(1)
Division of Pulmonary and Critical Care Medicine, Eastern Virginia Medical School, Norfolk, VA, USA
Keywords
BloodBlood transfusionTransfusion related acute lung injury (TRALI)HemoglobinPlateletsFresh frozen plasmaTransfusion related immunomodulation (TRIM)Febrile non-hemolytic transfusion reactions (FNHTR)AnemiaDisseminated intravascular coagulation (DIC)Coagulation disordersHeparin-induced thrombocytopenia (HIT)Platelet transfusionIn transfusion medicine, several blood products can be prepared and used as replacement therapy; however, four of these products are more commonly used in general practice: RBCs, fresh frozen plasma (FFP), platelets and cryoprecipitate. RBC transfusions are mainly administered to improve tissue oxygenation in cases of anaemia or acute blood loss due to trauma or surgery. FFP, platelets and cryoprecipitate are used for the prevention and treatment of bleeding.
Red Blood Cell Transfusions
Red blood cell transfusions have been the standard of care for treating anemia for more than 100 years. Approximately 15 million units of red blood cells (RBCs) are transfused annually in the United States, and about 85 million units are transfused annually worldwide [1–3]. Historically, patients have been transfused when the hemoglobin level fell below 10 g/dL [4]. The 10/30 transfusion trigger has been ascribed to a paper by Adams and Lundy published in 1941 [5]. In this paper the authors made the following recommendation “when concentration of hemoglobin is less than 8–10 g/100 cm 3 of whole blood, it is wise to give a blood transfusion before operation” [5]. The 10/30 transfusion trigger was widely accepted without supporting evidence from clinical trials. Over the last two decades the transfusion trigger has drifted down as RCTs have failed to demonstrate a benefit from the 10/30 trigger.
Anemia is common in critically ill patients. More than 90 % of patients have a “subnormal” hemoglobin concentration by the third day of ICU admission. The etiology of anemia of critical illness is multi-factorial and complex. Decreased production of erythropoietin (EPO), impaired bone marrow response to erythropoietin, reduced red cell survival as well as blood loss from repeated phlebotomies and surgical procedures have been implicated in the anemia of critical illness. Despite the fact that blood transfusions have not been shown to improve the outcome of ICU patients (discussed in detail below) and that the current guidelines only recommend blood transfusion when the hemoglobin falls below 7.0 g/dL, almost half of all patients admitted to an ICU receive a blood transfusion [6, 7].
Why Transfuse?
![$$ {\mathrm{DO}}_2=\mathrm{C}\mathrm{O}\times \left[\left(\mathrm{H}\mathrm{b}\times \mathrm{S}\mathrm{a}\mathrm{t}\times 1.34\right)+0.031\times {\mathrm{PaO}}_2\right] $$](/wp-content/uploads/2016/10/A69851_3_En_38_Chapter_Equa.gif)
Table 38.1
Effect on oxygen delivery (DO2) by increasing cardiac output, PaO2 or hemoglobin by 20 %
Hemodynamic variable | 20 % increase | % increase in DO2 |
---|---|---|
Cardiac output | 5–6 L/min | 18 % |
PaO2 | 60–72 mmHg | 6 % |
Hemoglobin | 10–12 g/dL | 18 % |
Risks Associated with Blood Transfusion (See Fig. 38.1)
For much of the last century RBC transfusion has been viewed as having obvious clinical benefits and blood transfusion was considered as a lifesaving strategy [5]. However over the last 20 years RBC transfusion practice has come under increased scrutiny. Initially this was driven by concerns over transfusion related infections, HIV in particular. While the risk of transfusion transmitted infections has received considerable attention, the risks of this complication with modern blood banking techniques is now exceedingly remote [13]. On the other hand, it is now becoming clear that there are other important, less recognized risks of RBC transfusion related to RBC storage effects and to immunomodulating effects of RBC transfusions which occur in almost all recipients [14, 15]. The risks of blood transfusion include both infectious and non-infections complications; these are listed below [16]. Several strategies such as leukoreduction and shorter storage times (still under investigation) have been employed to reduce transfusion complications. However, the most obvious approach to reduce transfusion-related complications is to reduce the number of transfusions administered.
Risks Associated with Blood Transfusion
Infectious
Human immunodeficiency virus (HIV)
Hepatitis B, C, D
Cytomegalovirus
Parvovirus B19
Epstein–Barr virus
Human T cell leukemia/lymphoma virus
Human herpes virus 6, 7 and 8
Toxoplasmosis
Malaria
West Nile virus
TT virus
Prion disease?
Noninfectious
Immune activation
◦ Non-hemolytic febrile reactions
◦ Anaphylactoid allergic reactions
◦ Acute hemolytic reaction
◦ Delayed hemolytic reactions
◦ Transfusion related acute lung injury (TRALI)
◦ Delayed TRALI syndrome
◦ Transfusion associated graft versus host disease
Immune tolerance
◦ Nosocomial/postoperative infections
◦ Multi-organ failure
◦ Transplant tolerance
◦ Cancer recurrence?
◦ Auto-immune disease
Activation of clotting
◦ Increased risk of acute coronory syndrome
◦ Increased risk of thromboembolic disease
◦ Increased risk of arterial thrombosis
Proinflammatory
Increased oxidative injury
Complications associated with massive blood transfusion (>10 units in 24 h)
Citrate toxicity
Hypocalcaemia and hypomagnesaemia
Hyperkalemia
Hypothermia
Coagulopathy
Transfusion-Associated Immunomodulation
Transfusion related immunomodulation (TRIM) occurs to some degree in almost all recipients of blood and blood products and are the cause of most of the complications associated with blood transfusion [14]. Clinical evidence for the existence of TRIM was first reported in 1973. Opelz and colleagues provided evidence that allogenic blood recipients had improved renal allograft survival [18]. This observation was subsequently confirmed in prospective clinical trials [19]. TRIM may result in either immune activation or immune suppression, this being dependent on the interaction between host and donor factors. Clinical syndromes associated with immune activation in the recipient include a variety of transfusion reactions including febrile non-hemolytic transfusion reactions (FNHTR), transfusion associated graft-versus-host disease (TA-GvHD), transfusion related acute lung injury (TRALI), alloimmunization and possible development of various autoimmune diseases. Syndromes associated with tolerance induction and immunosuppression include increased predisposition to nosocomial and postoperative infections, cancer recurrence, microchimerism and enhanced survival of various allografts in recipients.
