in infants of insulin‐dependent diabetic mothers, children with malformation syndromes such as Noonan syndrome, or in patients with glycogen storage diseases such as Pompe disease. In Noonan syndrome, a genetic disorder commonly associated with congenital cardiac disease, approximately 15–20% of children have HCM, with less favorable outcomes than children with non‐syndromic HCM [80, 83]. Pompe disease represents a glycogen storage disease type II (GSD‐II) which is a rare autosomal recessive disorder occurring in 1 out of 40,000 live births, in which lysosomal glycogen accumulates in both cardiac and skeletal muscle due to a deficiency of acid α‐glucosidase [84, 85]. Children presenting with the infantile form of this disease exhibit severe HCM, and, if untreated, often die in the first year of life from respiratory or cardiac complications. Therapy with long‐term intravenous recombinant α‐glucosidase often leads to significant resolution of the cardiac hypertrophy and skeletal muscle weakness [86] Infants presenting for surgery prior to therapy with recombinant human α‐glucosidase are at a high risk for anesthetic complications [87]. In a retrospective review, 6% of patients with infantile‐onset Pompe disease receiving anesthetics developed an arrhythmia or cardiac arrest soon after induction of anesthesia [88]. Two‐thirds of these events were attributed to the use of sevoflurane or propofol. The most common finding in patients with HCM is a hyperdynamic left ventricle with abnormal diastolic relaxation and compliance; LVOT obstruction is present in no more than 25% of patients [89] (Figure 27.6). The degree of outflow tract obstruction is a dynamic process and may be reduced or abolished by decreasing myocardial contractility, increasing preload, or increasing afterload. Conversely, the dynamic obstruction is increased during periods of increased ventricular contractility or sympathetic stimulation, as for instance, with exercise. The outflow tract gradient is felt to be caused in part by anterior movement of the mitral valve toward the ventricular septum during early systole, and more likely to occur in the setting of a hypertrophied anterior septum [89]. Anterior movement of the mitral valve may interfere with optimal valve closure, causing mitral regurgitation, occurring more commonly in the setting of LVOT obstruction. The combination of severe left ventricular hypertrophy, LVOT obstruction, and abnormalities of the intramural coronary arteries all place these patients at an increased risk of myocardial ischemia and ventricular arrhythmias. Figure 27.6 MRI image of a patient with severe hypertrophic cardiomyopathy causing left ventricular outflow tract (LVOY) obstruction. RA, right atrium; RV, right ventricle; LA, left atrium; LV, left ventricle. (Source: Courtesy of Michael Taylor, MD.) Treatment modalities for HCM include medical therapy, surgical therapy, the