Ralph Gertler1, Dean B. Andropoulos2, and Ashraf Resheidat3 1 Department of Anaesthesiology and Intensive Care, HELIOS Klinikum Munchen West Teaching Hospital of the Ludwig‐Maximilians‐Universitat, Munchen, Germany 2 Texas Children’s Hospital and Department of Anesthesiology, Baylor College of Medicine, Houston, TX, USA 3 Staff Cardiovascular Anesthesiologist and Intensivist, Arthur S. Keats, M.D. Division of Pediatric Cardiovascular Anesthesiology, Assistant Professor, Anesthesiology and Pediatrics, Baylor College of Medicine, Houston, TX, USA On May 6, 1953, John H. Gibbon, Jr. successfully performed, for the first time, open‐heart surgery using cardiopulmonary bypass (CPB) for the closure of a large atrial septal defect. The heart‐lung machine, consisting of a simple oxygenator in conjunction with roller pumps, took over the function of the heart and lungs for a duration of 26 minutes. The machine worked properly during the total bypass time of 45 minutes, apart from some fibrin formation on the oxygenator due to inadequate anticoagulation [1]. Gibbon’s success stood at the end of approximately 20 years of developing a heart‐lung machine. In a 1937 publication, Gibbon published his first results of an experimental CPB in cats. In 1939, the former president of the American Association of Thoracic Surgeons, Leo Eloesser, said during a discussion following a lecture by Gibbon that his presentation reminded him of the seemingly impossible, fantastic stories of the novel writer, Jules Verne, that later often became reality [2]. In a series of 19 consecutive bypass uses in dogs, 12 of the animals survived. But the idea of perfusion with artificially produced circulating arterial blood did not originate with Gibbon. The idea was first documented in 1812, inspired by the French physiologist, César Julien‐Jean Le Gallois. In his monograph “Expériences sur le principe de la vie” he described experiments to define the relationship of the respiratory and nervous system, and blood circulation. On August 9, 1951, in Turin, Dogliotti and Constantini used a partial bypass on humans for the first time [3]. When a patient whose condition during the surgical removal of a large mediastinal tumor drastically deteriorated, a heart‐lung machine was used to maintain perfusion for 20 minutes and the patient was stabilized. On March 16, 1943, Willem Johan Kolff observed, during the clinical use of an artificial dialyzer, that oxygen was quickly absorbed by a cellulose membrane. This observation was the basis for the development of membrane oxygenators. Later Kolff presented the design of the first membrane oxygenator at the first Congress of the American Society for Artificial Internal Organs on June 5, 1955 [4]. Also in the 1950s, Arthur Keats, Denton Cooley and colleagues at Texas Children’s Hospital pioneered several important breakthroughs in congenital cardiac surgery with bypass, including, in 1959, the first description of heparin–protamine titration [5]. In a seminal 1958 article, they described anesthetic problems in their first 200 cases of congenital heart surgery with bypass, which included bypass times; changes in blood gases, electrolytes, and lactate; cardiac rhythm disturbances; and blood transfusions [6]. The first commercial oxygenators became available in the 1960s and the number of open cardiac surgery procedures increased rapidly at that point. Subsequent attempts to use the heart‐lung machine to help correct complex congenital heart defects in small infants were hindered by high morbidity and mortality rates until Barratt‐Boyes and Castaneda started using deep hypothermic circulatory arrest in the late 1960s and early 1970s. Less than two decades later, pediatric cardiac surgery and anesthesia had progressed to providing multistage palliation for infants with single‐ventricle physiology. Today CPB is a standard feature of daily pediatric cardiac anesthesia practice. Advances in neonatal CPB techniques over the last 60 years have been among the most important factors leading to improved outcomes in pediatric cardiac surgery. Extracorporeal perfusion in newborns, infants, and children is in many aspects different from the adult patient. This is caused by the underlying physiologic changes as well as the different pathophysiology secondary to shunt physiology. In this chapter, we review the equipment and techniques used in CPB, with a primary focus on neonatal and pediatric bypass. We will also summarize the effects of CPB on different organ systems. Extensive reviews on the multiorgan effects of congenital cardiac surgery are presented in Chapter 12, and neurological monitoring and outcomes in Chapter 14. In this chapter, we emphasize specific management issues that occur in daily practice. Further details on specific technical issues can be found in excellent extensive textbooks on the topic [7]. A basic bypass circuit (Figure 11.1) consists of a venous reservoir, an oxygenator/heat exchanger unit, roller pumps for perfusion, suction, and cardioplegia and the connecting tubing, cannulae, as well as monitoring and alarm devices. There are major differences between adult and pediatric CPB, stemming from anatomic, metabolic, and physiologic differences in these two groups of patients (Table 11.1). Much progress has been made in the miniaturization of circuits and components. Current technology allows a total priming volume as low as 95 mL for a neonatal circuit setup allowing transfusion‐free repairs in smaller patients down to the 5–10 kg range in some institutions [8–10]. Figure 11.1 Schematic diagram of a cardiopulmonary bypass circuit. This scheme depicts a membrane oxygenator with an integral hard‐shell venous reservoir and an external cardiotomy reservoir. Many circuits have the cardiotomy reservoir, venous reservoir, and oxygenator integrated into one single unit. The systemic blood pump may be either a roller or centrifugal pump. Most pediatric venous cannulations are bicaval with two separate venous cannulas instead of the single venous cannula depicted here. Carbon dioxide can also be added to the inspired gas to facilitate pH‐stat blood gas management. Arrows indicate the direction of flow; P, pressure sensor; T, temperature sensors; X, placement of tubing clamps. (Source: Hessel et al. [7]. Reproduced with permission of Wolters Kluwer.) Table 11.1 Differences between adult and pediatric cardiopulmonary bypass HLHS, hypoplastic left heart syndrome; IAA, interrupted aortic arch. The selection of cannulas occurs on the basis of flow requirements and anatomic relations. Single‐stage venous cannulation is rare and selective superior and inferior vena cava cannulation plus additional cannulation of a left persistent superior vena cava are routine. Thus, the infant patient routinely has an aortic cannula and two venous cannulas. A second aortic cannula may be used for cases of interrupted aortic arch to perfuse the descending aorta (Figure 11.2). Particular care must be observed during inferior vena cava (IVC) cannulation, as obstruction or malposition into a hepatic vein can occur. The addition of a small cannula for venting the systemic ventricle places significant demand on the surgeon to manage the position of the multiple cannulae in the small pericardial space of neonates and young infants. Venting the heart through an existing patent foramen ovale or atrial septal defect (ASD) or creating a small atrial communication is the preferred method for many congenital cardiac surgeons, instead of a cannula in a superior pulmonary vein, to avoid damage to these delicate structures. Figure 11.2 Aortobicaval cannulation in a neonate. IVC, inferior vena cava; SVC, superior vena cava. In daily clinical practice, the amount of systemic venous return on CPB is directly correlated with the amount of pump flow and vice versa. Venous drainage is often limited by the small size of the cannula and tubing as well as individual characteristics, the circuit used, and the use of accessory systems. Generally, venous drainage is obtained by gravity, placing the venous reservoir of the CPB circuit about 30 cm lower than the level of the heart; in this way, a negative pressure equivalent to about 20–25 mmHg is obtained. This is adequate for the vast majority of adult patients undergoing conventional procedures. In pediatric patients, however, where relatively small‐size cannulas and tubing of the venous circuit are used to reduce the pump‐priming volume, vacuum‐assisted venous return is most often used. In this system, a constant vacuum (up to 80 mmHg) is created in the airtight venous reservoir, allowing more blood to be drained from the patient via the venous line. This system allows the performance of surgical procedures on CPB, even in small infants, without the need for large‐size venous tubing and, therefore, without increasing the volume of the pump priming [11, 12]. The major potential limit in the clinical application of this system is the risk of generating gaseous microemboli in the venous circuit. If the arterial pump is stopped for various reasons and the vacuum source is left on the venous reservoir, microbubble transgression can occur from the gas compartment to the liquid compartment of the oxygenator, creating another source of gaseous microemboli as soon as the arterial pump is turned on again [9, 12]. Arterial cannulation is usually via the ascending aorta. Exceptions, however, are frequent in the newborn with malformation of the ascending aorta (e.g. interrupted aortic arch and hypoplastic left heart syndrome [HLHS]). In that scenario, the ductus arteriosus is primarily cannulated to maintain body perfusion on bypass and the pulmonary arteries are snared to prevent runoff until an anatomic correction takes place. The clinician must also be aware that femoral cannulation is not feasible in small children <15 kg because of the small vessel size. Thus, on repeat sternotomy, this method of establishing CPB is usually not an option and careful retrosternal dissection must take place. Table 11.2 Cardiopulmonary bypass tubing volumes Table 11.3 Cardiopulmonary bypass tubing sizes The aortic cannula represents the smallest diameter in the pediatric CPB circuit. The bypass tubing is responsible for the large foreign surface area and priming volume. The strategic selection of tubing dimensions can reduce the volume effectively (Tables 11.2 and 11.3). For example, in a 3.5 kg newborn with an approximate blood volume of 300 mL, the addition of a cardiotomy suction line of 6.3 mm (1/4 in.) diameter and with a total length of 250 cm would require approximately one third of the patient’s blood volume (82.5 mL) before reaching the reservoir. Malposition of cannulas is particularly problematic in pediatric perfusion. Systemic perfusion may be adversely impacted when placement of either venous or arterial cannulas is not ideal. If a venous obstruction occurs, the consequences are magnified because of low perfusion pressures. If the IVC cannula causes venous obstruction in the splanchnic bed, increased hydrostatic pressure causes ascites, and reduced perfusion pressure results in significant renal, hepatic, and gastrointestinal dysfunction. Obstruction of the superior vena cava (SVC) may produce an increase in intracranial pressure and result in decreased cerebral perfusion pressure and cerebral edema. It is possible to observe preferential flow to one side of the cerebral circulation or down the distal aorta (Figure 11.3). Transcranial Doppler monitoring of cerebral flow velocity or cerebral oximetry can provide an early warning of altered flow patterns caused by cannula misplacement [11]. Cooling patterns demonstrating rectal cooling preceding nasopharyngeal cooling may suggest a disproportionate amount of pump flow being directed away from the cerebral circulation [13]. The two pumps most commonly used for CPB are roller pumps and centrifugal pumps. Roller pumps have the advantages of simplicity, low cost, ease and reliability of flow calculation, and the ability to pump against high resistance without reducing flow. Disadvantages include the need to assess occlusiveness, spallation of the inner tubing surface, which potentially produces particulate arterial emboli, the capability of pumping large volumes of air, and the ability to create large positive and negative pressures. Centrifugal pumps offer the advantage of lesser air pumping capabilities, less ability to create large positive and negative pressures, less blood trauma, and virtually no spallation. Disadvantages include higher cost, the lack of occlusiveness (creating the possibility of accidental patient exsanguination), and afterload‐dependent flow requiring constant flow measurement. In the setting of short‐term CPB for cardiac surgery, it remains uncertain whether the selection of one pump over another is of clinical significance; most institutions