22 Dan Miulli and Javed Siddiqi The patient has a Glasgow Coma Scale (GCS) score of 6: 3 for decorticate/flexor posturing, 2 for incomprehensible sounds, and 1 for not opening his eyes. The definition of severe head injury is a GCS of 8 or less and according to Guidelines for the Management of Severe Traumatic Brain Injury,1 initial management and resuscitation should include intubation. Intubation may help prevent significant secondary brain injury from hypoxia, which is oxygen (O2) saturation <90%, partial pressure of oxygen in arterial blood (PaO2) <60 mm Hg, apnea, or cyanosis. Stocchetti et al2 demonstrated that hypoxic patients without hypotension had 3 times the mortality rate and 20 times the severe morbidity rate of nonhypoxic patients. Furthermore, prehospital intubation of severe trauma victims significantly reduced mortality.3 Systematic withholding of endotracheal (ET) intubation in patients with severe head injury is not recommended. Careful and rigorous neurologic examination, including assessment of brainstem reflexes, might help to identify patients with a very high probability of death despite mechanical ventilation.4 Considerable national variation in the care of severely head-injured patients still persists, even though Guidelines for the Management of Severe Traumatic Brain Injury1 were first published in 1995. Those that actively follow these management strategies have an associated decreased mortality rate for patients with severe head injury, with no significant difference in functional status at discharge among survivors.5 In addition to data supporting intubation for severe head injury, the literature supports intubation for stroke as well. However, intubation in the face of stroke still remains somewhat controversial. Magi et al6 concluded that the likelihood of deterioration is high in acute stroke patients who present to hospital intubated and ventilated is high. However, the observed survival rate is sufficient to justify this treatment, even in cases not requiring other invasive procedures, such as neurosurgery and angiography. The severity of the ischemic event often leads to mechanical ventilation and, in two thirds of those individuals, death in the hospital; the remainder are severely disabled. In a study of a multiethnic urban population, survival was unlikely if patients were deeply comatose or deteriorated clinically after intubation. However, the authors concluded that mechanical ventilation for stroke was relatively cost effective for extending life but not for preserving quality of life.7 The brain and spinal cord require O2 and blood flow. Ischemia must be prevented at all costs. Depending on the severity and onset, in the absence of O2, glucose and glycogen are depleted in 4 minutes. In addition to the general lack of energy substrates, there is a demand for additional energy from the injured brain, as demonstrated by an increased metabolism in the layer surrounding the penumbra and increased brain temperature. When the brain tissue partial pressure of oxygen (PO2) is <20 mm Hg, anaerobic respiration becomes the predominant energy exchange system. This leads to glucose store–dependent lactic acidosis, decreased pH, vasodilation, mitochondrial damage, and cell death. Guidelines for the Management of Severe Traumatic Brain Injury1 addresses blood pressure and oxygenation as follows: Hypotension ([systolic blood pressure, SBP] < 90 mm Hg) or hypoxia (apnea or cyanosis in the field, PAO2 < 60 mm Hg, saturation < 90%) must be scrupulously avoided, if possible, or corrected immediately. Prior to intubation, the patient is preoxygenated with 100% O2 to maintain the arterial O2 (O2 saturation) at the highest level. Maintain cervical spine precautions by continued use of a cervical collar. Use a jaw thrust technique to keep the patient’s airway open for oxygenation. Do not use a head tilt technique in a patient with any suspected spinal injuries. After suctioning the upper airway, maintain spinal immobilization and begin rapid sequence intubation with an in-line orotracheal tube, a blind nasotracheal tube, an esophageal-tracheal Combitube (Kendall-Sheridan Corp. Argyle, NY), or a laryngeal mask airway. The equipment must be available, and tube cuffs must be checked prior to delivering the paralytics. If tracheal intubation cannot be performed because of facial fractures, then intubation