© Springer International Publishing AG 2017
Anthony R. Absalom and Keira P. Mason (eds.)Total Intravenous Anesthesia and Target Controlled Infusions10.1007/978-3-319-47609-4_2828. TCI and TIVA for Neurosurgery: Considerations and Techniques
(1)
Anesthesiology Institute, Cleveland Clinic Abu Dhabi (CCAD), Swing Wing L7, Al Maryah Island, 112412, Abu Dhabi, United Arab Emirates
Keywords
AnestheticsIntravenous/administration and dosageAnesthesia recovery periodCraniotomy/methodsNeurophysiologyPostoperative complications/epidemiologyPostoperative nausea and vomitingPostoperative nausea and vomiting/epidemiologyPropofol/administration and dosageThe main goals of neurosurgical anesthesia are to provide the patient with an adequate anesthetic that provides a stable hemodynamics, reduces cerebral metabolism, avoids intracranial hypertension, avoids interference with intraoperative neuromonitoring, and allows rapid emergence from anesthesia for neurological examination [1]. Volatile anesthetics combined with synthetic opioids have been used in the past years for neurosurgical procedures because these combinations allow for rapid recovery and prompt neurological assessment [2, 3]. However, volatile anesthetics have been shown to affect cerebral autoregulation [4] and intracranial pressure (ICP ) [2, 5], which can make the surgery more difficult and dangerous, increasing the risk of ischemic cerebral insults.
The modern use of total intravenous anesthesia (TIVA ) began in the late 1970s with the introduction of propofol which was then combined with synthetic opioids [6–8] to provide general anesthesia (GA) during cranial procedures and spine surgery. Propofol, barbiturates (thiopental), and etomidate have minimal effect on or even decrease ICP [9]. A clear advantage of this new anesthetic technique was the fast recovery and a reduction in postoperative side effects as nausea and vomiting when compared to inhalational anesthetics [10].
It is important to understand how the normal brain and nervous system are affected during general anesthesia to avoid overdosing anesthetic drugs causing an intraoperative impairment of cerebral autoregulation and postoperative adverse events such as delirium and delayed awakening.
In specific conditions such as in patients with acute brain injuries (traumatic brain injury, subarachnoid hemorrhage, intraparenchymal hemorrhage) and acute spine trauma, one of the major goals is to avoid secondary neuronal injuries caused by administration of anesthetics (and their consequences such as hypotension) if our patients should have to undergo emergent neurosurgical procedures. For these reasons, it is essential to have continuous monitoring of the depth of anesthesia and where appropriate of the spinal pathways during the intraoperative period [11, 12].
More recently, target-controlled infusion (TCI ) of intravenous anesthetics has been introduced during neurosurgical procedures. The use of these new anesthetic techniques associated with an accurate depth of anesthesia monitoring represents an open field of research in terms of recovery and outcome.
Neurophysiology of the Brain During Anesthesia
Assessment of Consciousness During General Anesthesia
Loss of consciousness is an essential element of general anesthesia (GA) [13], but anesthesiologists have no reliable ways to be certain that a patient is unconscious. In practice, responsiveness to verbal commands is the standard method to assess the level of consciousness [14]. However, the level of consciousness cannot be reliably assessed in patients who are paralyzed by neuromuscular blocking agents [15]. The standards for assessing if patients are adequately anesthetized include indirect measures of brain state—such as changes in heart rate and blood pressure, which reflect sympathetic activity, and muscle tone—along with estimated blood and brain concentrations of intravenous anesthetics, presumed pharmacodynamic effects thereof, and, for inhaled anesthetics, the exhaled anesthetic agent concentrations.
The electroencephalogram (EEG ), which measures scalp electrical potentials generated by cortical postsynaptic currents [16], has long been considered the most feasible approach for tracking brain states under GA. Despite attempts to characterize EEG morphology under GA [17, 18], reading the EEG has not become part of routine anesthesiology practice. A variety of EEG patterns are known to arise during GA maintained by both GABA-A receptor-specific and ether-derived anesthetics. These EEG patterns include increases in frontal EEG power [19, 20], a shift in EEG power toward lower frequencies (alpha from 8 to 12 Hz and delta 0–4 Hz) [21], changes in coherence [22, 23], and burst suppression and isoelectricity [24].