employ roller pumps for congenital heart surgery. Figure 11.3 Malposition of the aortic cannula high in the ascending aorta. This cannulation technique is helpful for any surgery on the ascending aorta. Particular attention is needed to avoid preferential perfusion of the left cerebral hemisphere. Note the obstruction of flow to the innominate artery in this case. (Source: Gottlieb et al. [11]. Reproduced with permission of John Wiley & Sons.) The efficiency of gas exchange in the natural lung is mainly attributable to the large surface area generated by the airway and circulatory networks and the low resistance to diffusion. These same features are essential to the design of an efficient artificial lung. Other necessary features of an ideal oxygenator include minimal trauma to blood, thromboresistance, minimal reaction with blood components, minimal generation of gaseous microemboli, the ability to maintain performance over long periods, low prime volume, consistent physical properties, reliability, ease of use, and low cost. The efficiency of a membrane oxygenator is two to three times less than that of the natural lung at rest, and about eight times less than the natural lung under conditions of maximal exercise. The primary limiting factor to efficient gas exchange in membrane oxygenators appears to be blood phase resistance to both O2 and CO2 diffusion. A second factor that has limited the use of microporous membranes in situations of long‐term extracorporeal support is the progressive decrease in gas exchange function. The most common microporous membrane oxygenator design in clinical use is the hollow‐fiber type in which the polypropylene membrane is formed into fibers that are bundled or woven together. The fibers are 200–250 μm in diameter and 10–15 cm long, and the membrane thickness is 25–50 μm. Although the existence of micropores in the membrane significantly increases the gas exchange of membrane oxygenators, long‐term use results in the progressive wetting of the surface, plasma leakage through the pores, and subsequent deterioration of membrane performance, thus limiting its use to acute CPB procedures. The newest oxygenators incorporate an arterial line filter to reduce priming volumes. Bypass prime composition and volume are adapted to the particular requirements of the patient. The composition, however, is often a matter of opinion and differences are as great as the number of pediatric heart centers. If there is a consensus, it is probably only with regard to reducing volumes to a minimum to reduce transfusion requirements and dilutional effects of the patient [8, 14]. Despite recent advances made in technology, the majority of neonates and infants still require perioperative transfusion of homologous blood components. The lower the body weight of the patient, the more banked blood products need to be added to the prime. To maintain colloid osmotic pressure and a minimal amount of coagulation factors, albumin and fresh frozen plasma (FFP) are added. The most suitable priming in neonates seems to be the combination of albumin or FFP and red blood cells in such proportions so as to maintain the hematocrit (Hct) at about 28–30% during normothermia. A few centers are still able to obtain fresh whole blood (<48 hours after donation) for neonates, but outcomes of studies using this technique vs. “reconstituted” whole blood (FFP plus packed red blood cells [PRBCs] from the same or different donors) are conflicting [10, 15, 16]. Albumin is reported to maintain colloid osmotic pressure (COP; normally around 20–25 mmHg) and to reduce fluid accumulation [8, 10, 17, 18] while adding FFP to the prime maintains COP increases the level of coagulation factors and reduces intraoperative transfusion requirements in complex neonatal and cyanotic cases [12, 19]. However, postoperative transfusion requirements are not affected despite higher fibrinogen concentrations and better thrombelastographic values at the end of CPB [9, 20]. The level of ionized calcium is adjusted, as all blood products contain significant amounts of citrate. This can lead to acute hypocalcemia and cardiac arrest with the initiation of bypass. In addition, added erythrocyte concentrates should be as fresh as possible (<3–5 days old) to avoid hyperkalemia and hyperlactatemia as side effects. The reasons for this suggestion are that the level of 2,3‐diphosphoglycerate in stored red blood cells decreases, metabolic load increases (potassium and lactate levels by the end of the second day are up to 7–25 mmol/L [11, 21]), and the risk of complications with bypass is higher. The risk is particularly high in infants <5 kg if the prime contains irradiated blood [13, 22]. If the red blood cells (RBCs) in the prime are older than 5 days, a prime blood gas should be checked and corrected. Circulating and filtrating the prime for 20 minutes alleviates most of these problems. Alternatively, processing PRBCs via a cell saver is reasonable and may add additional benefits such as the avoidance of hyperglycemia, high citrate levels, and hyperkalemia [14–16, 23, 24]. In addition, lactate levels are reduced and microaggregates >20 μm are eliminated. Care must be taken to avoid using normal saline as a washing solution, as a hyperchloremic metabolic acidosis can be induced in newborns and infants. In addition, the use of cell‐saving devices during pediatric cardiac surgery provides another means of reducing banked blood exposure [25]. A desirable practice is to adjust the prime composition in neonates and small infants to the patient’s blood, with pH, Na+, K+, Ca2+ and temperature within narrow ranges. PRBC requirements can be calculated on the basis of weight and starting Hct. The change in Hct, ΔHct, is calculated as follows: where HctPAT is patient hematocrit, BVPAT is the patient blood volume (see Table 11.4) and PV is the priming volume. The transfusion requirement is calculated as: where 60% is the average Hct of stored PRBCs. After initial prime composition, an Hct on the prime can be measured, and a further adjustment before CPB is made to achieve Hct in the goal range. In newborns, a goal Hct on the pump of 30–35% is maintained. Older patients or special circumstances (e.g., severe hypoxia) may require adjustments to a lower limit of around 25% or higher than 30% (severe hypoxia), even though both limits are controversial. Historically, many centers permitted marked hemodilution on CPB to avoid transfusion. Recent studies question this approach and provide evidence of improved neurological function when higher Hcts are maintained during CPB. A randomized controlled clinical study in infants undergoing CPB demonstrated adverse perioperative and developmental outcomes with extreme hemodilution [26]. This issue remains controversial, although the clinical data in support of higher Hcts of at least 25% are compelling [27, 28]. Also, hemostasis may be improved by higher Hct levels. An interesting strategy described in recent years is utilizing a neonate’s own umbilical cord blood (UCB) for prenatally diagnosed patients undergoing cardiac surgery with bypass [29]. UCB is collected in a sterile fashion in the delivery room, and with milking of the umbilical cord, 50–140 mL of UCB can be obtained. After sterile processing, the UCB can be utilized as whole blood, or separated into packed RBC and fresh frozen plasma components. UCB contains stem cells and other progenitor cells, growth factors, and cytokines, in addition to RBC. Theoretical advantages of UCB are lack of immunogenicity, high concentration of fetal hemoglobin to facilitate unloading of oxygen into the tissues, and potential benefits of the progenitor cells. Half of the collected blood can be used to prime the bypass machine, and half can be used for post‐bypass transfusion. Drawbacks to UCB include complexity of collection, bacterial contamination, and limited storage time. Small single‐center studies from a few institutions are the only data available, but UCB appears to be safe. Larger studies with a more careful assessment of the fate of all of the cell types are needed before wider use of UCB for neonatal surgery. Table 11.4 Estimated patient blood volumes by weight Major differences need to be considered when dealing with the pediatric patient on CPB (Table 11.1). These include the degree of hemodilution, flow rates and perfusion pressure, temperature, cannulation, prime and blood gas management, ultrafiltration, hemodynamic management, and, in certain cases, the presence of aortopulmonary collaterals. Recent efforts to minimize circuit volumes have led to the development of smaller circuit elements. However, there continues to be a gross degree of hemodilution realized in the neonatal patient. This can be as much as three to 15 times the amount of hemodilution seen in an adult. For example, in a 3 kg child given 85 mL/kg, an estimated blood volume (EBV) of 255 mL contrasts with an average circuit prime volume of 300–400 mL. Thus, a prime : EBV ratio can exceed >2–3 : 1, or >100–150% of a neonate’s blood volume. In the average adult circuit, only a 25–33% dilution rate is realized. This degree of dilution necessitates the addition of donor blood up to a body weight of approximately 10 kg to maintain an adequate Hct for optimal oxygen delivery on bypass. Management of the pediatric patient undergoing CPB is made difficult by competing hemodynamic goals for the brain, heart, and viscera. The brain is protected from hypoperfusion by systemic vasoconstriction and pressure autoregulation. Optimal mean arterial pressure targets are not uniform. They depend on cerebral autoregulation and vasoreactivity. This varies by age and underlying conditions. Patients with prematurity, previous brain injury, underlying congenital heart disease, hypothermia, or patients on ECMO can have abnormal cerebral autoregulation which makes it difficult to optimize the brain perfusion pressures. Invasive measurements of cerebral blood flows are not feasible, but non‐invasive continuous monitoring of pressure autoregulation may be performed using near‐infrared spectroscopy (NIRS) as a surrogate of CBF by using the hemoglobin volume index (HVx). The HVx is a moving correlation between arterial blood pressure and blood volume measured by cerebral oximetry and is able to evaluate individual autoregulatory thresholds [30]. See Chapter 14 for additional detail. Perfusion pressures in the neonate can be quite low, less than 30 mmHg. This is often due to the lack of reactivity of the neonatal vasculature or the presence of a shunt like a patent ductus arteriosus (PDA). With meticulous management of blood gases, Hct, temperature, and flow rates, the goal should be the minimal use of vasoconstricting agents (i.e. phenylephrine) to maintain ideal blood pressure. As a rule of thumb, perfusion pressure (MAP [mean arterial pressure] – CVP [central venous pressure]) goals in the neonate are 35–45 mmHg, and over the first 2 years of life, the target perfusion pressure increases to 45–50 mmHg. Although not yet validated by neurologic outcome studies, the lower limit of autoregulation as measured by the HVx in neonates averages about 30 mmHg in the first week of life and increases to about 40 mmHg by 30 days, with considerable variation [31]. At best, normal flow rates are combined with reasonable perfusion pressures to reach all tissue beds, including those that require a certain opening pressure for function (kidney, brain, splanchnic bed). One should also keep in mind that the lower limit of myocardial perfusion pressure is around 15 mmHg, calculated as MAP – CVP. This is relevant for diastolic pressures below 30 mmHg. The flow rates for neonates are quite variable, ranging from 0 to 200 mL/kg/min. Deep hypothermic circulatory arrest (DHCA) is at one end of the spectrum, contrasted with high metabolic demands, vent return, circuit shunts, or patient collaterals, all of which contribute to the necessity of high flow rates at the other end. This can often exceed a cardiac index of 3 L/min/m2. The flow rates are calculated on the basis of weight, but the perfusionist must adapt flows according to the individual case and to meet the demands. One strategy is to employ standard full flow rates in neonates and infants up to 10 kg of 150 mL/kg/min. With hypothermia and decreased metabolic demands, many centers will reduce flows to 80–100 mL/kg/min to reduce venous return to the field, lowering flows further to 25–50 mL/kg/min during deep hypothermia for intracardiac complex repairs. Recent data has demonstrated the feasibility of goal‐directed, personalized CPB flow rates utilizing parameters such as mixed venous oxygen saturation (MVO2) measured continuously in line from the CPB circuit, cerebral and somatic oxygen saturation measured by near‐infrared spectroscopy, arterial blood gases, hemoglobin, and lactate concentration. Goals