Chapter 33
The Endocrine System and Anesthesia
General Principles of Endocrine Physiology
Body homeostasis is controlled by two major regulating systems: the nervous system and the endocrine or hormonal system.1,2 Both of these systems communicate, integrate, and organize the body’s response to a changing internal or external environment.1,3
Hormones
Endocrine function is mediated by hormones. Hormones are the signaling molecules or chemical messengers that transport information from one set of cells (endocrine cells) to another (target cells). Hormones are released from endocrine glands into body fluids in minute quantities but exert powerful control over most metabolic functions.1,4
Transmission of a hormonal signal through the bloodstream to a distant target cell (e.g., pituitary gland to the adrenal gland) is called an endocrine function. If a hormone signal acts on a neighboring cell of a different type (e.g., pancreas α cells to pancreas β cells), the interaction is termed a paracrine function. If the secreted hormone acts on the producer cell itself or on neighboring identical cells, the interaction is called an autocrine function.1,2
Types of Hormones
Peptide or Protein Hormones
Most hormones have a peptide or protein structure. This group of hormones includes insulin, growth hormone, vasopressin (antidiuretic hormone), angiotensin, prolactin, erythropoietin, calcitonin, somatostatin, adrenocorticotropic hormone, oxytocin, glucagon, and parathyroid hormone. Peptide hormones are synthesized in endocrine cells as prehormones and prohormones. They are processed by the cell and stored in secretory granules within the endocrine gland.1,2 The proper stimulus to secretion causes exocytosis of the peptide or protein hormone into the extracellular fluid.
Amine- or Amino Acid–Derivative Hormones
Several hormones are amino acid or amine compound derivatives. Serotonin, important for its central nervous system effects, is synthesized from the naturally occurring amino acid tryptophan.2 Thyroid hormones and catecholamine hormones (dopamine, epinephrine, and norepinephrine) are derived from the amino acid tyrosine. Thyroid hormones and catecholamine hormones are stored in the thyroid gland and adrenal medulla, respectively, and are released by the appropriate stimulation.1
Steroid Hormones
All steroid hormones are lipid soluble derived from cholesterol or have a chemical structure similar to that of cholesterol.4 Common steroid hormones include hormones of the adrenal cortex (e.g., cortisol, aldosterone) and reproductive hormones (e.g., estrogen, progesterone, testosterone). Active metabolites of vitamin D are also steroid hormones.1 In contrast to most other hormones, steroid hormones are not stored in discrete secretory granules but are compartmentalized within the endocrine cell and released into the extracellular fluid by simple diffusion through the cell membrane.1,2
Circulating steroid and thyroid hormones are bound to transport proteins, whereas circulating catecholamine hormones and most protein hormones are not bound to carriers. Plasma protein binding protects hormones from metabolism and renal clearance.2 The circulating half-life of steroid and thyroid hormones is therefore typically longer than that of peptide and catecholamine hormones. For example, the thyroid hormone thyroxine, which is 99.95% protein bound, has a plasma half-life of 6 days, whereas insulin, which has essentially no plasma protein binding, has a half-life of about 7 minutes.1
The major sites of hormone degradation and elimination are the liver and the kidneys. Some hormone degradation also occurs at target-cell sites.1,2,4
Hormone Receptors
Binding to a specific target-cell receptor is the primary event that initiates a hormone response.2 The hormone receptor displays high specificity and affinity for the proper hormone ligand, and the location of the receptor directs the hormone to the specific target organ or target-cell site.3 Some hormones, such as insulin and growth hormone, act on widespread target sites; others, such as thyroid-stimulating hormone, act on one target tissue.1 After binding, the hormone-receptor complex induces a cascade of intracellular events that produce specific physiologic responses in the target cell.2
Hormone Receptor Activation
Hormone receptors are located either on the surface of cells or inside cells.4 Receptors for protein, peptide, and catecholamine hormones are located in or on the surface of the target-cell membrane. Hormone binding to a cell membrane receptor triggers a response by activating enzyme systems in or near the plasma membrane bilayer. The activated enzymes generate intracellular signals, called second messengers, which carry the hormone’s message within the intracellular space.