The relationship between these or other EEG patterns and the loss and recovery of consciousness remain poorly understood. In particular, it has been difficult to identify specific EEG signatures that are associated with the point of loss of consciousness (LOC), because most anesthesia-related EEG data come from clinical settings in which GA induction is performed rapidly, causing the crucial transition from consciousness to unconsciousness within 30–60 s [25]. Compounding this rapid LOC is the problem of measuring level of consciousness. The most common approach is to ask patients to respond to a verbal or physical stimulus and rate the quality of responses on a 0–5 numerical scale [26]. This highly subjective assessment usually is repeated on a time scale of minutes [27] and resolves poorly the time point at which consciousness is lost or regained. EEG signatures that predict return of consciousness have been difficult to establish for similar reasons. Purdon and colleagues studied the relationship between EEG activity and the LOC and recovery of consciousness (ROC) [28] recording high-density EEGs while administering increasing and decreasing doses of propofol to healthy volunteers who were executing an auditory-response task used to assess conscious behavior. They used a propofol TCI from 0 to 5 μg/ml and then decreased the target-effect site concentration to 0 μg/ml. They found that LOC was marked simultaneously by an increase in low-frequency EEG power (<1 Hz), the loss of spatially coherent occipital alpha oscillations (8–12 Hz), and the appearance of spatially coherent frontal alpha oscillations. These dynamics reversed with recovery of consciousness. The low-frequency phase modulated alpha amplitude in two distinct patterns. During profound unconsciousness, alpha amplitudes were maximal at low-frequency peaks, whereas during the transition into and out of unconsciousness, alpha amplitudes were maximal at low-frequency nadirs. This latter phase–amplitude relationship predicted recovery of consciousness. Their findings can be used to track transitions into and out of unconsciousness. Commercially available monitors for bispectral analysis are unable to detect these modulation patterns most likely because the broad range of frequencies that are pooled together (0.5–47 Hz) cancels out phase information.
In some neurosurgical patients, these neurophysiological monitoring techniques are not always possible or are only partially possible (e.g., when a full EEG montage cannot be used) when the underlying brain tissue can be altered because of an inflammatory process or a tumor. Nonetheless, it is surprising how much reliable information concerning the depth of anesthesia is still found in the EEG .
The situation appears to be more promising with regard to sensory-evoked potentials, in particular auditory-evoked potentials (AEPs ). AEPs are obtained by giving a series of very brief tones via headphones to a normal subject and then recording and averaging the resulting electrical potential over the scalp. The middle latency response (MLR ) lasts from 10 ms to about 100 ms following stimulus onset, arises from the auditory cortex, and resembles three to five cycles of a 40 Hz wave [29]. Later responses such as the P300 wave peaking at 300 ms can be greatly enhanced with selective attention [30]. Madler and colleagues studied the effect of various anesthetics on the 40 Hz oscillations in the MLR [31]. For patients anesthetized with Pentothal or propofol, the 30–40 Hz oscillations shifted to lower frequencies (10Hz or less) or even disappeared. Nonspecific gas anesthetics such as isoflurane or enflurane are associated with a concentration-dependent reduction in the oscillatory frequency of the AEPs . However, these 30–40 Hz oscillations were not abolished by receptor-specific agents, such as fentanyl, ketamine, or various benzodiazepines. The same authors conclude that as long as 30–40 Hz oscillations are present, the patient is still to some extent conscious. The above results become more significant when seen in the light of other reports [31] of stimulus-induced 40–60 Hz oscillations in the neuronal discharge of neurons in the visual cortex of the anesthetized and the awake cat.
Anesthetic Drugs and Models Used for Intravenous Anesthesia in Neurosurgery
Anesthetic Drugs
The use of intravenous agents for induction and maintenance of general anesthesia during neurosurgical procedures is becoming more popular than in the past when the use of TIVA was not supported by sufficient evidence and accurate pharmacokinetic models (and the necessary computer power) were not available to guide or even control the administration of intravenous drugs [3].
Propofol shares with volatile anesthetics the important attribute of depressing cerebral metabolic rate [32], but, in common with other intravenous agents, it causes cerebral vasoconstriction in proportion to the suppression of metabolic rate [33]. Propofol has clear advantages as it is able to maintain stable the cerebral blood volume hence preserving autoregulation and vascular reactivity [34]. In a randomized prospective study of neurosurgical patients undergoing craniotomy for cerebral tumors with propofol, the patients presented lower ICP , less cerebral swelling at the opening of the dura, and higher CPP than those anesthetized with volatile anesthetics [35]. There are still some conflicting results on the effect of propofol on CBF as in healthy subjects it has been demonstrated to significantly reduce both CBF and jugular venous oxygen saturation when compared to sevoflurane, especially when associated with hypocapnia [36] although recent data show that increases in propofol concentrations do not affect jugular venous bulb oxygen saturations within the dose range used clinically [37].
Remifentanil can be considered a valuable component of neuroanesthetic pharmacology. When it was compared with fentanyl in a randomized, multiinstitutional, double-blinded, prospective trial [38], it was found to be a reasonable alternative to fentanyl during elective supratentorial craniotomy for space-occupying lesions. The frequency of adverse events, hemodynamic profiles, and median recovery times were generally similar between groups. However, 7 of 32 patients in the fentanyl group required naloxone to recover from anesthesia compared with no patients in the remifentanil group. More recently, another randomized controlled trial [39] compared remifentanil and fentanyl when used in patients undergoing surgical procedures to remove intracranial space-occupying lesions. Remifentanil was given according to manufacturer recommendations. Fentanyl was given according to the usual practice of the anesthesiologist. The study demonstrated no differences between opioid groups for the frequency of responses to intubation, pinhead holder placement, skin incision, or closure of the surgical wound. Adverse event frequencies were similar between groups. Times to follow verbal commands and tracheal extubation were more rapid for remifentanil. The percentage of patients with a normal recovery score at 10 min after surgery was higher when remifentanil was used. The study demonstrated a lesser need for opioid use when remifentanil was associated with isoflurane leading to a faster recovery from anesthesia. The association of propofol–remifentanil has shown a reduced risk of coughing during emergence [40], and this is desirable after neurosurgical and neurointerventional procedures, where coughing can precipitously increase the ICP .