after cricothyrotomy or tracheotomy must be considered. The steps in rapid sequence intubation are as follows: Premedication/induction/sedation in head injury patients may prevent the increased ICP that can occur from pain or suctioning. Use drugs depending on the time requirement for intubation and the need to assess the patient via another neurologic exam after intubation (Table 22–1). Paralytic prophylaxis prior to succinylcholine or a paralytic in place of succinylcholine is summarized in Table 22–2. Remember that, after intubation, the patient will need to have a neurologic exam. Sometimes the patient may need to be examined for brain death, for example, when the intubation was a reaction to a code. It is best to decide the neurologic status prior to intubation. However, the GCS should be determined after (not in place of) resuscitation (Table 22–2). The side effects of commonly administered drugs include the following: Ochs et al9 evaluated the ability of paramedic rapid sequence intubation to facilitate intubation of patients with severe head injuries in an urban out-of-hospital system. They examined adults with severe head injuries who were more than 10 minutes away from the hospital when injured. The patients were premedicated with midazolam, paralyzed with succinylcholine, and given rocuronium after tube placement was confirmed. The Combitube was used as a salvage airway device. Outcome measures included intubation success rates, preintubation and postintubation O2 saturation values, arrival arterial blood gas values, and total out-of-hospital times for patients intubated en route versus on scene. Of the 114 enrolled patients, 84% underwent successful ET intubation, and 15% required Combitube intubation, with only 1 (0.9%) airway failure. There were no complications, and blood gases were superb with paramedic-performed rapid sequence intubation of patients with severe head injuries. Emergent cricothyroidectomy should only be performed by individuals familiar with the anatomy after unsuccessful or unavailable ET intubation in an upper airway obstruction. The procedure requires a knife blade and handle, antiseptic solution, and a tracheostomy tube, a pediatric ET tube, or a 14-gauge catheter-over-needle. The steps in an emergent cricothyroidectomy are as follows: The cricothyrotomy may be converted to a tracheostomy under controlled conditions in the operating room once the airway has been secured. Tracheostomies are not appropriate procedures for urgent situations.10 Once a patient in a coma from stroke or head injury is intubated, O2 and CO2 in the patient without known chronic obstructive pulmonary disease (COPD) should be kept in a specific range for the initial period of 24 hours. Oxygen partial pressure should be maintained at ~115 mm Hg and CO2 at ~35 mm Hg. Brain tissue oxygenation or the related cerebral perfusion pressure depends on cerebral blood flow. Therefore, treatment modalities that cause vasoconstriction and decrease cerebral blood flow, such as aggressive hyperventilation, should be questioned and modified in the management armamentarium. Hyperventilation works by decreasing plasma PCO2, which causes an increase in the ambient pH in or around the brain cerebrovasculature, leading to cerebral vasoconstriction, decreasing the cerebral blood flow, and, in some cases, decreasing ICP. Soon after closed head injury, cerebral blood flow is low. During the first day after injury, it is less than half that of normal individuals; it then increases for at least 3 days, except in those patients who have uncontrollable ICP. Cerebral blood flow has been measured with hyperventilation, and there are significant differences in and around contusions, including local variability secondary to hyperventilation-induced steal. Cerebral blood flow changes with hyperventilation; there is a 3 to 4% change in cerebral blood flow per millimeter change in PCO2, as demonstrated by Obrist et al.11 When cerebral blood flow is decreased, the level at which irreversible ischemia or infarction takes place is not precisely known. The same study by Obrist et al11 suggested that, in patients with severe head injuries, there is a depression of normal cerebral metabolism. Furthermore, the reduced cerebral blood flow that occurs may, in many cases, be appropriate for the metabolic needs of the brain. Cerebral blood flow is lowest