Several different second-messenger systems operate in response to cell membrane receptor-hormone binding. Probably the most widely described second-messenger system is the cyclic adenosine monophosphate (cAMP) system. This hormone transduction mechanism is initiated when receptor occupation activates the plasma membrane enzyme adenyl cyclase. The membrane-bound adenyl cyclase then catalyzes the intracellular conversion of adenosine triphosphate (ATP) to cAMP; cAMP in turn becomes the hormone’s intracellular messenger, activating intracellular enzymes, modifying cell-membrane permeability or transport, and altering cellular gene expression.1 The enzyme phosphodiesterase catalyzes the hydrolysis of cAMP and terminates its intracellular actions. Hormones that use cAMP as their second messenger include thyroid-stimulating hormone, vasopressin, parathyroid hormone, glucagon, some catecholamines, corticotropin, follicle-stimulating hormone, and luteinizing hormone.
In contrast to peptide and catecholamine hormones, thyroid and steroid hormones produce the desired target-cell response chiefly by interacting with specific intracellular hormone receptors.1 Thyroid and steroid hormones are small lipophilic molecules that enter target cells by simple diffusion or by special transport mechanisms. Once within the cell, these hormones occupy specific intracellular receptors.2 In combination with their receptors, the hormones interact with DNA in the cell nucleus to enhance or suppress gene transcription or translation.1,2
Thyroid and steroid hormones enable the cell to alter gene expression, protein formation, and cell activity in response to environmental and developmental stimuli.2 Every hormone has a specific onset and duration of action.4 Hormones that act by binding to cell membrane receptors (peptide, protein, and catecholamine hormones) usually generate a hormonal effect in seconds to minutes. Hormones that bind to intracellular receptors and activate the transcription processes of specific genes (thyroid and steroid hormones) may require several hours or even days to generate a hormonal response.1,4
Hormone Receptor Regulation
Receptors are dynamic molecules that are constantly being destroyed and replaced. The receptor for insulin, for example, has a normal half-life of only about 7 hours.2 Hormone receptor destruction may be part of a normal endocrine response or part of an acquired or genetic disease state.
In many instances, the hormone receptor number is inversely related to the concentration of the circulating hormone. A sustained elevation of the plasma level of a given hormone may cause the target site to decrease the number of receptors per cell. This down-regulation of receptor number serves to decrease the responsiveness of a target cell to hormone excess.1 The insulin resistance observed in obesity and type 2 diabetes mellitus may be partly explained by down-regulation of the insulin receptors in response to chronically high levels of circulating insulin.2
Conversely, a low circulating hormone concentration may cause the target gland to increase the number of hormone receptors per cell.2 This up-regulation of hormone receptor number amplifies the cell’s sensitivity to hormone stimulation.1,2
The number of receptors in a target cell usually changes from day to day.4 Regulation of receptor turnover, and thus hormone receptor number, is a mechanism by which hormone activity can be precisely modulated.1
Regulation of Hormone Secretion
Neural control can evoke or suppress hormone secretion. Pain, emotion, smell, touch, injury, stress, sight, and taste can alter hormone release through neural mechanisms.5 Glucagon, cortisol, antidiuretic hormone, and catecholamines, for example, are all stimulated by the stress response to anesthesia, surgery, and trauma.
The secretion of many hormones is governed by genetically encoded or acquired biorhythms. These intrinsic hormonal oscillations may be circadian (e.g., the daily variability in glucocorticoid secretion), weekly (e.g., the menstrual cycle), or seasonal (e.g., thyroxine production).3,5 The biorhythms also may vary at different stages of development and life (e.g., growth hormone secretion).6
Feedback control is another sophisticated mechanism through which a hormonal response is controlled. Many endocrine disorders arise from the breakdown of feedback loops.2 Negative feedback acts to limit or terminate the production and secretion of a given hormone once the appropriate response has occurred. Negative feedback of a target-cell product to the hormone producer (the endocrine gland) limits or prevents hormone excess. When concentrations of the product are low, feedback inhibition to the endocrine gland is lessened and hormone secretion enhanced.