While TRIM appears to be a ubiquitous phenomenon the mechanisms leading to immunomodulation remains poorly understood. A number of mechanisms have been proposed relating to the transfusion of donor antigen presenting cells (APC), donor stem cells (leading to microchimerism), donor white blood cells, donor immunoglobulins, donor cytokines from activated WBC’s and iron and free hemoglobin from hemolyzed red blood cells. It is likely that these mechanism act in concert to alter the immune status of the host. Allogenic blood transfusions introduce a multitude of foreign antigens including HLA-class II bearing donor dendritic antigen presenting cells (APC) in recipients. Blood transfusions induce TRIM in two opposite ways causing either i) allo-immunization or ii) tolerance induction. Immunization is reflected by the induction of HLA alloantibodies and T cell activation, while the induction of tolerance is suggested by enhanced renal, hepatic, cardiac, pancreatic and skin allograft survival in transfused versus non-transfused recipients. Presence or absence of “autologous” HLA-DR Ag on the leucocytes of the transfusion donor plays a decisive role whether immunization or immune suppression will ensue following allogenic blood transfusion [20]. Transfusions sharing at least one HLA-DR antigen with the recipient will induce tolerance while fully HLA-DR mismatched transfusions lead to immunization. Accumulation of various soluble bioactive substances occurs during storage and includes histamine, lipids, cytokines, fragments of cellular membranes, soluble HLA class I antigens, many of which are WBC derived and play an important role in TRIM. TRIM associated immunosuppression has been associated with a decrease in the helper:suppressor T-lymphocyte ratio, a decrease in natural killer cell function, defective antigen presentation and suppression of lymphocyte blastogenesis. Since WBC’s are assumed to play a pivotal role in TRIM, it has been suggested that leukodepleted blood may have less immunomodulating properties and hence reduce the complications associated with the transfusion of non-leukodepleted blood. Hebert and colleagues reported a retrospective before-and-after cohort study conducted from August 1998 to August 2000 in 23 hospitals in Canada, enrolling 14,786 patients who received RBC transfusion following cardiac surgery or repair of hip fracture [21]. A total of 6,982 patients were enrolled during the control period and 7,804 patients were enrolled following prestorage leukoreduction. In this study the adjusted odds of death following leukoreduction were reduced (OR 0.87; CI 0.75–0.99) but serious nosocomial infections did not decrease (OR 0.97; CI 0.87–1.09). Furthermore, the frequency of febrile non-hemolytic transfusion reactions (FNHTR) decreased significantly (OR 0.86; CI 0.79–0.94) as did antibiotic use (OR 0.90; CI 0.82–0.99). While the risk of nosocomial infections were not reduced in the study by Hebert et al., a meta-analysis investigating the use of leukodepleted RBC transfusions in surgical patients demonstrated a significant reduction of postoperative infections (OR 0.522; CI 0.33–0.82, p = 0.005) [22].
“Age” of Transfused Red Blood Cells
Transfused RBC’s are stored refrigerated in a preservative solution. Saline-adenine-glucose-mannitol (SAG-M) is the most commonly used preservative solution and enables refrigerated storage of RBCs for up to 42 days following collection. This “shelf-life” is based on criteria set by the Food and Drug Administration (FDA), which requires that 75 % of transfused RBCs must be recoverable in the peripheral blood circulation 24 h after transfusion [23]. The reported mean storage duration of blood in the US is 18 days [2]. However this varies widely, with larger tertiary/quaternary hospitals generally transfusing older blood than smaller community hospitals. The mean age of blood transfused in ICU patients varies from about 16 to 24 days with “younger” blood being transfused in European as compared to US ICUs [6, 7]. Differences in blood banking protocols may explain this finding.
During refrigerated storage of RBC units, the RBCs undergo numerous physicochemical changes, collectively referred to as the RBC storage lesion, which affects the quality, function and in vivo survival of the transfused RBCs [24, 25]. Many of these changes are the consequence of oxidative stress, leading to the generation of reactive oxygen species, altered proteins and lipids, loss of cell membrane and cell constituents forming microparticles and changes to the RBC cytoskeleton resulting in alterations in the shape and deformability of the RBC. With storage the RBC loses its biconcave shape, become more spherical and spiculated. The physical changes that occur to stored RBCs appear to be similar to those that occur to diseased RBCs (such as in malaria, sickle cell disease, thalassemia). Loss of RBC membrane results in the formation of microparticles which float in the supernatant. As a result of ongoing glycolytic metabolism, lactic acid and protons accumulate in the storage solution. Furthermore, stored white cells become activated resulting in the release of cytokine and other inflammatory mediators.