such as cerebral oxygen saturation (rSO2) above 50%, maintaining somatic rSO2 values higher than cerebral rSO2, MVO2 >70%, and lactate less than 2.5 mmol/L have been reported [32]. Bojan and colleagues retrospectively reviewed 180 neonatal surgeries and serum lactate values, and calculated oxygen delivery (DO2, mL/min/m2) utilizing CPB flow rates, hemoglobin concentrations, and PaO2. [33] They determined a minimum DO2 to maintain aerobic metabolism, indicated by a peak lactate concentration after aortic cross clamp removal <2.5 mmol/L, at normothermia and different levels of hypothermia. The average minimum DO2 at 37 °C was 340 mL/min/m2, a value significantly higher than older children and adults. Minimum DO2 decreased linearly with temperature (Figure 11.4). The same group published a retrospective study of somatic to cerebral rSO2 difference during CPB in 24 neonates, and reported that there was a predictable increase in lactate concentration when the somatic to cerebral rSO2 difference decreased or became negative [34] (Figure 11.5). Although potentially more complex than a standard flow rate specified according to weight or body surface area, combined with temperature, this approach of varying CPB flow according to oxygen demand may balance minimum oxygen delivery needs with the need to reduce flows to decrease blood return to the surgical field. More data, along with outcome studies, are needed to determine whether the goal‐directed approach has any advantage over current methods. Figure 11.4 Oxygen delivery as a function of body temperature during aortic crossclamping. In the left panel are shown the observed oxygen deliveries (DO2) in patients with first lactate after aortic crossclamp was removed (lactOFF) <2.5 mM, and the estimated −1, −1.5, and −2 DO2 Z scores, as a function of body temperature. In the right panel are the observed DO2 values in the overall population, as a function of temperature, and several DO2 thresholds tested for associations with lactate production. These were empirical thresholds chosen in an attempt to refine the −2 Z score. DO2 threshold of 357 mL/min/m2 was identified in the lactOFF <2.5 subgroup and found to be linked with lactate production. The final analysis revealed that the lowest threshold linked with lactate production and thought to represent the lowest suitable DO2 during normothermic neonatal bypass was 340 mL/min/m2. (Source: Bojan et al. [33]. Reproduced with permission of Elsevier.) Figure 11.5 The somatic‐cerebral NIRS gradient, lactate concentrations, and temperature during cardiopulmonary bypass in a neonate. For each time interval between two lactate measurements, the lactate concentration variation and the somatic‐cerebral rSO2 gradient were calculated. The somatic‐cerebral rSO2 gradient was the sum of all positive and negative areas resulting from excursions of the somatic rSO2 above and below the cerebral rSO2 and was calculated aby the trapezoidal method. rSO2, regional oxygen saturation. (Source: Bojan et al. [34]. Reproduced with permission of Oxford University Press.) It is the current practice in some institutions to employ pH‐stat management in all cases in which temperatures are taken to hypothermic levels. In this temperature‐corrected strategy, the PCO2 remains unchanged from 37 °C (40 mmHg). This strategy allows for cerebral vasodilation and more even cooling. The corrected PCO2 ranges at 37 °C can be >80–100 mmHg. Uncommon in adults, but fairly common in patients with chronic cyanosis with decreased pulmonary blood flow, aortopulmonary collaterals can be a challenge. It can be difficult to empty the heart. Flow rates are frequently affected, and temperatures may need to be lowered substantially to accommodate lower bypass flows to reduce blood return to the operative field. Flows may need to be decreased in conjunction with the use of vasodilating agents. Phentolamine 0.1 mg/kg and nitroglycerine are the drugs of choice during cooling and rewarming, titrated to effect. Phenylephrine is contraindicated and would only enhance collateral flow. As a very basic comparison, there is a trend toward conducting adult cases at tepid or normothermic temperatures while many neonatal cases are still utilizing cold temperatures with or without DHCA. However, more and more neonatal cases are performed at mild hypothermia. The perfusion considerations are multiple, and this topic is discussed in a separate section on hypothermia and DHCA. It is of utmost importance to maintain euglycemia in the neonate. Although there are increased glycogen stores in the neonatal myocardium, there are low hepatic glycogen stores. Exogenous glucose may be necessary in the early neonatal period to maintain normal glucose levels and should be continued into the pre‐bypass period. Perfusion considerations on CPB are directed at efforts to maintain normal glucose levels such as washing packed cells or minimizing glucose content in cardioplegia or intravenous (IV) fluids. When glucose levels are greater than 300 mg/dL, a saline hemodilutional washout with the hemoconcentrator is utilized. Frequent monitoring is recommended. Levels should be maintained at approximately 150 mg/dL just prior to DHCA. Hyperglycemia worsens neurological injury, as elevated glucose levels result in the increased anaerobic metabolism of glucose and increased lactic acidosis. This leads to further depletion of ATP. Hypoglycemia alone can be treated but coupled with hypothermia, cerebral blood flow may be compromised by altering autoregulation. This can be further exacerbated with hyperventilation, as this may occur in weaning a patient with pulmonary hypertension from CPB. Overall, tight glycemic control (blood glucose level in the range of 80–110 mg/dL) is not indicated in children as the outcome was not any different from standard care in the intensive care unit (ICU) [35]. Hyperglycemia was not associated with lower neurodevelopmental outcomes at 8 years of age in the Boston Circulatory Arrest Study. However, lower glucose measurements below 90–100 mg/dL after CPB were associated with a higher risk of electroencephalogram (EEG) seizures. The authors speculate that limiting glucose as an important energy substrate for the neonatal brain, which may have increased requirements under conditions of low CPB flows or DHCA, may be a reason for this association [36]. In a more contemporary study of 93 neonates undergoing the arterial switch operation, patients with more than 50% of the intraoperative and early postoperative periods spent at glucose values between 80 and 110 mg/dL had more perioperative complications than those with >200 mg/dL more than 50% of the time [37]. The practice of many pediatric cardiac anesthesiologists is to maintain plasma glucose levels in the 100–200 mg/dL range for neonates and small infants. One circuit size for the adult patients at a given institution can adequately provide flows for patients from 50 kg and more. Most pediatric centers employ two or three circuits on the basis of the patient’s weight, procedure, and flow requirements. Utilization of ultrafiltration in some form, whether it be conventional or modified, is seen in >90% of our neonatal and pediatric cases. Several system modifications may be necessary to allow for these options. Cardiac cases using CPB can be divided into several basic phases: pre‐bypass period and anticoagulation, bypass period with initial cooling, cross‐clamping and myocardial protection, reperfusion of the heart, separation from CPB, modified ultrafiltration and hemostasis, and, lastly, sternal closure and transfer to the ICU. The pre‐bypass period begins with surgical incision and lasts through to initial dissection and preparation for cannulation. During this period, transesophageal echocardiography (TEE) is performed to confirm the diagnosis and establish a basis for post‐bypass comparison. Baseline activated clotting time values are obtained and metabolic abnormalities are corrected. Cannulation of the great vessels just prior to CPB can often precipitate arrhythmias, hypotension, and arterial desaturation, especially in small infants and children. Ventilation may need to be limited to minimize lung excursion to allow precise cannulation in small infants. Coordination with the surgeon is particularly important. Hemodynamic stability is maintained by cautious fluid administration and small boluses of vasopressors, as deemed necessary. After placement of the aortic cannula, pressure in the aortic root is measured by the perfusionist through the cannula in situ and should be close to the MAP of the patient. A small volume infusion of 2–5 mL/kg is often used at that point to ensure unobstructed aortic cannula flow before bypass. If the aortic cannula is already in place, it is common practice to coordinate the administration of volume between the anesthesiologist and perfusionist while the surgeon completes cannulation. Development of the coagulation system is incomplete at birth and continues in the postnatal period until the age of about 6 months. This increases the risk of bleeding disproportionately in the neonatal and infant group up to 1 year or approximately 8 kg of weight [38–40]. Coagulation in cyanotic infants may be particularly impaired secondary to polycythemia, lower fibrinogen levels, low platelet count and abnormal platelet function [41], decreased concentrations of factors V, VII, and VIII, and increased fibrinolysis [42, 43]. Of particular importance is the role of antithrombin (AT) levels that do not reach adult values until 3–6 months. This low level of AT may reduce the ability of heparin to provide anticoagulation adequate to prevent thrombin generation during CPB in infants [38, 42, 44–46] and an exaggerated inflammatory response may occur [38, 42, 43, 47, 48]. Infants may require an initial heparin dose of 400 units/kg or higher. Manlhiot and colleagues reported AT levels and heparin consumption in 90 infants <1 year undergoing CPB surgery.[49] Baseline AT levels were strongly dependent on age, with lower levels in neonates (Figure 11.6). Lower AT levels were also associated with lower heparin response as measured by anti‐factor Xa levels and higher heparin consumption (Figure 11.7A and B). It has also recently been shown that in infants with CHD, levels of other thrombin inhibitors are depressed compared with healthy infants. This may partially explain the high levels of thrombin generation during infant CPB. The coagulopathy induced by CPB affects children more profoundly than adults. There are many factors implicated, including hemodilution, contact activation, and initiation of a systemic inflammatory response. Despite large doses of heparin during CPB, heparin does not block thrombin generation completely but partially inhibits thrombin after it is produced. Thrombin is continuously generated, and a consumptive coagulopathy is initiated [44, 46]. The lower potential of newborn plasma to generate thrombin might, in part, downregulate thrombin markers during CPB. This, however, does not seem significant enough to completely prevent the subsequent reperfusion‐induced thrombin peak [42, 43, 47–48, 50]. Thromboelastography (TEG) has actually shown that neonates and infants develop faster and stronger clots than adults [38–40, 51]. In addition, acquired coagulation defects in 58% of non‐cyanotic and 71% of cyanotic infants have been reported [41, 52–54]. If thrombin formation could be completely inhibited during CPB, the consumption of coagulation proteins and platelets could largely be prevented. Initial heparin doses range from 300–400 units/kg bolus before cannulation to 200–400 units/kg in the circuit, and to 50–100 units/kg ongoing administration every 30–120 minutes. Heparin’s peak therapeutic effect occurs within 2 minutes. CPB may delay the peak effect by 10–20 minutes from hypothermia or hemodilution. Plasma binds 95% of heparin with some uptake by the extracellular fluid, alveolar macrophages, splenic/hepatic endothelial cells, and vascular smooth muscle. The plasma half‐life is dose‐dependent (i.e. 126 ± 24 minutes at a dose of 400 units/kg vs. 93 ± 6 minutes at a dose of 200 units/kg) [38–40, 55]. Heparin is metabolized by the reticuloendothelial system and is eliminated by the kidneys. Hypothermia and renal impairment, but not hepatic impairment, delay elimination. A roughly linear relationship exists between heparin dose and the activated clotting time (ACT) if certain criteria are maintained [41, 56], namely normal ATIII and factor XII activities, normothermia, near‐normal platelet function, a platelet count >50,000/dL, and fibrinogen concentration >100 mg/dL. However, in children, the correlation is rather poor [42, 43, 57]. An ACT is measured before heparinization and repeated a minimum of 3 minutes after giving heparin. CPB is not initiated until an adequate ACT or heparin level is