use of pacing and/or implantable cardioverter‐defibrillators, and cardiac transplantation. Aggressiveness of therapy in any given patient depends on the risk factors for morbidity and mortality. Identified risk factors for patients with HCM include family history of sudden death or syncope, extreme septal hypertrophy, and LVOT obstruction [90, 91]. Asymptomatic patients with HCM may not require any therapy. When medical therapy is initiated, it usually consists of either a β‐blocker or calcium‐channel blocker, and can be guided by treadmill exercise testing or patient symptoms [70]. Another treatment option is dual‐chamber pacing, which has been used as an alternative to surgery with mixed results. Although pacing has been shown to be of benefit in some patients, randomized controlled trials showing long‐term improvement in outcomes are lacking. For patients with HCM that have had a sudden cardiac death event and/or sustained ventricular tachycardia/ventricular fibrillation, placement of an implantable cardioverter‐defibrillator is indicated [79]. Indications for surgical intervention include a left ventricular outflow gradient >60 mmHg, significant symptoms such as dyspnea, angina, or syncope unresponsive to medical therapy, and an increase in mitral regurgitation [92]. Septal myectomy is an effective treatment in both pediatric and adult patients [76, 78]. In pediatric patients with HCM undergoing septal myectomy at the Mayo Clinic over a 28‐year period, there were no early deaths and survival rates at 5 and 10 years were 97 and 95%, respectively. Late follow‐up was remarkable for 96% of patients remaining in New York Heart Association (NYHA) functional class I or II [91]. The surgical approach is commonly transaortic, and often technically challenging in smaller children. Transesophageal echocardiography (TEE) can be used to aid in the assessment of intracardiac anatomy before resection and of the adequacy of resection following myectomy. Despite an excellent surgical result with minimal residual left ventricular outflow gradient, these children remain at risk for cardiac arrhythmias and sudden death [91]. Patients with congestive heart failure, myocardial ischemia, and/or life‐threatening arrhythmias, despite optimal medical and surgical therapies, may be considered for cardiac transplantation. Patients with HCM are at a significant risk for cardiac complications during anesthesia and surgery. A recent retrospective study looking at the incidence of complications in children with HCM undergoing general anesthesia at a tertiary care pediatric center demonstrated a 0.6% mortality rate; minor complications were seen in 12% of patients [92]. One adult study was remarkable for 40% of patients experiencing at least one perioperative adverse cardiac event, and predictors of adverse outcome included major surgery and duration of surgery [93]. To avoid hemodynamic