RBC Storage Lesion [25]
RBC changes
Acidosis, decreased pH
Slowed metabolism, decreased ATP
Decreased 2,3 DPG, decreased O2 off-loading
Shape change, cytoskeletal damage, band 3 denaturation
Loss of cation pumping
Oxidative damage, increased lipid peroxidation
Loss of cell membrane (shedding of microparticles)
Cell shrinkage, decreased deformability
Loss of cell membrane phospholipid, cell asymmetry
RBC lysis
Accumulation in the supernatant
Acidotic, decreased pH, increased lactate
Increased K+
Increased free hemoglobin
Oxidized protein and lipids
Increased RBC microparticles
Cell debris
Bioactive mediators
Storage of RBC’s under standard blood banking conditions results in the accumulation of cell-free and microparticle-encapsulated hemoglobin. The concentration of cell-free hemoglobin increases linearly with increasing storage time [26]. Cell-free hemoglobin is cytotoxic. The cytotoxic effect of free heme is related to its pro-oxidant activity driven by the divalent Fe atom contained within its protoporphyrin IX ring, which promotes the production of free radicals [27]. Free heme has been demonstrated to play a critical role in the pathogenesis of sepsis [28]. The pro-inflammatory cytokines released following exposure to a pathogen act synergistically with free heme to promote oxidative injury [28]. In addition, free hemoglobin binds with nitric oxide (NO) leading to endothelial dysfunction and contributing to the intravascular thrombosis, vasoconstriction, and leukocyte adhesion which occurs in the septic patient [26]. The pathogenetic importance of free heme in patients sepsis is supported by an observational study reported by Janz and colleagues who demonstrated a significant increase in the mortality of septic patients with increasing concentration of cell-free hemoglobin [29]. Heme oxygenase-1 (HO-1) is the rate-limiting enzyme in the breakdown of heme into equimolar amounts of biliverdin, iron and carbon monoxide. HO-1 has a protective effect in severe sepsis and it is likely that genetic polymorphisms of this enzyme play a role in determining the severity of the septic response [27, 28].
Oxidation of cell-free hemoglobin releases free iron. The most recent theory to explain the increased risk of infectious complications following blood transfusion and the interaction with the duration of storage relates to an increased concentration of circulating non-transferrin-bound iron, which promotes proliferation of pathogenic bacteria [30, 31]. In mouse models, transfusion of RBCs stored for longer durations was followed by brisk extravascular clearance of cells damaged during storage by macrophages in the spleen and liver [31]. The iron liberated by phagocytic digestion of these RBCs rapidly entered the systemic circulation in amounts that exceeded the transport capacity of plasma transferrin, increasing the concentration of circulating non-transferrin-bound iron. A study conducted in healthy volunteers reported the presence of higher extravascular hemolysis after older RBC transfusion (storage of 40–42 days) compared with fresh blood (storage of 3–7 days) [32]. In this study transferrin saturation and non-transferrin-bound iron increased significantly after transfusion of the old blood. The increased concentrations of non-transferrin-bound iron correlated with enhanced proliferation in vitro of a pathogenic strain of Escherichia coli.
A large range of adverse effects related to RBC storage have been reported in patients when RBC’s stored for 2–4 weeks are transfused. These include increased mortality, nosocomial infections, multiple organ failure, renal failure, deep vein thrombosis, and an increase in ICU and hospital length of stay and duration of mechanical ventilation [33]. However, the clinical importance of the storage lesions is controversial as all of the studies demonstrating an association between storage time and adverse outcomes are observational studies many of which are small studies [33]. Furthermore, a number of studies have been unable to find an association between adverse clinical outcomes and the duration of storage [25, 33]. Those studies that have shown an increased risk of complication with “old” blood suggest that the increased risk of complications occurs with blood stored for longer than 14 days [10, 33, 34]. To complicate this issue further, Phelan et al. demonstrated that the deleterious effects of aging on banked blood are ameliorated by pre-storage leukoreduction [35, 36].
Currently a number of RCT’s are being conducted which should help resolve this controversial issue. The Canadian ABLE study (Age of Blood Evaluation trial) is planned to enroll a total of 2,510 ICU patients in Canada, France, and the United Kingdom who will be randomized to standard transfusion practice or receive fresh blood (7 days or less) [37]. TRANSFUSE (clintrial.gov NCT01638416) is a large (5,000 patients) pivotal, multicenter, randomized, controlled trial in critically ill patients to determine whether, compared with standard care, transfusion of the freshest available RBC decreases patient mortality. The Red Cell Storage Duration Study (RECESS) is a similar study in patients undergoing cardiac surgery (clintrial.govNCT00991341), while the Age of Blood in Children in Pediatric Intensive Care Units (ABC PICU) study (clintrial.gov NCT01977547) is investigating the effect of fresh versus aged blood in pediatric ICU patients.
The most important complications associated with the transfusion of packed red cells are reviewed below (briefly).
Increased Risk of Postoperative and Nosocomial Infections
Starting in the mid-1980s, a dose–response relationship has been reported between the quantity of RBC’s transfused and infections in various settings. Multiple observational studies have demonstrated that blood transfusion is associated with an increased risk of postoperative and nosocomial infections, increased length of hospital stay and increased mortality. While sicker patients receive more blood transfusions, multivariate analysis has consistently demonstrated that blood transfusions are independent predictors of infectious complications, morbidity and mortality. We performed a meta-analysis of 45 cohort studies that assessed the effect of RBC transfusion on patient outcomes [15]. These studies included postoperative, cardiac and ICU patients. Twenty-two studies examined the association between RBC transfusion and nosocomial infection; in all these studies blood transfusion was an independent risk factor for infection. The pooled odds ratio for developing an infectious complication was 1.8 (95 % CI; 1.5–2.2). Hill and colleagues performed a meta-analysis of studies investigating the risk of postoperative infections in patients receiving a blood transfusion [38]. In this study, the odds ratio ranged from 1.43 to 15.15, with a common odds ratio of 3.45. A more recent meta-analysis by Rohde et al. demonstrated that in hospitalized patients a liberal transfusion strategy (as compared to a restrictive strategy) was associated with an increased risk of health care associated infections [39]. It is important to note that leukodepletion has reduced but not eliminated the risk of infections complications following a blood transfusion. A metaanalysis by Blumberg et al. reported that leukoreduced transfusions reduced the odds of a postoperative infection by approximately 50 % (OR 0.522; CI 0.332–0.821; p = 0.005) [22]. In a large prospective study which included 5,158 adult patients undergoing cardiac surgery at 10 centers in the United States and Canada, Horvath and colleagues demonstrated a dose-related association between the quantity of RBCs transfused (all leukocyte-reduced) and risk of infection, with the risk increasing by an average of 29 % with each RBC unit transfused [40]. Similarly in ICU patients, Juffermans and colleagues have demonstrated that transfusion of leukodepleted blood increases the risk of secondary infections [41, 42]. In this study patients who received older blood had an increased risk of infections [42]. These studies provide overwhelming evidence that RBC transfusions increase the risk of postoperative and nosocomial infections; this risk is reduced but not eliminated by the transfusion of leukodepleted blood.