Virtually all hormones are controlled by some type of negative feedback mechanism.3,6 For example, parathyroid hormone is controlled by calcium, insulin and glucagon are controlled by glucose, and vasopressin is controlled by serum osmolarity.5 The negative feedback mechanism is a very important factor in the regulation of hormones of the hypothalamus and pituitary gland. Hypothalamic hormones stimulate the release of pituitary hormones from the pituitary gland. The pituitary hormones in turn may stimulate an output of product from peripheral target cells. Product from peripheral target tissues may then initiate feedback to the pituitary gland or the hypothalamus to inhibit pituitary or hypothalamic hormone synthesis and discharge.2,5
Positive feedback is a less common hormone-regulating mechanism in which a given hormone response initiates signals amplifying hormone release. The surge in luteinizing hormone (LH) that precedes ovulation is stimulated by LH; this is an example of positive feedback.2
Pituitary Gland
Relationship Between Pituitary Gland and Hypothalamus
The pituitary gland, or hypophysis, is known as the “master endocrine gland.”7 It secretes hormones that have far-reaching effects on various homeostatic, developmental, metabolic, and reproductive functions of the body. The pituitary is a small endocrine gland (only 500 mg in weight and about the size of a pea) centrally located at the base of the brain. It is enclosed within a bony cavity of the sphenoid bone called the sella turcica.5,6 The pituitary gland is connected to the overlying hypothalamus by the hypophyseal stalk (pituitary stalk). The hypothalamus is located below the thalamus, behind the optic chiasm and between the optic tracts. The pituitary, hypothalamus, and some of the surrounding structures are shown in Figure 33-1.
The brain, via the hypothalamus, is an important regulator of pituitary gland secretion. The hypothalamus collects and integrates information (e.g., pain, emotions, energy needs, water balance, olfactory sensations, electrolyte concentrations) from almost all parts of the body and uses this information to control the secretion of vital pituitary hormones.5,6 Pituitary hormone secretion also is regulated by feedback control from peripheral target-organ hormones or other target-organ products.6 The pituitary gland and hypothalamus have virtually no blood-brain barrier, allowing feedback products to exert potent effects.8,9
Functionally and histologically, the pituitary gland is divided into two distinct portions: the anterior lobe (adenohypophysis) and the posterior lobe (neurohypophysis).5,6 The anterior pituitary lobe is embryologically derived from an upward invagination of pharyngeal epithelial cells. The posterior pituitary lobe develops from a downward outpouching of ectoderm from the brain. Blood supply to the pituitary is from the superior and inferior hypophyseal arteries.5,7
Anterior Pituitary Lobe
The anterior pituitary lobe, which constitutes about 80% of the pituitary gland by weight, secretes six primary hormones.6 Target sites for the anterior pituitary hormones are shown in Figure 33-2.
1. Growth hormone (GH, somatotropin) promotes skeletal development and body growth and regulates protein and carbohydrate metabolism.
2. Adrenocorticotropic hormone (ACTH) regulates the growth of the adrenal cortex and the release of cortisol and androgenic hormones from the adrenal gland. ACTH possesses mild melanocyte-stimulating properties, resulting in skin pigmentation at high levels.
3. Thyroid-stimulating hormone (thyrotropin or TSH) controls the growth and metabolism of the thyroid gland and the secretion of thyroid hormones, which regulate the rates of most chemical reactions in the body.5,6
4. Follicle-stimulating hormone (FSH) stimulates ovarian follicle development in females and spermatogenesis in males.
5. Luteinizing hormone (LH) induces ovulation and corpus luteum development in females and stimulates the testes to produce testosterone in males.
6. Prolactin promotes mammary gland development and milk production (lactogenesis) by the breasts. Prolactin also exerts an effect on reproductive function by inhibiting the synthesis and secretion of LH and FSH. Prolactin synthesis is markedly increased during pregnancy.5
Several other less important or less well-defined hormones also are secreted from the anterior pituitary lobe.6
Anterior pituitary hormones are synthesized and secreted by five distinct endocrine cell types within the gland: somatotrophs synthesize GH; gonadotrophs synthesize the two gonadotropic hormones, LH and FSH; thyrotrophs synthesize TSH; corticotrophs synthesize ACTH; and lactotrophs (mammotrophs) synthesize prolactin. About 40% to 50% of the anterior pituitary cells are somatotrophs and about 20% are corticotrophs.5
Control of Anterior Pituitary Hormone Secretion
Synthesis of anterior pituitary hormones is controlled by signals from the hypothalamus. Neurosecretory cells in various hypothalamic nuclei respond to input from the body by synthesizing specific neurohormones that have corresponding anterior pituitary target-cell types.6
Hypothalamic neurohormones are released into a capillary bed of the hypothalamus in an area called the median eminence. The hypothalamic hormones travel from the capillary plexus of the median eminence, down the pituitary stalk, in a specialized vascular system called the hypothalamic-hypophyseal portal vessels. At the anterior pituitary lobe, the hypothalamic hormones are released in high concentrations into capillary sinuses located among the glandular cells.6 The hypothalamic hormones then locate and bind to their specific target-cell type.