in patients with subdural hematomas, diffuse injuries, and hypotension, and highest in those with epidural hematoma or normal computed tomography (CT) scans. There is a direct correlation between cerebral blood flow and GCS during the first 4 hours after injury, as demonstrated by Bouma et al.12 Therefore, the consequences of cerebral-blood-flow-reducing hyperventilation become more apparent. Aggressive hyperventilation to a PCO2 of 25 or less has been the cornerstone in the management of severe head injury for more than 20 years because it can cause a rapid reduction of ICP. However, there are no studies showing an improvement in outcome in neurologic patients with severe head injury using hyperventilation empirically. Hyperventilation reduces ICP by causing cerebral vasoconstriction and a subsequent reduction in cerebral blood flow. As stated earlier, cerebral blood flow is reduced by 50% during the first day after severe head injury; therefore, hyperventilation can only exacerbate this already deadly condition. Raichle et al13 looked at a group of healthy individuals treated with hyperventilation. When these individuals had their PCO2 decreased by 15 to 20 mm Hg from normal, there was a 40% decrease in cerebral blood flow after 30 minutes. Four hours later, despite continued hyperventilation, the cerebral blood flow increased to 90% of baseline, even though the PCO2 was still decreased, demonstrating that hyperventilation to reduce ICP may be only a temporary phenomenon. This suggests that hyperventilation, if necessary, should be used only in short bursts. In the same study, when the original PCO2 was quickly restored, the cerebral blood flow was increased to 31% above baseline. The quick restoration increases cerebral blood volume and, in turn, can increase ICP. Therefore, when elevating PCO2 from below normal levels, gradually increase the PCO2. In the autoregulatory intact individual, there is a 3 to 4% change in cerebral blood flow per mm Hg change in PCO2. This decrease in cerebral flow occurs less when cerebral blood flow is already reduced; also, the lower CO2 vasoresponsivity is associated with a poorer outcome. Local CO2 responsivity can differ from values by more than 50%. Cold et al14 demonstrated that in some patients cerebral autoregulation is preserved with normocapnia and lost with hypocapnia. Others have found varying responses with hyperventilation. Crockard et al15 found an increase in ICP associated with a decrease in PCO2, and Obrist and Martin16 found a decrease in ICP in only half of the patients treated with hyperventilation; however, over 90% of those patients also had a decrease in cerebral blood flow. In a landmark class I study, Muizelaar et al17 published the results of a prospective randomized clinical study in which 77 patients were randomized to a group treated with chronic prophylactic hyperventilation for 5 days after injury (PCO2 = 25 ± 2 mm Hg) or to a group that was kept normo-capnic (PCO2 = 35 ± 2 mm Hg). Those patients who were treated with prophylactic hyperventilation had a significantly worse outcome than those in the normocapnic group at 3 and 6 months. The researchers did find that hyperventilation may be beneficial with hyperemia, and hyperventilation is needed in signs of acute herniation. Guidelines for the Management of Severe Head Injury1 recommends the following: In the absence of increased intracranial pressure, chronic prolonged hyperventilation therapy (PCO2 of 25 mm Hg or less) should be avoided after severe traumatic brain injury. Guideline: The use of prophylactic hyperventilation (PCO2 less than or equal to 35 mm Hg) therapy during the first 24 hours after severe traumatic head injury should be avoided because it can compromise cerebral perfusion during a time when cerebral blood flow is reduced. Option: Hyperventilation therapy may be necessary for brief periods when there is acute neurological deterioration or for longer periods if there is intracranial hypertension refractory to sedation, paralysis, cerebral spinal fluid drainage and osmotic diuretics. [Jugular venous oxygenation saturation, SjvO2], [arteriovenous oxygen content difference, AVDO2] and cerebral blood flow monitoring may help to identify cerebral ischemia if hyperventilation, resulting in PCO2 that is less than 30 mm Hg, is necessary. Several studies have demonstrated that aggressive