Febrile Non-hemolytic Transfusion Reactions (FNHTR)
A FNHTR is defined, arbitrarily, as a temperature increase of 1 °C (1.8 °F) or more associated with a RBC transfusion in the absence of any other likely causes for fever [43]. This reaction may occur either during or up to 1–2 h following the transfusion. Additional features include increases in respiratory rate, changes in blood pressure, anxiety and, more unusually, nausea or vomiting. FNHTRs are the most commonly encountered transfusion reaction occurring in approximately 0.5–2.0 % of units transfused and are more likely to occur following transfusion of platelets than RBCs. FNHTRs and believed to be due to leucocyte activation, either donor or receipt with the release of cytokines (similar mechanism to acute TRALI). Leucocyte reduction decreases the incidence of FNHTRs with prestorage leucocyte reduction being more effective than post-storage leucocyte reduction. Fever following a transfusion is attributed to FNHTR when other potential life-threatening transfusion reactions, such as acute homolytic transfusion reaction, bacterial contamination or TRALI are excluded. In patients with suspected FNHTR the transfusion should be immediately discontinued. The remainder of the transfused unit and a post-transfusion blood sample from the patient should be sent to the laboratory for further investigation. FNHTRs are typically benign, and usually resolve completely within 1–2 h after the transfusion is discontinued. Antipyretics may be administered to shorten the duration of the fever and provide analgesia. About 10–15 % of patients who experience an FNHTR may have a similar reaction in the future transfusion [43]. Administration of antipyretics (acetaminophen) 30–60 min before starting transfusion is often recommended for a patient who has had two or more FNHTRs, although there is little data to support this approach.
Transfusion Related Acute Lung Injury (TRALI)
TRALI is a serious transfusion adverse reaction characterized by the acute onset of non-cardiogenic pulmonary edema following transfusion of blood products. Although all blood components have been implicated, TRALI is more commonly associated with plasma-containing products (e.g., FFP and aphaeresis platelets), which account for the majority (50–63 %) of TRALI fatalities. TRALI is characterized by the abrupt onset of respiratory failure within 6 h following the transfusion of a blood product. While TRALLI is usually mild and resolves within hours it is the commonest cause of death following the transfusion of a blood product. It is likely that TRALI is underdiagnosed with the features of respiratory compromise frequently being ascribed to circulatory overload (TACO). TRALI is usually caused by donor anti-leukocyte antibodies. A single unit of packed cells or blood component product (FFP and platelets) is usually implicated in initiating this syndrome. It has, however, recently been recognized that the transfusion of blood products in critically ill or injured patients increases the risk for the development of acute lung injury (ALI) 6–72 h after the transfusion. This “Delayed TRALI Syndrome” is common, occurring in up to 25 % of critically ill patients receiving a blood transfusion, and is associated with a mortality of up to 40 % [44]. While the delayed TRALI syndrome can develop after the transfusion of a single unit, the risk increases as the number of transfused blood products increase. The management of both the classic and delayed TRALI syndromes is supportive.
Transfusion Associated Circulatory Overload (TACO)
TACO is defined as cardiogenic pulmonary edema that occurs during or immediately following a blood transfusion. It is believed to occur due to rapid intravascular volume expansion in the setting of diminished cardiac reserve (systolic or diastolic heart failure). It may be quite difficult to distinguish TACO from TRALI; however patients with TACO are likely to have abnormal LV function on echocardiography.
Transfusion-Associated Thrombosis
The transfusion of stored RBC increases the risk of thrombotic complications. A number of mechanisms have been postulated to explain this finding [45]. The microparticles shed from the RBC membrane contain high concentrations of phosphatidyl-l-serine, a potent promoter of factor VIIa activation and thrombin generation [46]. Plasminogen activator inhibitor 1 (PAI-1) accumulates in stored blood. The transfusion of blood products has been associated with the release of CD40 ligand from platelets. CD40L binding to vascular endothelial cells stimulates the expression of metalloproteinases, matrix-degrading enzymes implicated in plaque rupture and thrombosis. RBC transfusions in patients with cancer, traumatic injuries and subarachnoid hemorrhage have been reported to have an increased risk of venous thromboembolism and arterial thromboses [47–49]. Increasing storage time (presumably with an increased released of bioactive lipids) appears to increase the pro-thrombotic properties of transfused blood [48].
Decreased Survival and Tumor Recurrence Following Surgery
As blood transfusions may result in immune tolerance and interfere with immune surveillance it has been suggested that perioperative blood transfusions may increase the likelihood of tumor recurrence. Evidence for a possible deleterious effect of allogenic blood transfusion has been reported in the context of tumors of the colon, rectum, breast, head and neck, lung, prostrate, stomach, kidney, cervix and vulva [50]. In a prospective study by Nosotti and colleagues in patients with stage I lung cancer undergoing lobectomy, blood transfusion resulted in decreased disease-free and overall survival [51]. A meta-analysis of randomized controlled studies of patients undergoing curative resection of colorectal cancer by the Cochrane group reported a pooled odds ratio of cancer recurrence of 1.42 (95 % CI, 1.20–1.67) associated with blood transfusion [52]. More recently Ng and colleagues demonstrated that transfusion of leukodepleted blood (as compared to no transfusion) was associated with a worse disease-free and overall survival in patients with resected stage I non-small cell lung cancer [53].