confirmed. Figure 11.6 Antithrombin levels by age at surgery. Blue circles represent individual patient values; solid lines show the estimates from regression models, and dotted lines are the 95% confidence interval. Manlhiot et al. [49] / Reproduced with permission of Elsevier. Figure 11.7 (A) Association between preoperative AT level, and heparin response as measured by anti‐factor Xa level per 100 units/kg heparin. (B) CNEW. Association between preoperative AT level, and heparin dose throughout CPB, indexed for weight and duration of CPB. Blue circles represent individual patient values; solid lines show the estimates from regression models, and dotted lines are the 95% confidence interval. (Source: Manlhiot et al. [49] / Reproduced with permission of Elsevier.) Given the variability in the ACT, heparin concentration can also be measured directly. A two‐point (straight line) dose‐response curve assists in judging how much additional heparin to administer. Acceptable levels during CPB are 2–4 units/mL, and in the newborn population up to 6 units/mL. This regimen increases required heparin doses on CPB but results in lower protamine doses and less blood loss [45, 58]. The optimum method of assessing adequate anticoagulation during CPB in children has thus not yet been defined and much work is required in this area. The use of heparin‐coated biocompatible perfusion circuits is probably helpful in reducing the degree of activation of the coagulation system in children [47, 48, 59, 60], but it is rather expensive and has not gained wide acceptance. The optimal ACT for CPB is controversial. Although the minimum recommended ACT is 400 seconds, other authors recommend 480 seconds [44, 46, 61], as heparin only partially inhibits thrombin formation. This is done to minimize the consumptive coagulopathy that may result from barely adequate anticoagulation. Failure to achieve a satisfactory ACT may be due to inadequate heparin or low concentrations of ATIII, or “heparin resistance.” [49, 62]. Increases in acute‐phase reactant proteins such as factor VIII and fibrinogen commonly shorten the APTT and may appear as heparin resistance. If 500 units/kg of heparin fail to achieve an adequate ACT, ATIII deficiency becomes more likely and FFP or recombinant ATIII (30 IU/kg) [50, 55] is necessary to increase antithrombin concentration. Heparin dosing, ACT targets, and protamine reversal dosing were first established in the 1950s and published in a landmark paper by Keats, Cooley, and Telford from Texas Children’s Hospital [63]. Today more than 60 years later, this approach is still used in most congenital heart surgery centers. The need for alternatives to heparin for bypass anticoagulation has been discussed for years, in part because of not only the problem of heparin‐induced thrombocytopenia predominantly seen in the adult population but also the ongoing issue of bleeding and thrombosis with heparin in pediatric cardiac surgery despite many advances in monitoring and treatment of coagulopathy. Direct thrombin inhibitors such as bivalirudin can be utilized for pediatric cardiopulmonary bypass; the drawbacks with this drug are several and include that there is no reversal agent and it has a half‐life of approximately 25 minutes, so a loading dose and infusion are necessary. In addition, bivalirudin lacks a widely available point‐of‐care test. The ecarin clotting time for bivalirudin is primarily a research tool. The ACT is used as a rough guide to anticoagulation, along with a viscoelastic method of coagulation assessment. Hasija and colleagues published a randomized, controlled safety and efficacy study of bivalirudin vs. heparin in 50 acyanotic patients aged 1–12 years who were undergoing corrective cardiac surgery with bypass[64]. Furthermore, 400 units/kg of heparin plus pump prime, vs. 1 mg/kg bilvalirudin bolus followed by 2.5 mg/kg/hour, and a pump prime dose, was studied. Using a 480 second ACT goal, 1 of 25 heparin patients required extra dosing as compared to 13 of 25 bivalirudin patients. ACT returned to baseline after protamine in the heparin group but remained elevated for an average of 2 hours in the bivalirudin group. With viscoelastic testing, heparin prolonged the onset of clotting, decreased the rate and strength of clot formation, and inhibited platelet function to a greater degree than bivalirudin. Duration of surgery was longer in the bivalirudin group, but chest tube drainage and transfusion were similar. No adverse events occurred. More pediatric cardiac patients are arriving in the operating room (OR) because cardiac surgery is being anticoagulated with direct thrombin inhibitors. Patients on ventricular assist support achieve better and more accurate anticoagulation with these agents, namely bivalirudin, and this agent is now standard of care in many programs for a ventricular assist device (VAD), and even some ECMO patients [65, 66]. Bivalirudin infusion is usually discontinued prior to or on arrival to the OR. It is not thought to affect anticoagulation or hemostasis with heparin on bypass because of its relatively short half‐life, but increased bleeding may occur with surgical dissection prior to bypass. After heparinization and cannulation, CPB is initiated by opening the venous outflow cannula to the reservoir. Slow decompression of the heart and maintaining a minimal output of the beating heart reduce the drop in blood pressure due to the hemodilution. Based on weight or body surface area, a flow requirement is calculated. The cardiac index is approximately 25–50% greater than that of an adult. For newborns, a flow of 2.6–3.2 L/min/m2 is recommended, and for infants, a flow of 2.4–2.6 L/min/m2 is sufficient. If normothermic CPB is chosen, flow rates in the range of 3.0–3.5 L/min/m2 are required [51–53, 67]. This can be reduced during hypothermia (Figure 11.8) [68]. Infants have a much more compliant vasculature, which results in lower perfusion pressures on CPB. Causes of severe hypotension after initiation of bypass can be the presence of hemodynamically relevant shunts (e.g. major aortopulmonary collateral arteries) previously placed systemic to pulmonary artery (PA) shunt or a PDA, which both lead to a circulatory steal in the systemic circulation, requiring higher flows and possibly early control by the surgeon. Increased bronchial and non‐coronary collateral flow draining into the left atrium can be a particular problem, especially in cyanotic children, with a resultant significant negative impact on myocardial protection during periods of aortic cross‐clamping. Because of the small and easily obstructed vena cavae in infants, careful assessment for venous obstruction is carried out immediately after initiation of bypass. The patient’s head and face are assessed for signs of venous obstruction, including plethora and cyanosis of the scalp and face, distended fontanelle, and decrease in cerebral oxygen saturation to levels below the baseline. If a central venous catheter has been placed with its tip in the SVC, pressures should be low (i.e. <5 mmHg) and at times are negative if the placement is adjacent to the SVC cannula. Venous return judged by the volume in the venous reservoir is continually monitored by the perfusionist, with a sudden decrease prompting communication with the surgeon to investigate the cause. Figure 11.8 Nomogram relating oxygen consumption to perfusion flow rate and temperature. X indicates clinical flow rates used by Kirklin and Barrett‐Boyes [68]. (Source: Kirklin and Barrett‐Boyes [68]. Reproduced with permission of Elsevier.) The adequacy of perfusion is monitored by the usual parameters. These are MAP, the in‐line measurement of mixed venous saturation, pH, base excess, and the regular testing of blood for heparin levels and lactate and other indices of end‐organ perfusion (e.g., urine output, somatic and cerebral oxygenation values [NIRS for example]). (See above under flow) Systemic cooling is often utilized for myocardial, cerebral, and other end‐organ protection. Hypothermia is usually classified as mild (32–36 °C), moderate (24–32 °C), or deep (18–24 °C). In general, lower temperatures are used for more complex operations that carry a greater potential for requiring periods of low‐flow bypass or circulatory arrest. Cooling is primarily achieved through the heat exchanger in the bypass circuit, although surface cooling with lower ambient air temperatures or forced‐air warming systems set to lower temperatures is used in some institutions. Infants have a high ratio of surface area to body weight. Infants also have an immature thermal autoregulatory center. Because of these factors, wide fluctuations in body temperature occur easily. Warming and cooling on bypass occur much more readily. If the clinician is not careful, cooling can occur too rapidly, and deep brain structures may become dangerously cold. There is evidence to suggest that cooling too rapidly is deleterious to neurological function. Thus, it is important to cool slowly, evenly, and completely, and most investigators recommend at least 20 minutes of cooling if circulatory arrest is used (i.e., no faster than 1 °C/min) [69]. The opposite is also true. It is very easy to warm too rapidly and for hyperthermic overshoot to occur. Recent data suggest that hyperthermia can be very damaging, and temperature differences of even 1 or 2 °C are highly significant [70]. Monitoring should include nasopharyngeal, rectal, and arterial inflow temperatures. Rewarming the patient should occur at less than 8 °C temperature difference between the heat exchanger and patient blood temperature, with maximal inflow temperatures of 37.0 °C to avoid the formation of any micro air bubbles [71–73]. Special bypass techniques such as regional cerebral perfusion (see later) have been developed to avoid the necessity of using DHCA and may also be performed during this time. In recent years, a number of centers have reported normothermic or mildly hypothermic bypass for a variety of congenital cardiac operations including some more complex neonatal surgeries such as the arterial switch operation [74]. Theoretical advantages include time saved by avoiding cooling and rewarming, improved coagulation and hemostasis, better oxygen and glucose transport in the neonatal brain, cellular membrane stability, better ATP production and utilization, and preservation of cerebral autoregulation. Despite the theoretical advantages, a meta‐analysis of seven randomized controlled trials in pediatric patients revealed no differences in duration of CPB or aortic crossclamp, or in lactate or creatinine concentrations [75]. Corno and colleagues published a retrospective review of 99 patients <2 years of age undergoing normothermic (>35 °C) vs. hypothermic (28–34 °C) bypass, matched for cardiac lesion and surgical procedure [76]. They reported earlier extubation, shorter ICU stay, and lower transfusion requirement in the OR and ICU in the normothermic patients. Limitations of this study were its retrospective single‐center nature, imperfect matching of patient groups, and small sample size. Neurodevelopmental follow‐up of the cohort at 2–3 years after surgery revealed no differences between groups across multiple tested domains [77]. From the data reported so far about normothermic bypass for a variety of congenital operations, this approach would appear to be safe, but not to consistently lead to improved early clinical outcomes or shorter operating times. Repair of most congenital heart lesions is becoming more feasible. Perioperative myocardial damage remains the most common cause of morbidity and mortality after successful surgical repair. Thus, effective myocardial protection assumes an even greater role than in the adult, as perioperative insults are less well tolerated and more difficult to treat. Neonatal hearts may be difficult to protect because of immaturity, cyanosis, hypertrophy of the right ventricle, complex coronary artery pattern, and duration of ischemia to achieve a good repair. The neonatal heart is ultrastructurally immature. Myofibrils are arranged in a disorderly fashion and have a smaller percentage of contractile proteins than do those in adults (30 vs. 60%) [78]. The immature heart shows fewer mature mitochondria and a lower oxidative capacity [79]. Control of myocardial contractility in infants depends more on adrenal function and circulating catecholamines than on direct autonomic influences. There are also differences in myocardial calcium metabolism. In the mature myocardium, the sarcoplasmic reticulum is the predominant source of calcium ion for excitation‐contraction coupling, but the sarcoplasmic reticulum is poorly developed in the immature heart (see Chapter 9). Because the neonatal myocardial cell is deficient in T‐tubules, it is incapable of internal release and reuptake of calcium for contraction and