hyperventilation causes secondary brain injury from decreased brain tissue oxygenation.17–20 The literature is also replete with documentation of improved survival for severe head injury utilizing modern treatment paradigms. The majority of physicians who are exposed to head trauma patients, explicitly emergency room doctors, are managing severe head injury patients in accordance with Guidelines for the Management of Severe Head Injury,1 with the exception of avoiding prophylactic hyperventilation. It is hoped that more education and exposure to the evidence regarding prophylactic hyperventilation of severely head-injured patients may improve adherence to the hyperventilation guidelines.21 Not all facilities have the ability to directly measure cerebral blood flow; therefore, they choose to indirectly measure it using AVDO2 or SjvO2. AVDO2 is indirectly a manifestation of metabolism and cerebral blood flow. The brain, which comprises approximately 2% of body weight, uses 20% of the cardiac output and 25% of the resting body glucose. It has a very small energy reserve; therefore, metabolism is tightly coupled to cerebral blood flow. Normally, metabolism is 1.5 μg/g/minute, or 3.4 mL/100 g/minute; this is the cerebral metabolic rate of O2. Approximately 19 mL/dL of O2 is delivered to the brain on the arterial side and ~12 to 13 mL/dL of O2 on the venous side, yielding approximately 35% O2 extraction, leaving an AVDO2 of 6.5 mL/dL. The brain uses 90% aerobic and 10% anaerobic respiration. This occurs through the citric acid cycle. Approximately half the energy is used for cell maintenance and half for brain function. Normally, there is a very small amount of lactate produced. Isolated patients with severe head injuries were studied, keeping SBP, hematocrit, and temperature normal.22 These patients were divided into two groups. One had mass lesions requiring surgery, and the other did not. Both were treated according to protocol: with PCO2 > 30 mm Hg, patients were intubated, sedated, and paralyzed, and given mannitol if the ICP was elevated. Patients had jugular venous catheters inserted, and the AVDO2 was calculated before and 30 minutes after treatment. In those patients who underwent evacuation of a mass lesion, their perfusion pressure was termed adequate to prevent ischemia; however, some individuals still demonstrated infarction by CT scan. ICP and cerebral perfusion pressure (CPP) were normal. The closer the AVDO2, the less likely the chance of having an infarction. Likewise, in the nonsurgical group, those patients who developed an infarction also had adequate ICP and CPP after treatment, but had a larger AVDO2, suggesting they required more oxygen for energy maintenance. There was a change in AVDO2 in both groups that predicted the outcome. An elevated AVDO2 signifies increased O2 extraction, which is consistent with ischemia. It would seem that the failure of the AVDO2 to improve with treatment was the one factor that was consistent with infarction. The ideal monitor, therefore, would give reliable continuous readings, would be portable, and would suggest a cause and appropriate therapy. The saturation of jugular venous O2 is the reciprocal of AVDO2. If O2 extraction increases, the AVDO2 will increase, but the SjvO2 will decrease. This measurement assumes that cerebral metabolism and hemoglobin are constant, and the effective dissolved O2 is negligible. There are limitations to SjO2 measurements. It is contraindicated with C-spine injuries, coagulopathy, and tracheostomies. It measures hemispheric flow, which may not be constant from one side to the other, and even on one side will give measurements of general hypoxia but will not allow region-specific treatment. Changes in SjO2 can occur after herniation has taken place. There are artifacts associated with catheter movement; placement has to be precise, not too proximal, and not against a vessel wall. SjO2 < 50% is consistent with ischemia. Profound or prolonged episodes of desaturation are associated with a poor outcome. Desaturations are most common with low cerebral blood flow; SjO2 < 50% is the equivalent of AVDO2 exceeding 9 mL/dL. The question of what level to keep the PO2 and O2 saturation is even more controversial than hyperventilation, leading to many debates between pulmonologists and neurosurgeons. The reason for the debate stems from the hemoglobin molecule