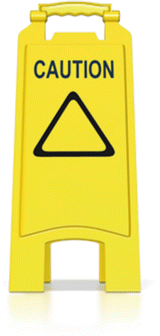
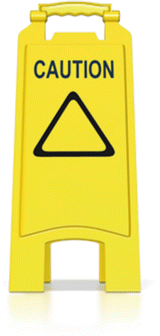
Blood transfusion should be considered as an organ transplant. The need for transfusion should be carefully evaluated, avoided when possible and considered only as a last-resort life-saving measure.
Tolerance to Anemia
In health, the amount of oxygen delivered to the whole body exceeds resting oxygen requirements almost fourfold. An isolated decrease in hemoglobin concentration to 10 g/dL with all other parameters remaining constant will result in an oxygen delivery that remains approximately twice that of the resting oxygen consumption. Humans have a remarkable ability to adapt to anemia by increasing cardiac output (in the absence of volume depletion), increasing microcirculatory density, as well as by increasing red cell synthesis of 2,3-DPG with a resultant rightward shift of the oxyhemoglobin dissociation curve (aids oxygen unloading) and by increasing oxygen extraction. Healthy volunteers can tolerate isovolemic hemodilution down to hemoglobin concentration of 4.5 g/dL without apparent harmful effects [54]. However, due to the high extraction ratio of oxygen in the coronary circulation, coronary blood flow appears to be the major factor which limits the tolerance of low hemoglobin concentrations. In experimental animal models of coronary stenosis, depressed cardiac function occurs at hemoglobin concentrations between 7 and 10 g/L [55, 56].
Extensive experience in patients who decline blood for religious reason, as well as in patients with chronic renal disease, myelodysplastic syndromes and severe autoimmune hemolytic anemias have confirmed that humans tolerate extreme anemia quite well [57, 58]. The best data comes from the Jehovah Witness literature [57]. Carson and colleagues performed a retrospective cohort study in 1,958 patients who underwent surgery and declined blood transfusions for religious reasons [59, 60]. In those patients without cardiovascular disease and with a blood loss of less than 2.0 g/dL there was no significant increase in perioperative mortality (for a baseline hemoglobin of 6–6.9 g/dL and a decline in hemoglobin of less than 2 g/dL the odds ratio (OR) for death was 1.4; 95 % CI, 0.5–4.2). However, in patients with cardiovascular disease, pre-operative anemia was associated with a significant increase in peri-operative mortality. This data confirms that humans can adapt to very low hemoglobin levels with cardiovascular disease being the major limiting factor.
Weighing the Risks and Benefits of Blood Transfusion
The benefit/harm of blood transfusion are related to a number of factors including:
Leukodepleted versus non leukodepleted blood
The length of storage (age of the blood)
The number of units transfused
The immune status of the recipient
The baseline hemoglobin
Presence of coronary artery disease
Presence of ongoing bleeding
Presence of comorbidities
So, When Should Patients’ Be Transfused?
No randomized clinical trial comparing transfusion with no transfusion has been performed. However, a number of randomized clinical trials have been conducted that compared a more or less restrictive transfusion strategy using different transfusion triggers. Three randomized controlled trials which enrolled 2,364 patients evaluated a restrictive hemoglobin transfusion trigger of <7 g/dL as compared with a more liberal trigger. These studies include:
The Canadian Critical Care Trials Group Study (TRICC) which randomized 838 adult ICU patients to a transfusion trigger of <7 or 10 g/dL [61].
The TRIPICU study randomized 889 pediatric ICU patients to a transfusion trigger of <7 or <9.5 g/dL [62].
Villanueva et al. randomized 921 patients with severe acute upper gastrointestinal bleeding to a transfusion trigger of <7 or <9 g/dL [63].
The pooled results from these three studies showed that a restrictive hemoglobin transfusion trigger of <7 g/dL resulted in reduced in-hospital mortality (RR 0.74; CI 0.60–0.92), total mortality (RR, 0.80; CI, 0.65–0.98), rebleeding (RR, 0.64; CI, 0.45–0.90), acute coronary syndrome (RR, 0.44; CI, 0.22–0.89), pulmonary edema (RR, 0.48; CI, 0.33–0.72), and bacterial infections (RR, 0.86; CI, 0.73–1.00), compared with a more liberal strategy [17].
Carson et al. randomized 2,016 patients >50 years of age who had undergone hip surgery and had risk factors for cardiovascular disease to a liberal transfusion strategy (trigger of 10 g/dL) or a restrictive transfusion strategy (trigger of 8 g/dL) [64]. There is no difference in morbidly or mortality between the two groups. In a pilot study, Walsh et al. randomized “older” (>55 years of age) patients requiring mechanical ventilation to a restrictive (transfusion trigger < 7 g/dL) or liberal transfusion strategy (transfusion trigger < 9 g/dL) [65]. Mortality at 180 days postrandomization trended toward higher rates in the liberal group (55 %) than in the restrictive group (37 %); relative risk was 0.68 (95 % CI, 0.44–1.05; p = 0.073).