instability, anesthetic management should be tailored toward maintaining preload and afterload, decreasing myocardial contractility, and avoiding tachycardia. Maintenance of normovolemia prior to induction is vital, and the placement of an intravenous catheter and administration of intravenous fluids prior to surgery may be beneficial. While halothane meets many of the hemodynamic goals and has been well tolerated in such cases, it is no longer available [94]. Sevoflurane and desflurane, on the other hand, may cause significant increase in heart rate and reduction in systemic vascular resistance and should be used with caution. The use of an intravenous access for anesthetic induction allows for careful titration of anesthetic agents and for rapid treatment of hypotension and/or cardiac arrhythmias. High‐dose opioid anesthesia provides stable hemodynamics and maintenance of a normal to low heart rate. Remifentanil, a short‐acting potent opioid administered by continuous infusion, may be an excellent choice when extubation of the trachea following surgery is planned. For patients not already on β‐blocker therapy, esmolol can be used in the perioperative period to control heart rate and reduce cardiac contractility. Depending on the procedure and severity of HCM, arterial vascular access and central venous access may be indicated. Patients with a critical LVOT gradient may benefit from pre‐induction placement of an arterial line for close monitoring of systemic blood pressure during anesthetic induction. However, one must carefully judge whether the increased anxiety and pain caused by the procedure might precipitate an adverse event. During placement of a central venous line the patient must be closely monitored for the occurrence of an atrial dysrhythmia. Rapid treatment of dysrhythmias is important, as hemodynamics may deteriorate swiftly. Central venous pressure may be significantly elevated in patients with HCM due to the hypertrophied non‐compliant ventricle.
CHAPTER 27
Anesthesia for Left–sided Obstructive Lesions
Introduction
Aortic valve stenosis
Incidence, anatomy, and natural history
Pathophysiology
Severity
Mild
Moderate
Severe
Mean echo pressure gradient [mmHg]
<20
20–40
>40
Peak transvalvular velocity Vmax [m/s]
2.0–2.9
3.0–3.9
>4.0
Indexed aortic valve area [cm2/m2]
>0.85
0.60–0.85
<0.6
Velocity ratio
>0.50
0.25–0.50
<0.25
Surgical and transcatheter approaches and outcomes
Scoring System
Formula
Severe
Rhodes
14 (BSA + 0.943 (indexed aortic root) + 4.78 (long‐axis ratio) + 0.157 (indexed mitral valve area) − 12.03
≥0.35
CHSS 1
30.55 (inverse of age at study entry [d]–1) − 6.20 (aortic root z‐score) − 12.14 (echocardiographic grade of EFE) − 23.33 (logarithm of ascending aortic [mm]) − 28.30 (presence of moderate or severe tricuspid regurgitation) − 0.70 (LV long‐axis length z score) − 86.47
<0
Discriminant