instead depends heavily on transmembrane calcium transport for myocardial contraction. These differences in calcium handling by the cell provide some explanation for the clinical observation that newborns require greater serum ionized calcium levels for optimal myocardial contractility. Experimental evidence suggests that the newborn myocardium tolerates ischemia and reperfusion better than the adult heart. However, in practice, pediatric patients undergoing cardiac surgery have a greater incidence of low cardiac output postoperatively than adults. The discrepancy between the experimental evidence and the clinical experience may be related to the cardiac anomalies involved. Ventricular hypertrophy and cyanosis are common, and the hypertrophied heart has been found to have decreased subendocardial blood flow [80] and lower concentrations of high‐energy phosphates before arrest. Chronic cyanosis has been associated with a decreased threshold for anaerobic metabolism during stress [81], diminished myocardial reserve [82], asynchronous left ventricular wall motion [83], and downregulation of β‐adrenergic receptors [84]. Reoxygenation injury with the release of oxygen free radicals upon initiation of CPB can further exacerbate the pre‐existing injury [85]. The abrupt increase in oxygen levels on bypass in chronically hypoxic infants leads to the loss of antioxidant reserve capacity and subsequent loss of myocardial function. Preventive measures include leukodepletion of blood prime, use of in‐line arterial filters, and normoxic management (PaO2 of 80–100 mmHg) initiating bypass. Over the course of 10–20 minutes, the fraction of inspired oxygen ratio (FiO2) can be increased to obtain PaO2 levels in the usual 100–150 mmHg range. As blood is fully oxygenated at such levels, further increases in oxygen levels only confer minimal increases in the oxygen content of the blood. Finally, many congenital cardiac procedures require an incision of the ventricular muscle, which markedly changes the geometry of the chamber and induces focal edema. The cornerstone of myocardial protection in pediatric cardiac surgery is hypothermia. Systemic hypothermia is, to some degree, used in nearly all congenital cardiac repairs. It is particularly important in cyanotic infants with increased non‐coronary collateral flow to the heart. Myocardial protection can be problematic in these cases because of cardioplegia washout and rewarming of the myocardium. Because systemic hypothermia allows reduced flow rates, it decreases myocardial collateral flow. Blood cardioplegia is often used in adults, but its advantages may be lost in infants undergoing deep hypothermia. However, if greater cardioplegic temperatures are used (warm cardioplegia), there is a clear advantage to the use of blood cardioplegia [86]. The optimal electrolyte composition of cardioplegia for pediatric patients is controversial. There is great institutional variation among solutions used. A certain amount of calcium is necessary in the solution to prevent the severe injury that may result from calcium paradox [87]. However, excessive amounts are deleterious. The addition of magnesium, a natural calcium antagonist, may solve this dilemma. Increasing the ionized magnesium level has been shown to improve postoperative rhythm stability and reduce calcium‐induced mitochondrial dysfunction during reperfusion. Thus, in the absence of magnesium enrichment, hypocalcemic cardioplegia results in adequate myocardial protection in stressed hypoxic hearts. Magnesium was particularly beneficial during normocalcemic cardioplegia solution [88]. In addition to the specifics of cardioplegia administration reviewed in the following, a multifaceted approach to myocardial protection is taken by many institutions. For example, bypass prime composition is adjusted to closely match the patient’s blood with regard to pH, serum Na+, K+, Ca2+, and temperature. Initiation of bypass in a neonatal heart with cold, acidemic, hypocalcemic, hyperkalemic prime is a significant stress‐increasing risk of inadequate myocardial protection. Gentle surgical handling of the heart by the primary surgeon and assistants is also felt to play a role, with excessive traction felt to disrupt the delicate fibrous skeleton of the heart, rendering less efficient the coupling of the myofibrils to mechanical force transduction of the heart. Gentle handling of the myocardium also lessens the risk of arrhythmia such as ventricular fibrillation, which on bypass represents an ischemic state of the heart. Monitoring carefully for the return of any cardiac electrical activity is important, giving consideration to more frequent cardioplegia dosing. Aggressively treating ventricular tachycardia, especially fibrillation, on bypass is very important. Meticulously maintaining venous drainage with proper cannula size selection and positioning, as well as venting of the left side of the heart prevents ventricular distension and subendocardial ischemia. Maintaining cold myocardial temperatures with ice slush application is also utilized to preserve a low‐energy consumption state. There is some evidence that volatile anesthetics can provide a degree of myocardial preconditioning when administered before CPB, in the setting of adults undergoing coronary bypass surgery. The cellular mechanisms are complex but are demonstrated to include mitochondrial and sarcolemmal potassium‐ATP channel opening, transcription factors (NF‐KB, HIF‐1 alpha), new proteins (iNOS, COX‐2), and mediators (NO, PGE, neutrophil inhibition). There is limited pediatric data, and no clinical studies have been published; hence, it is not clear whether this strategy is effective for neonatal and infant myocardial protection [89]. Two basic methods of cardioplegic arrest are used: blood cardioplegia or crystalloid cardioplegia. The superiority of either method is still controversial [90]. Histidine‐tryptophan‐ketoglutarate (HTK), an intracellular crystalloid solution, has been widely used clinically for cardioplegic arrest during cardiac surgery. The HTK solution can offer sufficient cardiac protection to the neonatal heart for up to 2 hours of ischemia and provide equivalent myocardial protection to multidose cold blood cardioplegia with reduced transfusion requirements [91]. However, clinically significant hyponatremia can occur and severe fluctuations in sodium concentration should be corrected [92]. During induction, some institutions use warm blood cardioplegia with amino acid supplementation in stressed, hypoxic hearts to recover the intracellular energy stores before arrest [93]. This results in complete preservation of myocardial function, particularly in the chronic, hypoxic heart that might become ischemic under situations of stress. Del Nido Cardioplegia solution has been increasingly used in congenital heart surgery in the past decade [94]. It is a 1 : 4 by volume blood: Crystalloid (Plasmalyte‐A) solution containing potassium (~26 mEq/L), mannitol (~3.3 g/L), magnesium (~2 g/L), sodium bicarbonate (~13 mEq/L), and lidocaine (~130 mg/L). This solution is cooled and delivered at 8–12 °C, producing myocardial hypothermia to reduce oxygen consumption and promote myocardial preservation. The 20% by volume fully oxygenated patient blood supports aerobic metabolism for a finite period and also supports anaerobic glycolysis by providing buffering properties. Mannitol scavenges oxygen free radicals, reduces myocardial edema, and promotes osmotic diuresis. Magnesium acts as a calcium channel blocker, preventing the intracellular accumulation of calcium and diastolic dysfunction. Sodium bicarbonate and the Plasmalyte‐A solution maintain neutral intracellular pH. The high potassium concentration produces a reliable depolarized diastolic arrest, and lidocaine acts as a sodium channel blocker and increases the refractory period of the cardiac myocyte. Del Nido cardioplegia solution is administered antegrade as a single dose (20 mL/kg) during aortic clamping and has shown to provide safe myocardial protection with a longer period of arrest of up to 90 minutes. A dose of 10 mL/kg can be used for shorter crossclamp times of 30–45 minutes. Some centers re‐dose at 60 minutes with a full or half dose, depending on the anticipated duration of crossclamping. This allows a bloodless operative field with less interruption compared to intermittent multidose cardioplegia. Single dose cardioplegia has been shown to have a higher rate of return to spontaneous rhythm, but no significant improvement in morbidity or lower requirement in inotropic support. Surgeon preference, anticipated aortic crossclamp duration, and case complexity should determine the selection of cardioplegia when planning procedures. After aortic cross‐clamping and sequestration of the coronary circulation, cardioplegia is generally administered in an antegrade fashion into the aortic root. As the neonatal heart lacks stenotic lesions, adequate distribution is not an issue. Perfusion pressures should be in the normal range of diastolic blood pressures and should not be higher than 30–50 mmHg. Higher pressures can lead to myocardial edema, particularly in the neonatal hypoxic heart [95]. The need for multidose cardioplegia in infants is controversial [96]. Myocardial collaterals are more important in neonatal hearts and can quickly lead to rewarming of the myocardium. Profound hypothermia or the reduction of flow can only provide limited additional protection, as other vital organs can be compromised (brain, kidney). Periodic reapplication of blood cardioplegia at intervals of 10–20 minutes counteracts non‐coronary collateral washout. Retrograde cardioplegia is utilized by some surgeons in selected situations, including cases that include an open aortic root and coronary artery translocations or transfers [97]. These include Ross procedures, arterial switch operations, and aortic root replacements. Retrograde cardioplegia is also used in some cases of severe aortic insufficiency where the ability to arrest the heart with standard aortic root cardioplegia is impaired. A small cannula, i.e. 6 Fr., is placed under direct vision into the coronary sinus, and cardioplegia is infused at pressures not exceeding about 50 mmHg. Because pediatric patients rarely have coronary stenosis, retrograde cardioplegia is used infrequently, and many surgeons prefer to avoid it altogether. Instead, the aortic root is opened immediately after cross‐clamping, and cardioplegia solution is infused directly into each individual coronary artery ostia with special cannulae. Reperfusion is considered the phase when the coronaries are reperfused with regular systemic blood flow after cross‐clamp removal and the patient is fully warmed. A few minutes before cross‐clamp removal, lidocaine and magnesium sulfate may be administered to reduce the incidence of ventricular arrhythmias often seen after unclamping. Before aortic cross‐clamp removal, TEE can be used to assist the surgeon in de‐airing maneuvers, which may include manipulation of the heart and “milking” any intraventricular air through the aortic valve and out of the cardioplegia cannulation site. The patient may be positioned in Trendelenburg position before cross‐clamp removal to avoid air bubbles being ejected into the cerebral circulation. This period is an important time in the course of cardioplegic arrest, as many mechanisms of cellular damage are completed during reperfusion. Optimally, normal sinus rhythm and myocardial contractility are restored during this time, while the patient is slowly rewarmed. In adults, reperfusion with warm blood before unclamping the aorta improved metabolic and functional recovery. Substrate‐enriched reperfusion with the amino acids aspartate and glutamate, however, results in full recovery of function in infants [98]. Depending on the total ischemic time of the heart (aortic cross‐clamp time), the heart requires time to restore the ATP stores in the myocardium. In general, 10–15 minutes are considered the minimum time requirement. For longer cross‐clamp times, 25% of the time is considered appropriate as reperfusion time at our institution. Some centers administer calcium chloride 5–10 mg/kg into the CPB circuit 10–15 minutes after aortic unclamping to counteract the effect of higher magnesium concentrations from cardioplegia washout. The release of the cross‐clamp often leads to a drop in blood pressure due to the higher release of anaerobic metabolites from the heart which causes vasodilation to a greater degree compared with the adult. This hypotension should not be treated by the administration of calcium at this point to reduce the risk of immediate reperfusion injury. In addition, at this time air can be ejected into the right coronary artery, resulting in acute ischemia with ST‐segment elevation and poor myocardial contractility; this is best treated