Specific hypothalamic hormones have either an inhibitory or a stimulatory effect on their corresponding anterior pituitary target cells. Synthesis and release of most anterior pituitary hormones depend on a positive stimulatory signal from a given hypothalamic hormone. Some anterior pituitary cells are subject to both inhibitory and stimulatory control by more than one hypothalamic neurohormone.5
Synthesis of prolactin from anterior pituitary lactotroph cells is unique in that it is tonically restrained by an inhibitory hormonal signal (dopamine) from the hypothalamus. In essence, dopamine serves as a “physiologic brake” for lactotroph growth and prolactin synthesis. The inhibitory effect of dopamine agonists, such as bromocriptine, is exploited therapeutically for suppressing pathologic production of prolactin from pituitary tumors.5,7 Table 33-1 outlines the major hypothalamic releasing or inhibiting hormones and their corresponding anterior pituitary target sites.
Anterior Pituitary Disorders
Disorders involving the anterior pituitary system may be due to a defect at the peripheral endocrine gland (primary disorder), the pituitary gland (secondary disorder), or the hypothalamus (tertiary disorder).5 Pituitary tumors account for 15% of all intracranial tumors.10 Most pituitary tumors, both functional and nonfunctional, are benign adenomas. Pituitary carcinoma is exceedingly rare.7,10
Hyposecretion
Anterior pituitary hyposecretion may occur when large nonfunctional pituitary tumors (e.g., chromophobe adenoma, craniopharyngioma, Rathke’s pouch cysts) compress and destroy normal anterior pituitary cells. Postpartum shock (Sheehan syndrome), irradiation, trauma, infiltrative disorders (e.g., sarcoidosis, amyloidosis) and hypophysectomy are other causes of pituitary hormone deficiency states. Generalized pituitary hypofunction (panhypopituitarism) is more common than reduced output of a single anterior pituitary hormone.7,11
Important effects of panhypopituitarism include a decrease in thyroid function due to reduction in levels of TSH, depression of glucocorticoid production by the adrenal cortex due to the lowering of ACTH levels, and suppression of sexual development and reproductive function due to deficient gonadotropic hormone secretion.6 In addition, large pituitary tumors (macroadenomas greater than 1 cm) may extend into or compress the surrounding brain tissue, producing diplopia, visual loss, facial numbness, facial pain, or seizures.12
Surgical intervention may be implemented to control bleeding or for decompression or removal of the pituitary tumor. Surgical patients with hypopituitary disorders may require thyroid hormone replacement and glucocorticoid coverage in the perioperative period.13 Because of the possibility of diabetes insipidus after removal of the tumor, vasopressin should also be available.
Hypersecretion
Most pituitary tumors are benign hypersecreting pituitary adenomas.12 The three most common hypersecreting pituitary tumors are those that produce prolactin, ACTH, or GH. Tumors that secrete gonadotropin and thyrotropin hormones are rare. Pituitary tumors also may be inherited as part of multiple endocrine neoplasia (MEN) type 1.10,14
Prolactin-secreting tumors commonly produce symptoms of galactorrhea, amenorrhea, and infertility in women and decreased libido and impotence in men.7 The dopamine agonist bromocriptine is used to control prolactin levels, decrease tumor size, and restore normal gonadal function. Patients who have a suboptimal response to medical therapy benefit from microsurgical removal of the pituitary tumor.7
Growth Hormone
Growth hormone (somatotropin) is synthesized and secreted by somatotroph cells of the anterior pituitary lobe and is under dual control by the hypothalamus.6 Growth hormone–releasing hormone stimulates GH release, and growth hormone–inhibiting hormone (somatostatin) is a powerful inhibitor of GH release. Pulsatile fluctuations of the hypothalamic releasing and inhibiting hormones regulate somatotroph activity throughout the day.5
The GH secretion rate is generally increased in childhood, followed by a further increase in adolescence, a plateau in adulthood, and declining values in old age. The normal physical decline associated with aging may in part be due to the age-related decline in GH production.5,6 In addition, GH secretion is stimulated by stress (including anesthesia and surgery), hypoglycemia, exercise, and deep sleep.5
Unlike the other anterior pituitary hormones, GH does not exert its principal effects through a specific target gland but functions through all or almost all tissues of the body; it promotes the growth and development of most tissues capable of growing.6 A major target of GH is the liver, where it stimulates the production of insulin-like growth factor type 1 (IGF-1), a hormone that mediates many of GH’s effects.6,10 Skeletal muscle, the heart, skin, and visceral organs undergo hypertrophy and hyperplasia in response to GH and IGF-1.10,15