Anemia is a well-recognized to be a poor prognostic factor in patients with congestive cardiac failure as well as acute coronary syndromes (ACS) [66, 67]. However, this does not mean that blood transfusion improves outcome. Kansagara et al. performed a meta-analysis which included 6 RCT’s and 26 observational studies investing the role of blood transfusion in patients with heart disease [68]. These authors concluded that “evidence from suggests that liberal transfusion protocols do not improve short-term mortality rates compared with less aggressive protocols (RR 0.94; CI 0.61–1.42).” Chatterjee et al. performed a meta-analysis investigating the association between blood transfusion and outcomes in patients with acute myocardial infarction [69]. In this meta-analysis blood transfusion increased all-cause mortality (OR 2.91; CI, 2.46–3.44, p < 0.001). Multivariate meta-regression revealed that blood transfusion was associated with a higher risk for mortality independent of baseline hemoglobin level, nadir hemoglobin level, and change in hemoglobin level during the hospital stay. Blood transfusion was also significantly associated with a higher risk for subsequent myocardial infarction (OR 2.04; CI 1.06–3.93, p = 0.03). Rao and colleagues examined the potential impact of red blood cell transfusion in 24,111 patients with ACS [70]. Blood transfusion was an independent predictor of myocardial infarction and 30-day all-cause mortality (adjusted hazard ratio 3.94). Furthermore, the 30-day mortality was significantly increased when transfusions were given to patients with hematocrits of 25 % or above (compared to those with a hematocrit below 25 %). Similarly Aronson and colleagues demonstrated an increase in mortality and the composite end-point of death/recurrent MI/heart failure in patients with acute myocardial infarction who had a hemoglobin > 8 g/dL and received a blood transfusion [71].
The 2012 guidelines from the American Association of Blood Banks (AABB) recommend adhering to a restrictive transfusion strategy (7–8 g/dL) in hospitalized, stable patients (Grade: strong recommendation; high-quality evidence) [72]. Furthermore these guidelines state that “transfusion decisions should be influenced by symptoms as well as hemoglobin concentration” (Grade: weak recommendation; low-quality evidence). The 2013 guidelines from the American College of Physicians recommend recommends using a restrictive blood cell transfusion strategy (trigger hemoglobin threshold of 7–8 g/dL compared with higher hemoglobin levels) in hospitalized patients with coronary heart disease (Grade: weak recommendation; low-quality evidence) [73].
Based on current evidence in patients who are not actively bleeding, RBC transfusions should be restricted to those patients with a Hb < 7 g/dL. However, it is likely that many patients can tolerate a hemoglobin concentration lower 7 g/dL, suggesting that the patients’ physiologic reserve, presence of coronary artery disease and other comorbidities should be taken into account when making blood transfusion decisions. Furthermore, in most instances patients should be given one unit of RBC at a time. One unit of packed RBCs should increase levels of hemoglobin by 1 g/dL and the hematocrit by 3 %.
Coagulation Disorders in the ICU
Coagulation disorders are commonly encountered in the ICU. Many conditions including sepsis, malignancy, trauma, vasculitic disorders and obstetrical accidents may give rise a coagulopathy. In addition patients may have medical conditions which predispose them to developing a coagulopathy; e.g. patients with liver disease, renal failure, lupus, leukemia, etc. Sepsis, however, is the single most common factor leading to a coagulopathy and DIC. DIC has been reported in about 10–20 % of patients with gram-negative bacteremia and 70 % of patients with septic shock. In patients with sepsis, DIC appears to be an important independent predictor of ARDS, multiple organ dysfunction syndrome and death.
A coagulopathy is best defined as the presence of an abnormal coagulation test(s). The Scientific Subcommittee on Disseminated Intravascular Coagulation (DIC) of the International Society on Thrombosis and Haemostasis (ISTH) has suggested that DIC be considered “an acquired syndrome characterized by the intravascular activation of coagulation with loss of localization arising from different causes. It can originate from and cause damage to the microvasculature, which if sufficiently severe, produce organ dysfunction’ [74]. DIC is characterized by the generation of fibrin related products (soluble fibrin monomer, fibrin degradation products, D-dimer, etc.) and is indicative of an acquired (inflammatory) or non-inflammatory disorder of the microvasculature.
DIC results from the systemic activation of both the clotting and fibrinolytic systems leading to the consumption of many coagulation factors and platelets. The initial activation of coagulation in sepsis is primarily dependant on activation of the extrinsic (tissue-factor dependant) pathway. DIC is generally considered to be a systemic hemorrhagic syndrome. However, this is only because hemorrhage is obvious and often impressive. Less commonly appreciated is the formidable microvascular thrombosis that occurs. Fibrin deposition in the microcirculation is a frequent, if not invariable finding in patients with DIC. This microvascular thrombosis is closely related to the development of multiple organ dysfunction syndrome and is therefore closely linked to the prognosis of patients with DIC. This microvascular damage is especially pronounced in the lungs and kidneys. In septic patients with DIC, the thrombotic (anti-fibrinolytic) pathways tend to dominate. The DIC that characterizes the early stage of traumatic shock is characteristically hemorrhagic (fibrinolytic) which then becomes thrombotic by day 2–4; this pattern has important implications with regards to resuscitation with blood and blood products [75]. A dilutional coagulopathy and DIC frequently occur in cases of massive hemorrhage regardless of their cause. Coagulation tests can be used to assess the severity of the dilutional coagulopathy and DIC as well as their evolution under the influence of therapeutic interventions. However, coagulation tests fail to predict the risk of bleeding (see below).