with higher perfusion pressures and adequate time on CPB to allow passage of air through the coronary system and recovery of the myocardium. Calcium chloride 10–20 mg/kg can be administered to the CPB circuit or to the patient immediately before separating from bypass to correct the slight hypocalcemic state of CPB and improve myocardial contractility, but it should be avoided during the first 10–15 minutes of reperfusion of the heart. Additional doses of calcium are added to maintain normal levels of ionized calcium after bypass in neonates and infants, particularly as calcium and other electrolytes are lost quickly through the use of modified ultrafiltration and the infusion of citrate‐rich blood products. During rewarming, surgery is completed, and inotropic and vasoactive agents are started, but only after the ECG and myocardial contractility have improved to near normal. The rationale is that increasing myocardial oxygen demand too early in the post‐ischemic heart may be detrimental. Ventilation commences after manual ventilation lung recruitment maneuvers, administration of nebulized β‐agonist agents, if indicated, and thorough suctioning. The lungs are inspected visually if the pleura have been opened for complete inflation and deflation and any areas of atelectasis are addressed with inspiratory holding breaths near the patient’s vital capacity. Severe ventilation problems may rarely need to be assessed with fiberoptic bronchoscopy for accurate assessment of endotracheal tube placement, and further suctioning of secretions. Hemofiltration and blood transfusion are used to achieve the desired Hct. Pressure transducers are re‐zeroed and leveled. Left atrial and/or PA monitoring lines, if indicated, are placed at this time, as are temporary atrial and/or ventricular pacing wires. Forced air warming devices, circulating water mattresses, and ambient temperature are adjusted to desired levels. If the patient is incompletely rewarmed before separation from CPB, a significant temperature drop with the precipitous post‐bypass reduction in core body temperature can occur (“after‐drop”). This may lead to vasoconstriction, shivering, increased oxygen consumption, and acidosis. However, post‐ischemic hyperthermia can lead to delayed neuronal cell death and predispose to arrhythmias such as junctional ectopic tachycardia [99]. Mild degrees of hypothermia and certainly the avoidance of hyperthermia are essential in the perioperative period [100]. In the pediatric patient group, rectal temperature mostly reflects the peripheral temperature. Several endpoints have been proposed, such as nasopharyngeal temperatures greater than 35.0 °C, bladder temperature greater than 36.2 °C [101] or skin temperatures greater than 30 °C [102]. We use an endpoint of 35.5 °C rectal temperature, which is supported by the literature [103]. Cardiac rhythm is carefully assessed, and if the desired sinus rhythm at an adequate rate has not been achieved, atrial and/or ventricular pacing are instituted. If arrhythmias occur that have the potential to compromise hemodynamic status after CPB, they are treated before separation with pharmacologic approaches or internal cardioversion. Finally, blood gas values are measured before weaning from CPB to optimize electrolytes and Hct. Once the patient is ventilated, warm and in a stable rhythm, in addition to all the post‐CPB requirements being met, weaning is initiated by slowly decreasing the venous return. Arterial perfusion is continued until the appropriate filling is reached. If all parameters are stable, modified ultrafiltration is started. The heart is observed carefully during this process to avoid overfilling or to recognize right heart failure early on. Also, radial artery pressure may not be accurate following CPB and tends to underestimate both the systolic and mean central aortic pressures. A questionable pressure should be confirmed with central aortic pressure measured from the aortic cannula. A pulse oximeter waveform appearing immediately after termination of CPB is a sign of good peripheral perfusion and adequate rewarming. There may be a larger arterial–alveolar gradient between end‐tidal carbon dioxide and arterial carbon dioxide tension at the end of bypass due to ventilation‐perfusion mismatch. A rapidly increasing area under the curve of the capnograph and increasing end‐tidal CO2 are signs of good cardiac output and the pulmonary status during the termination of CPB. TEE and/or epicardiac echocardiography are essential to evaluate the cardiac repair. TEE is used prior to separation to assist in de‐airing the heart. After separation from bypass, it has become standard of care for most institutions and operations to evaluate heart function, assess the repair and any residual lesions prior to removing the cannulas. The recently published Residual Lesion Score (RLS) Study evaluated 1,149 infants undergoing five common procedures: tetralogy of Fallot, complete atrioventricular canal (CAVC), arterial switch, coarctation or arch interruption with ventricular septal defect (VSD), and Norwood stage I palliation [104]. RLS was assessed on postoperative echo and graded as 1 (trivial or no residual lesions), 2 (minor residual lesions), or 3 (major residual lesions with/without reintervention before discharge). RLS 3 was present in 15–35% of patients depending on the operation. Patients with RLS 3 for any lesion were significantly more likely to suffer adverse outcomes than RLS 1 (fewer days alive and out of hospital), and other major outcomes were worse with RLS3 for all lesions except TOF. (Total ventilation time, total ICU stay, major medical events including cardiac arrest, ECMO cannulation, neurologic event, and others) (Figure 11.9A). This important study emphasizes the importance of the immediate post‐bypass TEE/epicardial echo, and the contributions of the entire intraoperative team to decision making about immediate reinstitution of bypass and revision of the surgical repair. See Chapter 15 for further details about TEE and epicardial echocardiography. The application of the CPB machine in children leads to a significant capillary leak more often than is the case in adults. This is caused by the relatively larger foreign surface area exposure and the resulting greater inflammatory response. Hemofiltration has been defined as ultrafiltration with the return of intravascular replacement fluids to compensate for losses. In contrast, ultrafiltration simply removes fluid through a convective process involving filtration across membranes. Conventional ultrafiltration (CUF) during CPB or modified ultrafiltration (MUF) after the end of bypass both reduce levels of proinflammatory cytokines and total interstitial body water after extracorporeal circulation. CUF and/or MUF are therefore standard procedures in all pediatric perfusion systems. The most commonly used procedure is MUF first described by Naik et al. [105, 106]. In this case, the bypass circuit is modified, and the flow is reversed at the end of CPB before protamine is given. Blood from the aortic cannula is directed through a hemofilter (blood flow rate of 100–300 mL/min) and infused back into the right atrium after warming and oxygenation. The ultrafilter pump is run at 10–30 mL/kg/min ultrafiltrate removal, with a vacuum on the ultrafilter. Replacement intravascular volume is infused from the venous reservoir as necessary. A steal phenomenon has been described whereby excessive flow rates divert blood from the aorta to the hemofilter, leading to cerebral and systemic deoxygenation. Several different methods of deciding when to terminate MUF are employed; some surgeons simply use a cutoff time of 15–20 minutes and others stop when the circuit volume has become diluted or when the desired Hct has been reached. Frequently, the surgeon’s patience is the limiting factor. Filtering also will be stopped if the patient becomes hemodynamically unstable. Multiple beneficial effects can be observed (see Table 11.5); particularly important are the increase in Hct, the reduction of cytokines, improved myocardial perfusion, and a reduction in pulmonary vascular resistance (PVR) with the improvement of right heart function. Disadvantages of MUF are a delay in heparin reversal and decannulation of approximately 10–20 minutes, as well as the possibility of hemodynamic instability if preload is not adequately maintained by the perfusionist. However, surgical hemostasis can be carried out during this time period. The combination of MUF after CPB with zero balance conventional ultrafiltration (ZBUF) on bypass can eliminate additional unnecessary volume from cardioplegia or irrigation. The use of filtration during CPB (as conventional, dilutional, or zero balance ultrafiltration) also removes inflammatory mediators and vasoactive substances [107, 108]. Studies [109] have shown that compared with control patients, patients who have modified ultrafiltration after bypass have substantially less increase in total body water, have less interleukin‐8 (IL‐8) and complement in their bloodstream [110, 111], require less blood transfusion [112, 113], show improved coagulation factors [114, 115] and faster recovery of systolic blood pressure [105], pulmonary function [116], and cerebral metabolic activity [117] (Table 11.5). Modified ultrafiltration performed after CPB reverses hemodilution and decreases tissue edema and thereby accelerates postoperative recovery [118]. The combined use of ultrafiltration of prime solution, zero‐balance ultrafiltration on bypass, and MUF strategy seems to be the most effective method, even though the principal clinical outcomes are similar between modalities [119]. A recent review of 90 clinical studies of the various forms of ultrafiltration concluded that MUF resulted in improved myocardial function, reduced fluid overload, and reduced bleeding and transfusion complications [120]. Zero‐balance ultrafiltration (ZBUF) has been consistently associated with a reduction in inflammatory cytokines and improved pulmonary function and compliance. There is mixed evidence that any UF approach can reduce ventilation time and ICU stay. Because of the heterogeneity of the studies, no single approach was recommended. Figure 11.9 (A) Boxplot of days alive and out of hospital by RLS Score (higher number is better). The p values are from the Kruskal‐Wallis Test. The number and percentage of patients in the 3 RLS classes are provided below the boxplot. TOF, tetralogy of Fallot; CAVSD, complete atrioventricular septal defect; ASO, arterial switch operation; Arch/VSD, aortic arch repair with ventricular septal defect; Norwood, Norwood stage I palliation. (B) Incidence of major medical events. P values are from Fisher’s exact test. Includes mortality or heart transplant, cardiac arrest, ECMO cannulation, cardiac failure, mediastinitis, bleeding, neurologic event, renal failure, necrotizing enterocolitis, diaphragm or vocal cord paralysis, prolonged ventilation, sepsis, multisystem organ failure, noncardia operation, hemothorax, and readmission. (Source: Nathan et al. [104]. Reproduced with permission of Elsevier.) Table 11.5 Effects of modified ultrafiltration Source: Elliott et al. [109]. Reproduced with permission of Elsevier. CO, cardiac output; PAP, pulmonary artery pressure; PVR, pulmonary vascular resistance; SV, single ventricle; SVR, systemic vascular resistance; V/Q, ventilation‐perfusion. Occasionally, despite escalating inotropic support, a child is unable to maintain adequate cardiac output and systemic oxygen delivery and therefore a return to CPB must be considered. An assessment of arterial blood gas and Ca2+ values, and whether intended inotropes are infusing correctly is important. Immediate TEE evaluation should be performed for the possibility of residual defects that require surgical attention. If no further surgical intervention is warranted, the source of the difficulty in weaning from CPB should be sought and other therapies must be considered. Is the Hct level adequate for this child? Too much hemodilution can lead to decreased systemic vascular resistance (SVR). The ideal Hct depends on the pathology and is probably in the range of 35–45% for complex repairs. Is the SVR too low? In children with low SVR who are either non‐responsive to catecholamine infusions or who are experiencing adverse effects due to catecholamine therapy, arginine vasopressin has been shown to be a potent vasoconstrictor, with infusions resulting in increased MAPs and decreased catecholamine dependence. Its use in children appears promising when cardiac function is adequate [121, 122]. Fixed doses of 0.01–0.04 units/kg/hour of vasopressin are used. Exposure to endotoxin and cytokines as on CPB can trigger a de novo synthesis of the inducible, calcium‐dependent isoform of nitric oxide synthase. Methylene blue inhibits this process