The most obvious effect of GH is on the skeletal frame. It produces linear bone growth by stimulating the epiphyseal cartilage or growth plate at the ends of long bones.5,6 Throughout childhood, under the influence of GH, bones elongate at the epiphyseal plate, and the skeletal frame enlarges. After puberty, the growth plates unite with the shaft of the bone, bone lengthening stops, and GH has no further capacity to increase bone length.6
GH and IGF-1 support growth by increasing amino acid transport into cells and enhancing protein synthesis in the cell. GH also decreases the catabolism of existing proteins by stimulating lipolysis and mobilizing free fatty acids for energy use, a protein-sparing effect.6 In addition to its growth-promoting activities, GH is said to be a “diabetogenic hormone.” It increases blood glucose levels by decreasing the sensitivity of cells to insulin and inhibiting glucose uptake into cells.6
As is true of other anterior pituitary hormones, GH secretion is subject to negative feedback control. The primary negative feedback controller of the pituitary somatotrope is IGF-1. GH itself, as well as IGF-1, exert negative feedback control on the hypothalamus. GH release is also inhibited by hyperglycemia and increased plasma free fatty acids.5
Hyposecretion
Deficient GH production in childhood can result in insufficient bone maturation and short stature, a condition known as dwarfism. Mild obesity, decreased lean body mass, and hypoglycemia are common in GH-deficient dwarfs. Puberty usually is delayed. Symptoms of GH deficiency may be the result of hypothalamic dysfunction, pituitary disease, failure to generate normal insulin growth factor hormones, or GH-receptor defects.6,7
The biosynthesis of human GH by recombinant DNA techniques has enhanced the outlook for patients with GH deficiency. Treatment of these patients with GH leads to a positive nitrogen balance, accretion of lean body mass, and an improvement in metabolic homeostasis.7
Hypersecretion
Hypersecretion of GH, usually caused by a growth hormone–secreting pituitary adenoma (99% of cases), can produce a highly distinctive syndrome in adults called acromegaly. Acromegaly is produced by sustained hypersecretion of GH after adolescence. The condition occurs with equal frequency in both sexes.12 If hypersecretion of GH occurs before puberty—that is, before closure of the growth plates—the individual grows very tall (8 to 9 feet), a rare condition known as gigantism.
Because growth plates close with adolescence, the excessive production of GH associated with acromegaly does not induce bone lengthening but rather enhances the growth of periosteal bone by the stimulatory effects of GH on bone osteoblasts (bone forming cells). Periosteal growth causes new bone to be deposited on the surface of existing bone.6 The unrestrained bone growth in patients with acromegaly produces bones that are massive in size and thickness. Bones of the hands and feet (acral) become particularly large. Overgrowth of vertebrae may cause kyphoscoliosis and osteoarthritis.
Soft-tissue changes are also prominent with GH hypersecretion. The patient develops coarsened facial features (acromegalic facies) that include a large, bulbous nose, supraorbital ridge overgrowth, dental malocclusion, and a prominent prognathic mandible.10,16 The changes in appearance are insidious, and many patients do not seek treatment until the diagnosis is obvious and the disease course advanced.10,16,17
Overgrowth of internal organs is less apparent clinically but no less serious. The liver, heart, spleen, and kidneys become enlarged. Pulmonary function tests are consistent with increased lung volumes and extrathoracic obstruction, but gas exchange is usually not grossly abnormal.17 Exercise tolerance may be limited due to increased body mass and skeletal muscle weakness.7
Cardiomyopathy and hypertension in patients with acromegaly can lead to symptomatic cardiac disease (e.g., diastolic dysfunction, congestive heart failure, arrhythmias).13,17,18 Bi-ventricular concentric hypertrophy manifests early in the disease course.7,10
The insulin-antagonistic effect of GH produces glucose intolerance in most patients and frank diabetes in up to 25% of patients with acromegaly.7
Clinical manifestations resulting from the local effects of the expanding tumor may include headaches (55%), papilledema, and visual field defects (19%), which are caused by compression of the optic nerves and chiasm. Significant increases in intracranial pressure are uncommon. Compression or destruction of normal pituitary tissue by the tumor may eventually lead to panhypopituitarism.7 Common features of acromegaly are summarized in Box 33-1.