The “common” causes of coagulopathy/DIC in the ICU include:
Sepsis with DIC
Trauma
Massive hemorrhage
Liver disease
Malignancy
Obstetrical calamities
Excessive anticoagulation
Diagnosis of DIC
Laboratory features
◦ peripheral blood smear will show fragmented red blood cells
◦ prolonged PT and PTT
◦ thrombocytopenia
◦ decreased levels of fibrinogen
◦ decreased levels of Protein C
◦ decreased levels of anti-thrombin III
◦ increased levels of fibrin split products and D-dimer
Clinical features associated with bleeding
◦ bleeding from venipuncture sites, mucous membranes, hematuria, GI bleeds, intra-cerebral bleeds
◦ petechia, purpura, and subcutaneous hematomas
Clinical features of end organ damage due to thrombosis
◦ acute lung injury
◦ proteinuria and renal insufficiency
◦ hepatocellular dysfunction
◦ mental state changes and neurological deficits
The treatment of DIC remains controversial. This is primarily because there are very few studies which have objectively examined various therapeutic strategies in patients with DIC. The essential therapeutic modality is to treat the triggering disease process. Patients with DIC should NOT be given FFP in order to correct abnormal coagulation tests. The administration of FFP should however be considered in patients with DIC who are actively bleeding.
Fresh Frozen Plasma
Fresh frozen plasma (FFP) contains normal levels of the stable clotting factors, albumin and immunoglobulins [76]. It contains at least 70 % of the original coagulant factor VIII and at least similar quantities of the other labile clotting factors and natural inhibitors of coagulation.