by decreasing intracellular cyclic guanosine monophosphate concentrations through guanylate cyclase inhibition, thus blocking its vasodilator properties. It increases arterial pressure, SVR, and left ventricular stroke work, but does not increase cardiac output, oxygen delivery, or oxygen consumption. Methylene blue in a dose of 2 mg/kg followed by 1 mg/kg/hour has been used successfully in the setting of perioperative vasoplegia in adults [123], and neonatal sepsis [124], and in a case report of infective endocarditis in a 10‐year‐old girl [125]. Side‐effects are rare in doses <2 mg/kg, but pulmonary hypertension and other side‐effects can occur with repeat doses [126]. Is the right heart failing? Pulmonary hypertension with resultant right heart failure may occur post‐CPB, either as a result of long‐standing increases in PA pressures or PVR, now exacerbated by the effects of CPB or as a result of acute increases in PA pressures or PVR secondary to protamine administration. Management has been challenging, as IV medications often affect both SVR and PVR. Selective pulmonary vasodilatation became possible with the introduction of inhaled nitric oxide (iNO), an endothelium‐derived vasodilator that is rapidly deactivated by hemoglobin [127]. Several groups of patients have been shown to potentially benefit from iNO administration post‐CPB [128, 129]. Patients with single‐ventricle physiology undergoing total cavopulmonary anastomosis (Fontan procedure) with elevated PVR post‐CPB, as well as children with elevated pulmonary venous pressures secondary to total anomalous pulmonary venous connection or congenital mitral stenosis, frequently show improvement with administration of iNO. For patients on iNO, caution should be exercised when transporting from the operating room to the ICU in order to avoid interruption of therapy, as rebound pulmonary hypertension and rapid clinical deterioration may occur. Other options to lower PVR include sildenafil 0.5 mg/kg through a nasogastric tube [130], IV sildenafil, or nebulized prostacyclin [131]. If severe left or right ventricular dysfunction persists in the absence of residual anatomic defects that can be surgically corrected, consideration may be given to continued mechanical support of the circulation. Currently, immediate pediatric options for mechanical circulatory support are extracorporeal membrane oxygenation or short‐term left ventricular assist device in neonates, infants, and children. These techniques are most useful when recovery of ventricular function is expected within 24–72 hours, or when cardiopulmonary support is unavoidable. Cannulation configurations are shown in Figure 11.10 [132]. A recent review of postcardiotomy veno‐arterial ECMO of more than 40 studies reporting more than 3,000 patients from 1990–2018 indicated a post‐ECMO survival to hospital discharge of 40–60% in most studies [132]. Duration of ECMO support for survivors tended to be 3–5 days, and major complications included bleeding (13–69%), neurologic morbidity (11–51%), sepsis (10–50%), and renal failure (9–56%). Greater than 95% of reports of postcardiotomy mechanical support are ECMO. Veno‐venous ECMO can be utilized for isolated respiratory failure. Another approach is short‐term VAD support; Poh and colleagues reported 55 single ventricle patients supported with centrifugal VAD for postcardiotomy failure [133]. Median support was 3.5 days, and 53% were weaned off support, but only 33% survived hospital discharge. See Chapter 37 for further details about mechanical support. During modified ultrafiltration, cardiac function and the quality of the surgical repair are assessed via TEE, and if found to be satisfactory, protamine is administered to neutralize residual heparin after completing MUF. In small infants and palliated anatomies, the goal Hct separating from bypass should be 40–45%. This improves hemodynamic stability and allows immediate correction of coagulation disorders by infusion of blood products. Protamine 1–1.5 mg/kg is given for each 100 units of the initial heparin dose to reverse its effect. This assumes that any further doses are given to maintain a heparin level and prevent overdosing of protamine with its associated effects on platelet function (reduction of the interaction of the glycoprotein Ib receptor with von Willebrand factor) [134]. If the ACT is still elevated or CPB prime (heparinized) blood is reinfused into the patient, an additional 25% of the initial dose of protamine is added and the ACT rechecked. However, particularly in infants, the administration of protamine and the persistent treatment of a suspected incomplete heparin reversal should not distract and delay the treatment of other commonly associated post‐bypass coagulopathies such as thrombocytopenia, platelet dysfunction, and other coagulation factor deficiencies [135–138]. Individualized management of anticoagulation and its reversal results in less activation of the coagulation cascade, less fibrinolysis, and reduced blood loss and need for transfusions [58], while improving the clot stability as assessed by thromboelastography. However, care should be taken not to overdose the total amount of protamine [139, 140]. After one‐third to one‐half of the planned protamine dose is administered, blood from the surgical field must not be returned to the cardiotomy reservoir to avoid circuit thrombosis in case it is necessary to reinstitute bypass. An ACT or heparin level confirms adequate heparin neutralization. More protamine (0.5–1 mg/kg) can be given if either test remains prolonged and bleeding is a problem. Hypotensive protamine reactions can occur when protamine complexes with heparin because complement is released. This is much less common in children than in adults. Hypotension can be attenuated by adding calcium chloride (2 mg/mg protamine). Pediatric protamine reactions are rare, occurring in 1.7–2.8% of patients undergoing CPB [141]. Life‐threatening reactions to protamine represent true allergic or pulmonary hypertensive reactions. Protamine reactions should be treated with epinephrine as the first‐line agent and careful volume resuscitation as required. For severe reactions, it may be necessary to readminister heparin and resume CPB. Hemostatic management can be guided by thrombelastography. Newer devices allow a full “point‐of‐care” functional assessment of the coagulation within 10–15 minutes [142]. A recent retrospective study of 100 infants assessed before and after a heparin–protamine titration system (Hepcon®, Medtronic Corp. Minneapolis, MN, USA) reported that more limited protamine dosing neutralized heparin but also prevented anticoagulation effects (prolonged R values on thromboelastography [TEG]) caused by excessive protamine [139]. Further details can be found in Chapter 16. Bleeding is more common in pediatric cardiac surgery patients than in adults. Both qualitative and quantitative abnormalities in coagulation proteins have significant functional sequelae, which influence the hematologic responses to CPB. Cyanotic CHD itself is associated with coagulation abnormalities, including platelet abnormalities and fibrinolysis. There is extensive published research on the use of aprotinin and lysine analog antifibrinolytics to modify the adverse effects of CPB in adults, but for pediatrics, their dose and effects are much less clear. Figure 11.10 (A) Central ECMO cannulation via aorta and right atrium with the open sternum. (B) central cannulation via the aorta and right atrium with closed sternum and subxiphoid cannulae exit. (C) Peripheral ECMO cannulation via carotid artery and internal jugular vein with the closed sternum. Left atrial decompression may also be required with direct left atrial cannulation, atrial septostomy, or other methods. Also, if systemic to pulmonary artery shunt is present, it is usually partially occluded with a clip to limit pulmonary blood flow as in (A) and (B). (Source: Lorusso et al. [132]. Reproduced with permission of Elsevier.) Two agents are currently available to modify the hemostatic response to CPB: ε‐aminocaproic acid (EACA) and tranexamic acid (TA). Aprotinin, a serine protease inhibitor with anti‐inflammatory properties, was withdrawn from the market in 2007 due to safety concerns in adult patients and will not be discussed further. Both EACA and TA appear effective in reducing bleeding and transfusion in cyanotic patients, provided an adequate dose is administered. Their efficacy in other high‐risk and mixed populations is not as well established. These drugs seem to lack significant clinical anti‐inflammatory efficiency beyond their effects on coagulation [143, 144]. Suppression of excessive plasmin activity may play an important role in the generation of proinflammatory cytokines during and after CPB [145]. For an excellent and detailed recent review, please refer to Eaton [146]. Chauhan et al. [147], in a dose‐ranging study of TA published in 2004, found the most effective dosing scheme of the four studied to be a 10 mg/kg load, 10 mg/kg in the pump prime, and 10 mg/kg after protamine. More recent pharmacokinetic studies have shown effective concentrations of 20 μg/mL [148] using a dosing schedule with 6.4 mg/kg followed by 2–3.1 mg/kg, depending on weight [149]. Another pharmacokinetic‐based scheme specifies for neonates 0–2 mo.: 15 mg/kg bolus; 20 mcg/mL of CPB prime; 2.5 mg/kg/hour infusion; for infants 2–12 mo.: 9 mg/kg bolus; 20 mcg/mL of CPB prime; 2 mg/kg/hour infusion; and children >12 mo.: 4 mg/kg bolus; 20 mcg/mL of CPB prime; 2 mg/kg/hour infusion [150]. Continuous and discontinuous doses of TA effectively inhibit fibrinolysis [151]. A high‐dose regimen leading to blood concentrations greater than that required for D‐dimer inhibition may be more efficient than lower dosages [152]. Previous studies have described TA doses of up to 100 mg/kg as a loading dose in infants and children; reports of seizures associated with high dose TA in adults combined with the pharmacokinetic data cited above argue against these doses and they should not be used in children. [153] Based on a pharmacokinetic study [154], in neonates, an initial loading dose of 40 mg/kg, an infusion of 30 mg/kg/hour, and a pump prime dose of 0.1 mg/mL of prime will maintain plasma concentrations above 50 mg/L in 90% of neonates. In older patients, the initial loading dose is 75 mg/kg, a pump‐priming dose of 75 mg/kg followed by an infusion of 75 mg/kg/hour to establish and maintain a therapeutic plasma concentration (130 μg/mL) in 95% of patients [155]. Rapid IV injection of TA or EACA may cause hypotension. We infuse loading doses over approximately 20 minutes before the surgical incision. Some institutions delay antifibrinolytic dosing until the institution of bypass in patients who have systemic to pulmonary artery shunts, citing the risk of thrombosis. With the considerable variability among studies of the three drugs under consideration in terms of design, dose, and outcomes, it is difficult to draw any conclusions about relative efficacy from the literature. There are a few published comparison studies. Chauhan et al. [156] compared low‐dose aprotinin, EACA, and the combination in 300 cyanotic patients undergoing cardiac surgery. There was no difference between EACA‐treated and aprotinin‐treated patients in any measured variable. The same group compared TA and EACA in a placebo‐controlled study of 150 patients with cyanotic CHD [147]. Both drugs were superior to placebo, but there were no significant differences between the treated groups with respect to 24 hours blood loss, transfusion, or re‐exploration rate. Finally, the effects of aprotinin and TA were compared in 100 children, evenly divided into four groups: placebo, TA, aprotinin, and a combination of the two drugs [157]. Again, all treatment groups fared better than placebo in 24 hours blood loss and transfusion, with no significant differences among the three treated groups. Thus, the limited comparative evidence would suggest that the three drugs are equivalent in efficacy for reduction of bleeding and transfusion, at least with the doses and patients studied, and there is little or no advantage to combination therapy. In summary, evidence supports the efficacy of the lysine analog antifibrinolytics EACA and TA, and aprotinin to decrease bleeding and transfusion in pediatric patients undergoing cardiac surgery involving CPB. This benefit is likely to be more significant in high‐risk groups, such as cyanotic patients, newborns, complex surgeries, and reoperations. Aprotinin seems to have the strongest anti‐inflammatory capacity of the antifibrinolytics [158]. The safety profile of these drugs is not fully understood, but each may have significant side effects that require further research in the future [159–161]. Further discussion of antifibrinolytic therapy is presented in Chapter 16.