Life expectancy in patients with acromegaly is decreased on average by about 10 years.10 Most patients die of cardiovascular causes. Hormonal control has a definite impact on survival. Lowering serum GH to less than 2.5 mcg/L results in reduction of the mortality rate to levels comparable with the general population.19,20
Treatment for acromegaly is aimed at restoring normal GH levels. The preferred initial therapy for active acromegaly is microsurgical removal of the pituitary tumor, with preservation of the gland.12 Surgery achieves biochemical cure (normalization of IGF-1 and a glucose-suppressed GH of less than 2 mcg/L) in about 70% to 90% of microadenomas (less than 1 cm). Cure rates for macroadenomas by surgery are much lower (about 50%).7,10,21
Surgical approach to the pituitary tumor most often is via the endonasal transsphenoidal route, and this route is generally well tolerated by most patients.8,10,13 A transcranial approach may be used for very large tumors with suprasellar extension.13
For transsphenoidal pituitary surgery, the head of the bed is typically elevated 15 degrees to improve venous drainage.13,22 The anesthetist should consider monitoring for venous air embolism, especially if cavernous sinus invasion by the tumor is suspected and the patient is positioned in a steep head-up tilt.12 Infrequently, the approach and exposure of the tumor are associated with significant blood loss. The use of submucosal injection of epinephrine-containing solutions or topical vasoconstrictors to assist in hemostasis may result in large blood pressure increases.23 An anesthetic technique that incorporates muscle relaxation and allows for smooth extubation and rapid neurologic assessment is desirable.12 Nitrous oxide should be omitted from the anesthetic plan if air is injected surgically to aid with tumor visualization. Preparing the patient preoperatively for awakening with nasal packing is a consideration for some cases. Surgical complications are not common, but may include epistaxis, transient diabetes insipidus, cranial nerve damage, symptomatic hyponatremia, and cerebral spinal fluid leaks.22
Surgical ablation is usually successful in rapidly reducing tumor size, inhibiting GH secretion, and alleviating some symptoms.7,24
Administration of octreotide or lanreotide (long-acting somatostatin analogs), pegvisomant (a GH-receptor antagonist), and gland irradiation are adjunctive treatments for tumor regression or treatment options for patients who are not surgical candidates.10,18,21
Anesthetic Implications of Acromegaly
Preanesthetic assessment of patients with acromegaly should include a careful examination of the airway. Facial deformities and the large nose may hamper adequate fitting of an anesthesia mask. Endotracheal intubation may be a challenge because of the patient’s large and thick tongue (macroglossia), prognathism, enlarged thyroid gland, obstructive teeth, hypertrophy and distortion of the epiglottis, and general soft-tissue overgrowth in the upper airway.8,13,17,25–27 Subglottic narrowing and vocal-cord enlargement may dictate the use of a smaller-diameter endotracheal tube. Nasotracheal intubation should be approached cautiously because of possible turbinate enlargement.11 The occurrence of Mallampati III and IV grades is higher in patients with acromegaly, and the incidence of difficult intubations may be four to five times higher than patients without acromegaly.24,26 Preoperative dyspnea, stridor, or hoarseness should alert the anesthetist to airway involvement.11 Indirect laryngoscopy, computed tomography (CT) scan of the neck, and neck radiography may be performed for thorough assessment. If difficulties in maintaining an adequate airway are anticipated, optically guided intubation or fiberoptic-guided intubation in an awake patient is of proven value.12,13,17 Equipment for tracheostomy should be available if airway changes are advanced.12 The endotracheal tube should remain in place until the patient is fully awake and has total return of reflexes.
More than 60% of patients with acromegaly have a history of sleep apnea associated with upper airway obstruction.13,17 Sleep apnea and the predisposition to airway obstruction in these patients makes assiduous perioperative monitoring of the patient’s respiratory status an absolute precaution.12,18
The frequent occurrence of cardiac arrhythmias, coronary artery disease, and hypertension in acromegalic patients warrants a thorough preanesthetic cardiac evaluation. Hyperglycemia may complicate the perioperative period, mandating careful perioperative monitoring of blood glucose and electrolyte levels.17,18
Entrapment neuropathies, such as carpal tunnel syndrome, are common in patients with acromegaly.7 If arterial access is required, an Allen test should be performed before placement of a radial artery catheter; hypertrophy of the carpal ligament may cause inadequate ulnar artery flow. Alternatively, catheterization of other arterial sites should be considered.11
Posterior Pituitary Lobe
The posterior pituitary lobe secretes two important peptide hormones: antidiuretic hormone (arginine vasopressin or ADH) and oxytocin. Oxytocin and ADH are structurally very similar, but they have quite different actions. ADH controls water excretion and reabsorption in the kidney and is a major regulator of serum osmolarity. Oxytocin stimulates contraction of myoepithelial cells of the breast for milk ejection during lactation. It also powerfully stimulates uterine smooth muscle contraction.5 Oxytocin and its derivatives are used clinically for inducing labor and decreasing postpartum bleeding.