10.98 (body surface area) − 0.56 (aortic annulus z‐score) − 5.89 (left ventricular to heart long‐axis ratio) − 0.79 (grade 2 or 3 EFE) − 6.78
≥–0.65
CHSS 2
Multivariable regression equation using BSA, mitral valve z score, indexed heart long axis, minimum LVOT diameter, mid‐aortic arch indexed
<0
2 V
[(Mitral valve annulus in 4Ch/aortic valve annulus in PSLA)/(Left ventricular length 4Ch/Right ventricular length 4Ch) + main pulmonary artery diameter]/BSA
≤16.2
Anesthetic considerations
Subvalvular AS
Incidence, anatomy, and natural history
Neonates and infants
Older children
Aortic valve repair
Early mortality: 3–4%
10‐year survival: 94%
10‐year freedom from reoperation: 66%
Early mortality: 0.4–1.8%
10‐year survival: 94%
10‐year freedom from reoperation: 70%
Ross operation
Early mortality: 10–17%
10‐year survival: 79%
10‐year freedom from reoperation: 62%
Early mortality: 0–4%
10‐year survival: 96%
10‐year freedom from reoperation: 90%
Ozaki aortic valve replacement
Not yet reported
Early mortality: 0%
10‐year survival: not reported
3‐year freedom from reoperation: 80%
Mechanical aortic valve replacement
Not feasible
Early mortality: 0.5–7%
10‐year survival: 82%
10‐year freedom from reoperation: 78%
Homograft aortic valve replacement
Not reported
Early mortality: 5–13%
10‐year survival: 85%
10‐year freedom from valve reoperation: 50–60%
Lesions
Percentage of patients
Bicuspid valves
40
Aortic stenosis
28
Ventricular septal defect
24
Coarctation of aorta
12
Patent ductus arteriosus
12
Atrial septal defect
4
Pathophysiology
Surgical and transcatheter approaches and outcomes
Anesthetic considerations
Supravalvular AS
Incidence, anatomy, and natural history
Pathophysiology
Surgical and transcatheter approaches and outcomes
Anesthetic considerations
Hypertrophic cardiomyopathy
Incidence, anatomy, and natural history
Sarcomeric
Malformation syndromes: RASopathies
Noonan syndrome
Noonan syndrome with multiple lentigines
Costello syndrome
Cardiofaciocutaneous syndrome
Glycogen storage diseases
Pompe disease (glycogen storage disease type lla)
Danon disease (glycogen storage disease type llb)
Cori–Forbes disease (glycogen storage disease type III)
PRKAG2 syndrome
Lysosomal storage diseases
Mucopolysaccharidoses
Mitochondrial disorders
Fatty acid oxidation disorders
Very long‐chain acyl‐CoA dehydrogenase deficiency
Multiple‐acyl‐CoA dehydrogenase
Long‐chain hydroxyacyl‐CoA dehydrogenase
Carnitine‐acylcarnitine translocase
Carnitine palmitoyltransferase II
Carnitine‐acylcarnitine translocase deficiency
Endocrine disorders
Primary hyperinsulinism
Infant of a mother with diabetes mellitus
Acromegaly
Pathophysiology
Surgical and transcatheter approaches and outcomes
Anesthetic considerations
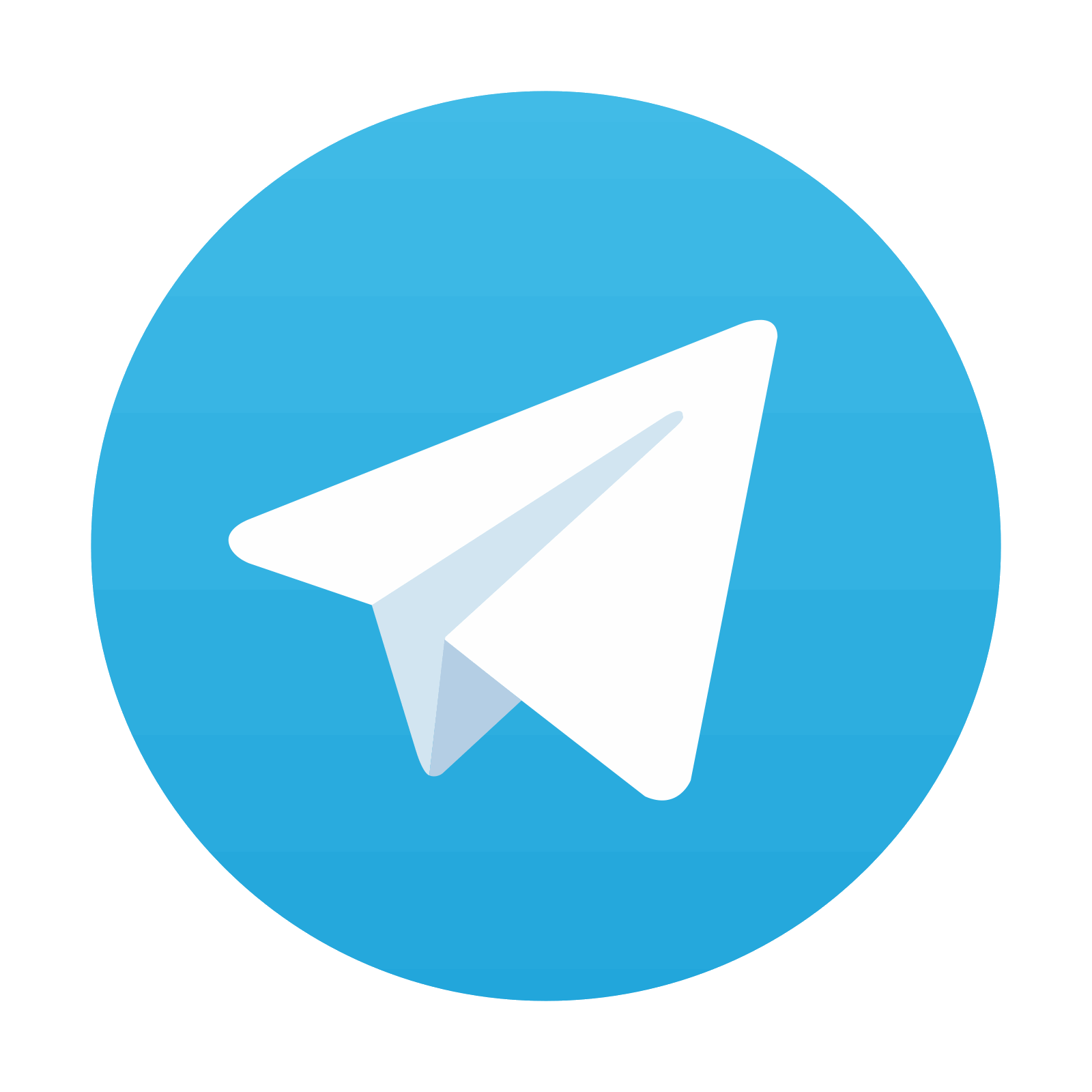
Stay updated, free articles. Join our Telegram channel
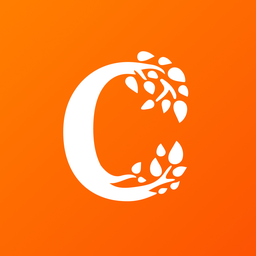
Full access? Get Clinical Tree
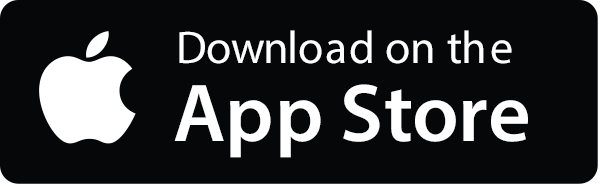
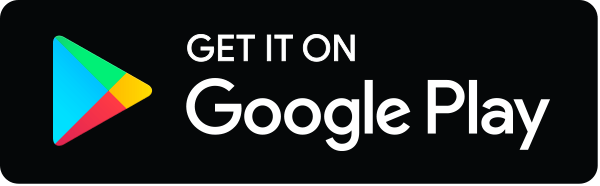