and the measurements of brain tissue O2. In whole blood, the hemoglobin molecule has an O2 carrying capacity of 17 to 21 mL O2/100 mL blood, when O2 is bound to its four hemoglobin sites. However, that figure does not reflect the additional, albeit small, amounts of O2 dissolved in the blood. That amount, the PaO2, also reflects free oxygen molecules dissolved in plasma and not just those bound to hemoglobin. Likewise, O2 saturation (SaO2) alone does not reveal how much total O2 is in the blood. The O2 saturation and the PO2 can be most readily adjusted by increasing the fraction of inspired oxygen (FiO2). O2 and PO2 are also affected by perfusion such as with cerebral blood flow. In general, without concomitant disease, the hemoglobin molecule is 100% saturated at a PO2 of 80 mm Hg, 75% saturated at a PO2 of 40 mm Hg, and 50% saturated at a PO2 of 27 mm Hg. In addition to the fact that blood plasma carries extra O2, red blood cells reach only 85% of the brain cells, the remainder being dependent upon the circulating plasma. O2 does not always bind to the hemoglobin molecule in the same manner. There is a decreased affinity of hemoglobin for O2 with increasing temperature, increasing PCO2, 2,3-diphosphoglycerate (DPG), and decreasing pH. This makes it harder for the hemoglobin to bind to O2 (requiring a higher partial pressure to achieve the same O2 saturation), but it makes it easier for the hemoglobin to release bound O2. Therefore, the debate hinges on the following: arterial hemoglobin saturation of 100% is the upper limit of usefulness; increasing the FiO2 to past the maximal saturation of hemoglobin only increases the dissolved O2 in plasma, which represents 2 to 3% of overall O2 transport. Use of FiO2 levels >60% may be harmful when used for longer than 24 hours in adults. That is only half the story, however; the other half rests on studies that actually measure brain tissue oxygenation and predict outcome. In these studies, increasing PO2 to higher levels than necessary to saturate hemoglobin, as performed in the O2 treated cohort, improved the partial pressure O2 supply in brain tissue (PBtiO2). During the early period after severe head injury, increased lactate levels in brain tissue were reduced by increasing FiO2.23 Other studies have demonstrated an improved mortality rate using hyperbaric O2. Measurements demonstrated hyperbaric O2 reduces mortality by 50% and improves aerobic metabolism.24̵26 The treatment PO2 varied from keeping PO2 = 150 mm Hg27 to a simple approach of ventilation with 100% O2 for at least the first 6 to 18 hours after any severe head injury when metabolic demand is greatest.23 There are two favored technologies for measuring brain tissue oxygenation: the Licox sensor (Integra Neuro Sciences, Plainsboro, NJ), and the Codman Neurotrend (Codman and Shurtleff, Inc., Raynham, MA) cerebral tissue monitoring systems. The Licox probe (~0.5 mm in diameter) can be inserted through a standard twist drill hole into a nonlesion, where there should be a steady PBtiO2, or into a lesion area with a low but dynamic PBtiO2. The Neurotrend system measures four areas of brain metabolism and tracks physiological information about the progression of secondary injury at the cellular level, which may indicate the onset of ischemic injury, as observed in 80% of trauma-related deaths. These measurements have shown that by increasing FiO2 beyond 80%, there continues to be an increase in PBtiO2.28–32 Specifically, increasing FiO2 from 30 to 100% increased PBtiO2 to a steady state of 40 mm Hg.33 Not only does PBtiO2 improve, but also the outcome improves.34–37 When investigating hyperventilation using one of these cerebral metabolism sensors, a PCO2 = 27 to 32 mm Hg decreases PBtiO2 in severe head injury patients.38–40 Increasing the PaO2 to levels higher than needed to fully saturate hemoglobin apparently can increase PO2, especially when it is low. This effect can continue over a period of several hours. Improved outcome is characterized by Poor outcome is characterized by There are many patients on the neurology and neurosurgery service who come to the neurosurgical intensive care unit (NICU) without a severe head injury or cerebral vascular accident. These patients may develop abnormal breathing patterns from masses, inflammation, or infections. Table 22–3 lists some typical abnormal breathing patterns.