In contrast to the anterior pituitary lobe, which communicates with the hypothalamus via a vascular system, the posterior pituitary lobe communicates with the hypothalamus through a neural pathway. Unlike anterior pituitary hormones, posterior pituitary hormones are not synthesized within the pituitary gland itself but rather within two large nuclei of the hypothalamus, the supraoptic nucleus and the paraventricular nucleus. ADH is chiefly synthesized in the supraoptic nucleus and oxytocin in the paraventricular nucleus.5,6 As shown in Figure 33-3, nerve fibers arising from these hypothalamic nuclei transport ADH and oxytocin down the pituitary stalk by axoplasmic flow to the posterior pituitary lobe. There, the hormones are stored in secretory granules at the nerve terminals. With proper excitation, nerve impulses originating in the cell bodies of the supraoptic or paraventricular nucleus are transmitted down the pituitary stalk and stimulate the release of ADH or oxytocin from the posterior pituitary lobe. The hormones then diffuse into nearby blood vessels and are transported to their distant target sites.
Three types of vasopressin receptors have been identified: V1, V2, and V3. Activation of receptor V1 mediates vasoconstriction. V2 receptors mediate water reabsorption in the renal collecting ducts. V3 receptors are found within the central nervous system and their stimulation modulates corticotrophin secretion.9
Antidiuretic Hormone
ADH is the body’s principal preserver of water balance. It acts on V2 receptors on renal collecting ducts to increase the absorption of solute-free water through water channels called aquaporins. The integrated role of thirst, vasopressin, and renal response conserve water in the body and support normal body-fluid osmolarity. Plasma osmolarity is physiologically controlled within a small range (285 to 290 mOsm/L).9 Without ADH, the collecting ducts are impermeable to water reabsorption; in this setting, water loss in the urine is excessive, and serious dehydration is provoked.6
ADH acts primarily to increase urine osmolarity, decrease serum osmolarity, and increase blood volume.6 Additionally, high levels of ADH stimulate V1 receptors and cause potent systemic vasoconstriction, especially in coronary, splanchnic, and renal vascular beds. ADH-induced vasoconstriction of vascular beds has been exploited therapeutically for the control of vasodilatory shock, hemorrhage, and sepsis.9 Current advanced cardiac life support protocols recommend vasopressin as an adrenergic alternative to epinephrine for promoting the return of spontaneous circulation after cardiac arrest. Desmopressin (1-deamino-8-d-arginine vasopressin [DDAVP]), a synthetic arginine analog of ADH, increases circulating levels of von Willebrand factor and factor VIII, and is used to reverse coagulopathy associated with platelet adhesion defects.9
Consonant with its role of maintaining normal fluid homeostasis, ADH is secreted in response to an increase in plasma osmolarity or plasma sodium ion concentration, a decrease in blood volume, or a decrease in blood pressure.6
The osmolarity of body fluids is the main variable controlling ADH secretion. Serum osmolarity changes are sensed by hypothalamic osmoreceptors, which in turn alter ADH synthesis and secretion.5 The plasma osmotic threshold for ADH release is only 2% to 4% higher than normal plasma osmolarity.5,28 When the plasma tonicity increases even subtly, healthy individuals release ADH into the blood.
The interplay between ADH and water is controlled by a delicate negative feedback loop. Water deprivation (increased plasma osmolarity) initiates signals in the hypothalamic osmoreceptors that cause ADH release from the pituitary gland to increase three- to five-fold. ADH, in turn, enhances renal tubular water reabsorption, dilutes the extracellular fluid, and restores normal osmotic composition.6 Conversely, water ingestion (decreased plasma osmolarity) suppresses the osmoreceptor signal for ADH release.