CHAPTER 11
Cardiopulmonary Bypass
Introduction
Basic bypass circuit setup
Parameter
Adult
Pediatrics
Temperature
Rarely below 32 °C
Commonly 18–20 °C
Deep hypothermic circulatory arrest (DHCA)
Rare
Common for arch repair (HLHS, IAA)
Pump prime
Crystalloids
Blood components and albumin
Dilution
25–33%
Up to 200%
Perfusion pressure
50–80 mmHg
30–50 mmHg
Flow rates
2.5 L/min/m2 or 50–65 mL/kg/min
0–250 mL/kg/min
pH management
Alpha‐stat
pH‐stat
Hypoglycemia
Rare, only in hepatic injury
Common due to low hepatic glycogen stores
Hyperglycemia
Frequent increases mortality
Less common, may be protective
Cannulation techniques
Standardized, mostly ascending aorta and single‐stage venous cannula
Variable, including ductus, aorta, main pulmonary artery; mostly bicaval venous cannulation
Ultrafiltration
Rare
Standard modified ultrafiltration or conventional ultrafiltration
Cannulation and tubing
Tubing diameter (in.)
Volume per meter length (mL/m)
1/2
126
3/8
71
1/4
33
3/16
18
Arterial and venous tubing sizes
Patient weight
3/16 in. arterial line, 1/4 in. venous line
<10 kg
1/4 in. arterial line, 3/8 in. venous line
<20 kg
3/8 in. arterial line, 3/8 in. venous line
<50 kg
Pumps
Oxygenator
Priming
Weight (kg)
Estimated blood volume (mL/kg)
<3 (neonate)
90
3–10
85
10–20
80
20–30
75
30–50
70
>50
65
Differences between pediatric and adult CPB
Hemodilution
Perfusion pressures
Flow rates
Blood gas management
Aortopulmonary collaterals
Temperature ranges
Glucose management
Equipment
Ultrafiltration
Management of pediatric CPB
Stages of CPB
Pre‐bypass period
Anticoagulation and hemostatic management
Initiation of CPB and flow requirements
Cooling and temperature management
Aortic cross‐clamping, myocardial ischemia, and protection
Induction and maintenance of cardioplegic cardiac arrest
Reperfusion
Separation from CPB and post‐bypass phase
Conventional ultrafiltration and modified ultrafiltration
Parameter
Effect
PVR, PAP
Decreases due to oxygenation and warming
Stroke volume, CO
Left ventricular stroke volume increases secondary to improved pulmonary blood flow
SVR, blood pressure
Increases due to the reduction of vasoactive substances (interleukins, bradykinin, etc.)
Hemoglobin, hematocrit
Increases due to hemoconcentration
Interstitial body and lung water
Decreases secondary to water removal
Improved gas exchange [79]
Improved V/Q mismatch
Failure to separate from CPB
Heparin reversal and transfusion management
Antifibrinolytic therapy
Epsilon‐aminocaproic acid (EACA) and tranexamic acid (TA)
Tranexamic acid dosing
EACA dosing
Comparison studies of antifibrinolytics
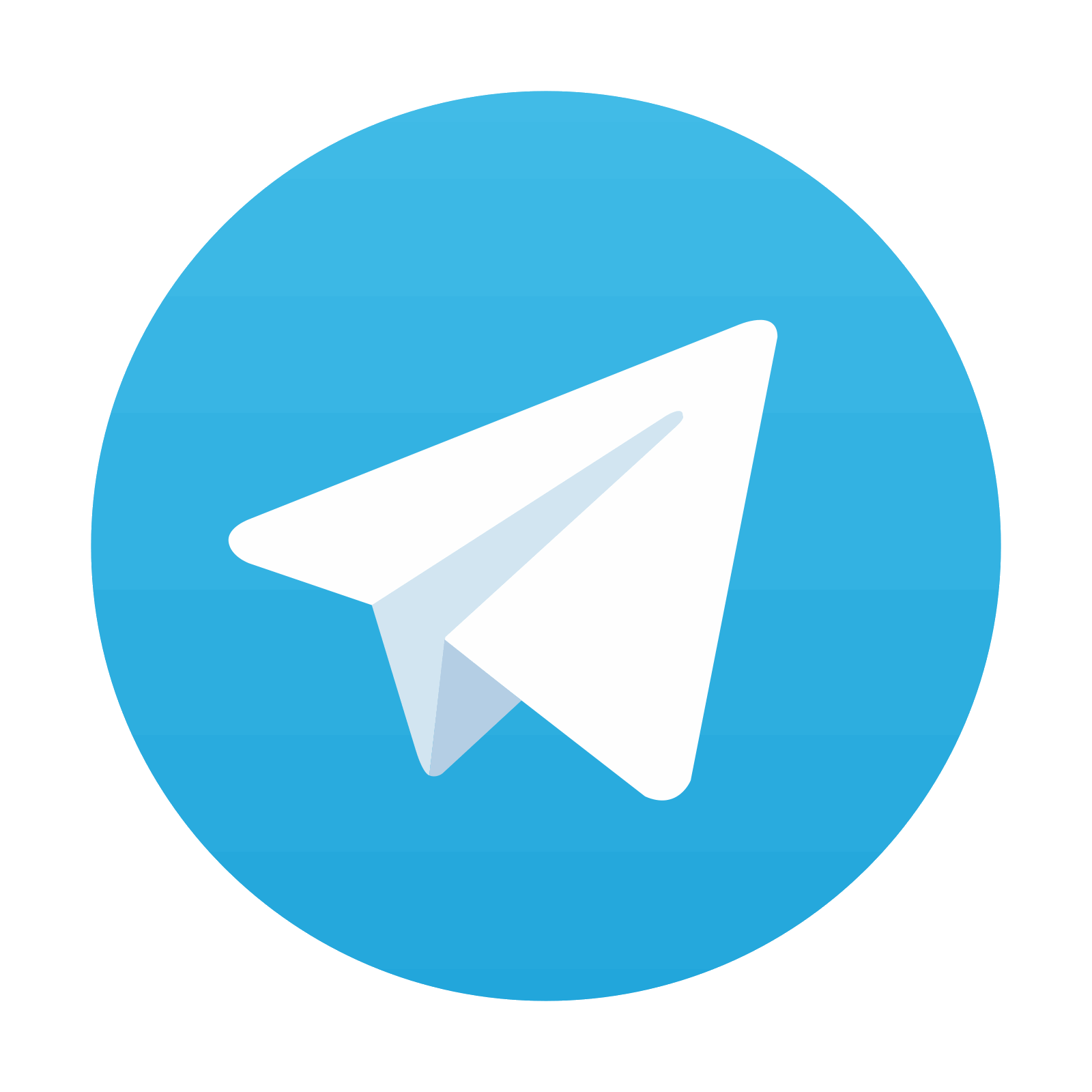
Stay updated, free articles. Join our Telegram channel
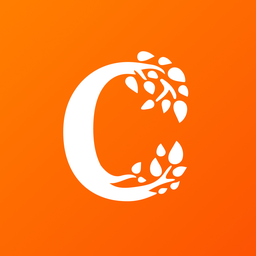
Full access? Get Clinical Tree
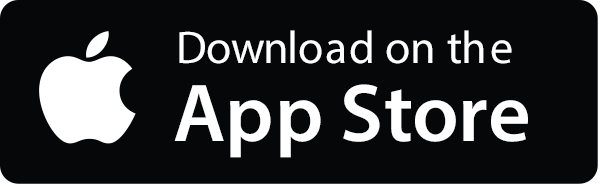
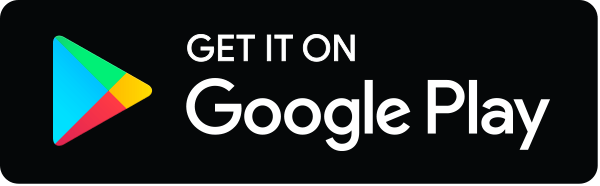