Ventilator Management
Requirements for Intubation
Reasons for Intubation
Methods Used in Intubation
Rapid Sequence Intubation
Emergent Cricothyroidectomy
Hyperventilation after Intubation
Measuring Cerebral Blood Flow
Arteriovenous Oxygen Content Difference
Jugular Venous Oxygen Saturation
Determining the Level of Oxygen
How Brain Tissue Oxygenation Is Measured
Pulmonary Problems
Type | Pattern | Location |
Cheyne-Stokes | Hyperventilation, short apnea, hyperventilation | Diffuse forebrain injury |
Central neurogenic | Hyperventilation | Midbrain, thalamus |
Apneustic | Prolonged pause at full inspiration | Pontine, brainstem |
Ataxic | Random deep and shallow breaths | Medulla |
Cluster | Irregular breaths and pauses | Low medulla |
Cheyne-Stokes respirations occur when there is diffuse forebrain injury. At that time, the patient hyperventilates low PCO2 and stops breathing, which normalizes PCO2. This results in hyperventilation and apnea, with the period of hyperventilation being longer than apnea, causing the patient to become alkalotic. In rarely occurring central heurogenic hyperventilation, there can be a midbrain lesion, such as in the thalamus.41 However, because of its unlikely presentation, the physician, when seeing continuous hyperventilation, should instead consider a cause of pulmonary edema or aspiration. With apneustic breathing, which is demonstrated by a prolonged pause at full inspiration, there may be a mid to caudal pontine lesion, brainstem stroke, or basilar artery occlusion. In ataxic breathing, there is very irregular breathing, with deep and shallow breaths randomly mixed. Ataxic breathing is a terminal stage usually pointing to a medullary lesion. A cluster pattern is characterized by clusters of breaths in irregular sequence with varying pauses, indicative of end-stage lower medullary failure or lesion. Each breathing pattern should be associated with a clinical condition verifying the level of the lesion. When there is no confirmatory exam, consider the many other causes of pulmonary dysfunction in the neurologic patient (Table 22–4).
Acute CHF | COPD/asthma | Neurogenic pulmonary edema |
ARDS | Diaphragm rupture | Pneumonia |
Airway obstruction | Diaphragm paralysis | Pneumothorax |
Apnea | Fat/brain emboli | Pulmonary contusion |
Aspiration | Hemothorax | Pulmonary edema |
Atelectasis | Hyper-/hypoventilation | Tracheobronchial |
Chest wall injury | Injury | V/Q mismatch |
ARDS, acute respiratory distress syndrome; CHF, congestive heart failure; COPD, chronic obstructive pulmonary disease; V/Q, ventilation/perfusion.
Patients with severe neurologic problems, such as severe head injury, develop secondary problems (e.g., with electrolytes) 59% of the time, pneumonia 41% of the time, and coagulopathy 18% of the time. These secondary problems usually occur 2 to 4 days after the injury and affect approximately 3% of the outcomes.42 Therefore, the neurocritical care specialist should be most cognizant of ways to prevent and treat such issues. Pneumonia can be attenuated early by ensuring airway protection, utilizing chest physiotherapy, and understanding nosocomial infections. Other nonpreventable associations with pneumonia include neutralization of gastric pH.
Pneumonia can initially be mistaken for pulmonary embolism, acute respiratory distress syndrome (ARDS), very early neurogenic pulmonary edema, or, in the nonintubated patient, even airway obstruction. Upper airway obstruction is common in the first 24 hours after stroke, especially if the patient remains in the supine position. The risks for obstruction prior to intubation are the same factors as for typical obstructive sleep apnea; body mass index and neck circumference appear to be the best predictors of its occurrence.43
The incidence of neurogenic pulmonary edema is unknown. It should be differentiated from pneumonia, aspiration, cardiac failure, and pulmonary contusion. Neurogenic pulmonary edema may occur in relation to epilepsy, meningitis, severe head injury, subarachnoid hemorrhage, or neoplasms. Early in its course, there may be dyspnea, chest pain, minor hemoptysis, tachypnea, tachycardia, fever, hypoxia, elevated SBP/pulmonary pressure, rales (not gallops), or murmurs. The chest x-ray may show bilateral central fluffy infiltrates. The etiology may be due to hydrostatic forces and permeability changes or to the release of catecholamines. If the pulmonary edema is due to increased ICP, then lower ICP and provide supportive care.