A 10% to 20% decrease in blood volume or blood pressure also provokes ADH release.28 Changes in blood volume are sensed in peripheral baroreceptors (especially the great veins and pulmonary vessels) and atrial stretch receptors. When these baroreceptors sense underfilling (volume depletion), they transmit afferent signals through vagal and glossopharyngeal nerves to the hypothalamus.5,28 The hypothalamus responds by increasing ADH synthesis and stimulating ADH release.
The perioperative period is characterized by enhanced ADH secretion.29,30 Pain, emotional stress, nausea, hemorrhage, and various drugs can be potent stimuli to ADH release. Positive-pressure ventilation enhances ADH release by reducing central blood volume.8 The mild hyponatremia sometimes observed postoperatively may be at least partly explained on the basis of ADH action. Box 33-2 lists factors that stimulate ADH release or enhance the action of ADH at the renal tubules.11
Thirst provides a second line of defense of water balance. The thirst threshold is set about 5% higher than the osmotic threshold for ADH.6,28
Deficient Antidiuretic Hormone and Anesthetic Implications
Inadequate ADH secretion from the posterior pituitary lobe or the inability of renal collecting duct receptors to respond to ADH (impaired receptor sensitivity) results in a disorder called diabetes insipidus (DI). Decreased ADH release produces neurogenic or central DI, and renal tubular resistance to vasopressin is termed nephrogenic DI.28
Common causes of neurogenic DI include severe head trauma, neurosurgical procedures (e.g., trauma to the median eminence, pituitary surgery), infiltrating pituitary lesions, and brain tumors.28 Neurogenic DI that develops after pituitary surgery is usually transient and often resolves in 5 to 7 days.8,13
Nephrogenic DI may occur in association with genetic mutations, hypercalcemia, hypokalemia, and medication-induced nephrotoxicity.28 Ethanol, demeclocycline, phenytoin, chlorpromazine, and lithium all inhibit the action of ADH or its release.8
The hallmark of DI is polyuria. The inability to produce a concentrated urine results in dehydration and hypernatremia. The syndrome is characterized by a low urine osmolarity (less than 300 mOsm/L), urine specific gravity less than 1.010, and urine volumes greater than 2 mL/kg/hr.28 The tremendous urinary water loss produces serum osmolarities greater than 290 mOsm/L and serum sodium concentrations greater than 145 mEq/L. Neurologic symptoms of hypernatremia and neuronal dehydration may be present and include hyperreflexia, weakness, lethargy, seizures, and coma.28
The thirst mechanism assumes a primary role in maintaining water balance in awake patients with DI. Ingestion of large volumes of water prevents serious hyperosmolarity and life-threatening dehydration.8
Significant deficiency (plasma osmolarity levels greater than 290 mOsm/L) may be treated with various ADH preparations. Desmopressin (DDAVP), a selective V2 agonist, is often a preferred agent because it has less vasopressor activity, a prolonged duration of action (8 to 12 hours), and enhanced antidiuretic properties.9,28 DDAVP may be administered nasally, intravenously, subcutaneously, and orally. Dosages for nasal application range from 5 to 40 mcg/day. Subcutaneous dosages are 0.5 to 2 mcg twice daily.
Perioperative administration of vasopressin is usually not necessary in the patient with partial DI, because the stress of surgery causes enhanced ADH release.8,30 The surgical patient with a total lack of ADH (complete DI) may be managed with desmopressin (1 mcg subcutaneously) or aqueous vasopressin (an intravenous bolus of 0.1 unit, followed by a continuous intravenous infusion of vasopressin at 0.1 to 0.2 unit/hr).8 Caution is advised when administering these drugs to patients with coronary artery disease or hypertension because of the arterial constrictive action of ADH.28
Plasma osmolarity, urine output, and serum sodium concentration should be measured hourly during surgery and in the immediate postoperative period.8
Isotonic fluids can generally be administered safely during the intraoperative period. If, however, the plasma osmolarity rises above 290 mOsm/L, hypotonic fluids should be considered and the vasopressin infusion increased above 0.2 unit/hr.8
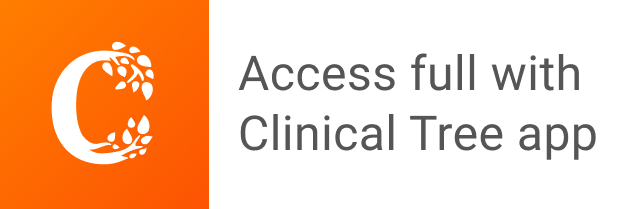