Derived from whole blood usually, sometimes apheresis
Frozen within 8 h of collection
Volume: 200–250 mL
Storage: frozen up to 1 year
Content: “normal” levels of all coagulation factors
Expiration: 24 h after thawing
The recommended therapeutic dose of FFP is 10–15 mL/kg of body weight
ABO compatibility required, crossmatching not required
Of all the individual blood components FFP is one of the most hazardous, the major risks include:
◦ TRALI
◦ Allergic reactions
◦ Transmission of infections
◦ Fluid overload
◦ Increased risk of infections
◦ Hemolysis due to anti-A and anti-B
FFP is an important cause of TRALI. Khan et al. demonstrated that TRALI was more likely to develop in patients who received FFP transfusions (OR, 2.48; 95 % CI, 1.29–4.74) and platelet transfusions (OR, 3.89; 95 % CI, 1.36–11.52) than in those who received only RBC transfusions (OR, 1.39; 95 % CI, 0.79–2.43) [77]. Watson demonstrated that FFP was an independent predictor of MODS and ALI in trauma patients [78]. In addition, similar to blood transfusion, transfusion of FFP has been associated with an increased risk of infections in ICU patients [79].
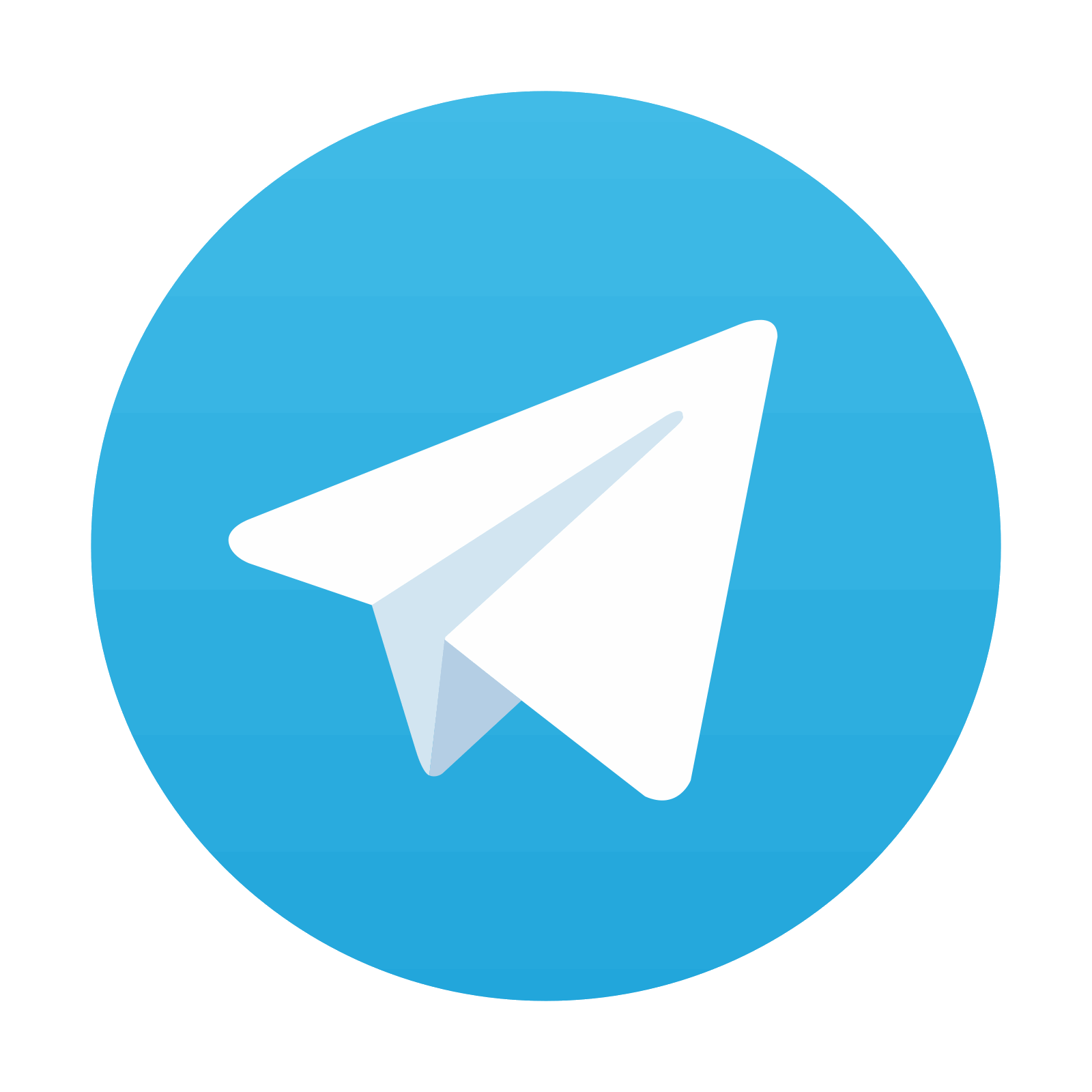
Indications for FFP [76]
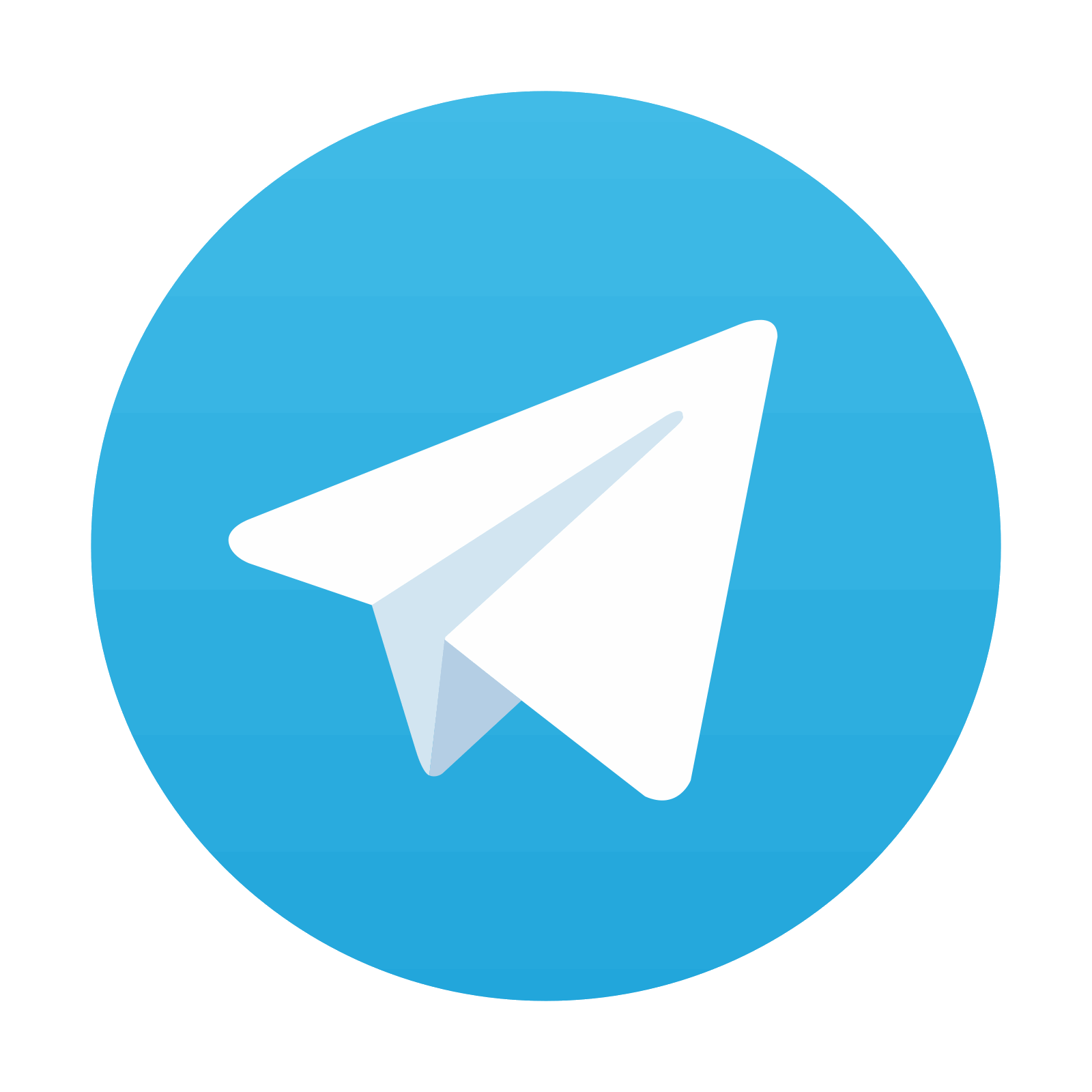
Stay updated, free articles. Join our Telegram channel
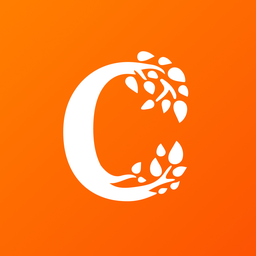
Full access? Get Clinical Tree
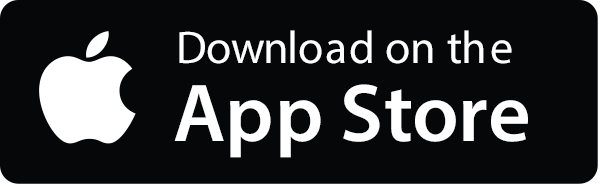
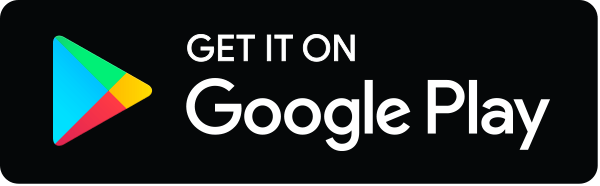
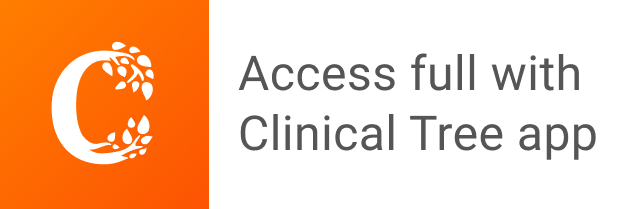