Ventilator Management
Newer techniques of mechanical ventilation allow the neurocritical care specialist access to an increased number of mechanisms not previously available. In a very large study by Esteban et al,44 who inquired into 412 medical/surgical units from the Americas and Europe, the patients requiring ventilation were on assist control (AC) in 47% of cases, synchronized intermittent mandatory ventilation (SIMV) in 46% of cases, or a combination of the two. The median tidal volume (Vt) was 9 mL/kg in AC, with a median pressure support of 18 cm H2O. Positive end-expiratory pressure (PEEP) was not used in 31% of the patients.
Ventilator types are volume-cycled ventilation, pressure-cycled ventilation, and time-cycled pressure-limited ventilation. In each type, the Vt would be (1) constant with inspiratory pressure limits dependent upon applied pressure and the compliance of the lung and chest wall with incomplete exhalation; (2) limiting, when there is incomplete exhalation and the respiratory rate increases to compensate (used mostly in infants and small children); or (3) dependent, maintaining airway pressure at a preset maximum for a preset time with an added inspiratory pause, used where there is a problem with the lung and chest wall compliance (Table 22–5).
Ventilator modes are controlled mechanical ventilation (CMV), AC, SIMV pressure support, and high-frequency jet ventilation (HFJV). CMV is independent of patient breathing; it delivers a minute ventilation determined by a preset Vt and rate. It is frequently used in the pharmacologically paralyzed patient. If the patient is not paralyzed, he or she may not be able to synchronize with the ventilator and may therefore become quite agitated. AC mode delivers only full breaths through preset Vt when the patient initiates a breath by creating negative pressure. Consequently, this assists spontaneous ventilations, and anxious patients would hyperventilate. Therefore, the triggering sensitivity must be adjusted to prevent respiratory alkalosis. SIMV modes have preset rate and Vt; however, the patient can breathe spontaneously unassisted between ventilator breaths. In pressure support (PS), the ventilator provides a constant pressure, assisting the patient’s own inspiration. By itself, PS does not provide a minimum minute ventilation, allowing the patient to work at breathing. HFJV delivers a minute ventilation at 50 to 300 breaths per minute at reduced mean airway pressure and therefore reduced ICP. It is used when there is high peak inspiratory pressure >70 to 80 cm H2O. HFJV can cause injury (e.g., dryness, mucosal injury) due to the quick movement of air at high pressures.
In addition to the modes of ventilation, PS and PEEP can be added. PEEP maintains the opening of small airways at end expiration to improve PaO2 so that a lower concentration of O2 can be used. It also increases pleural, intrathoracic, and central venous pressure (CVP) while decreasing cardiac output, SBP, free water clearance, urine, and urine sodium; therefore, amounts of PEEP should be increased or decreased slowly. The increased end-expiratory pressure can cause barotrauma. Because PEEP increases pulmonary vascular resistance and CVP, its usage in patients with severe neurologic problems has been questioned. Studies have determined that the adverse effects of PEEP are dependent upon intracranial compliance. Patients who require artificial ventilation can be safely ventilated using PEEP or an inverse inspiration:expiration ratio, if the blood pressure is monitored and a possible drop of the arterial blood pressure is treated.45 As far as the amount of PEEP, in humans 10 cm H2O of PEEP had no effect on ICP,46 whereas 15 cm H2O of PEEP in normal dogs produced mild effects that became worse if the ICP was elevated.47
Ventilator mode | Patient control | Parameters |
CMV | Machine driven | Preset Vt and rate |
AC | Patient driven, machine backup full breath | Preset Vt |
SIMV | Patient driven | Preset Vt and rate |
PS | Patient driven | Preset pressure |
HFJV | Machine driven | Preset minute ventilation |
AC, assist control; CMV, controlled mechanical ventilation; HFJV, high-frequency jet ventilation; PS, pressure support; SIMV, synchronized intermittent mandatory ventilation.
< div class='tao-gold-member'>
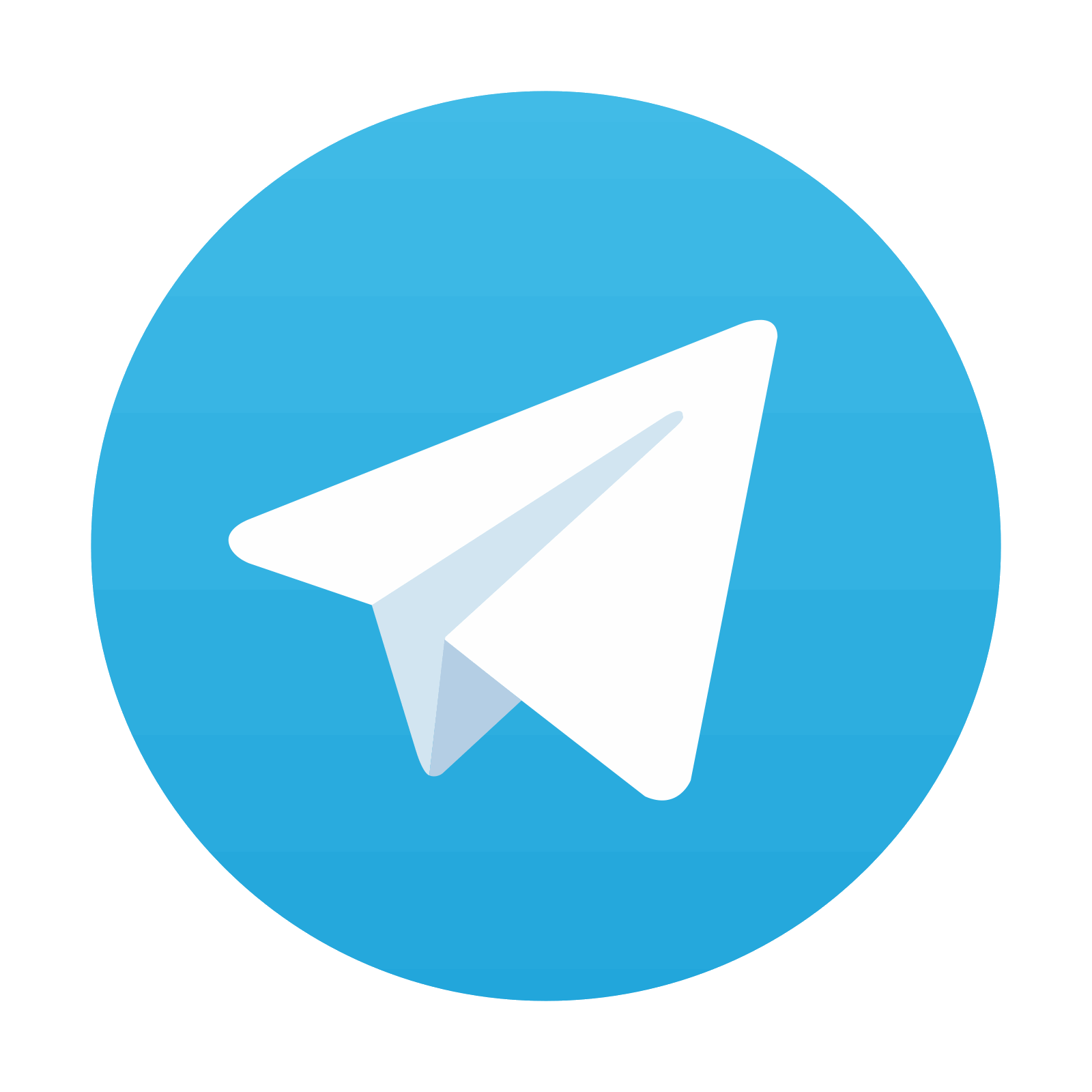