The use of sufentanil–propofol TIVA was compared to remifentanil–propofol [41], and both regimens had similar results in terms of postoperative nausea and vomiting, shivering, and respiratory depression. The sufentanil–propofol regimen had a better outcome in terms of reduced supplemental postoperative analgesic requirements and better postoperative cognitive recovery than in the remifentanil–propofol group.
Dexmedetomidine (DEX ) has been introduced to clinical anesthesia for its sympatholytic, sedative, anesthetic sparing and hemodynamic stabilizing properties without significant respiratory depression. Its role during general anesthesia for neurosurgical procedures has been validated as an adjunct to other agents to decrease the intraoperative opioid dose requirements [42]. Animal and human studies [43, 44] have shown that DEX causes a reduction in CBF and cerebral metabolism rate of oxygen (CMRO2) and suggest a careful control during its administration to avoid hazardous hypotension and a reduction in the cerebral autoregulation. Its role as an intravenous anesthetic agent has been studied as an adjunct to local anesthesia during awake anesthesia [45] or as an adjunct to TIVA techniques including remifentanil [43] during which it decreased allowed analgesic requirements and improved hemodynamic stability. A possible disadvantage could be related to its prolonged sedative effect when used as an adjunct to propofol although this has not been demonstrated in clinical studies. Anesthesiologists must remain alert to the main complications occurring after neurosurgical procedures, which can also occur after TIVA administration, and include postoperative hypertension, shivering, and postoperative nausea and vomiting (PONV ) [46]. Careful intraoperative management and choice of drugs and techniques may help reduce the incidence of these complications.
Anesthetic Techniques
Induction of anesthesia with anesthetic agents and opioids administered by manual injection can cause significant depression of the mean arterial pressure, apneic episodes, and chest wall rigidity. The introduction of TCI according to pharmacokinetic models facilitates smoother induction, with smaller doses, and slower infusion rates, than with manually administered and controlled infusions, and this may attenuate the reduction of mean blood pressure on induction.
The two main pharmacokinetic models used for TCI of propofol in neurosurgery are the Marsh model and the Schnider model [47]. The Schnider model requires the user to input age, height, gender, and total body weight during start-up of the TCI pump. The pump calculates the lean body mass for that patient and calculates doses and infusion rates accordingly. It is be more suitable in the elderly who have a lower lean body mass and provides smaller doses of propofol for induction and maintenance of a constant plasma concentration than in younger patients. The induction dose is small compared with that for manual administration (often <50 %). Actual plasma levels are difficult to predict as neurosurgical patients are often taking enzyme-inducing anticonvulsants that can influence drug metabolism and thus the achieved plasma concentration. Final levels chosen on the TCI pump therefore may vary widely.
Induction of anesthesia with propofol can be achieved by titrating the dosage by 0.5–1 μg ml−1 up to reach loss of consciousness then maintaining the dosage between 3 and 4 μg ml−1 or starting at 4.5 μg ml−1 and increasing up to 7 μg ml−1 if required. Monitoring of depth of anesthesia is essential when using TCI , to facilitate titration of the target concentration according to a combination of depth of anesthesia and the hemodynamic response, to avoid excessively deep levels of anesthesia such as that associated with prolonged periods of burst suppression. A new model has been recently suggested in obese patients to improve the performance of the Marsh and Schnider models [48]
The most commonly used pharmacokinetic model for remifentanil in neuroanesthesia is the Minto model, which is a three-compartment pharmacokinetic model specific for remifentanil. This model was produced from a study of pharmacokinetics of remifentanil in a heterogeneous population of healthy adults [49]. As with propofol infusions, if blood targeting is used and rapid onset of anesthesia is required, then the blood concentration should be set to a level higher than the desired and likely therapeutic effect site concentration. After induction, target concentration has to be adjusted accordingly to blunt responses to placement of the Mayfield head holder and subsequent surgical stimuli. Typical target concentrations for remifentanil during induction and intubation are initially set at around 4–7 ng ml−1. This could be soon reduced after intubation depending on the patients’ clinical response. In the absence of muscle relaxants, target concentrations below 3 ng/ml are not advisable, as coughing and movement are more likely, even with reasonable propofol doses. Target remifentanil concentrations up to 10–15 ng/ml may be required during neurosurgical procedures involving cranial nerve stimulation or extensive craniotomies, but anesthesiologists should bear in mind that these high concentrations may be associated with acute tolerance or hyperalgesia. In any case, a careful neuromonitoring assessment with pEEG monitors should be performed to avoid excessive sedation and prolonged periods of burst suppression rate.
Propofol and remifentanil show synergistic interactions. Partly this is caused by pharmacokinetic interactions (remifentanil causes a 41 % decrease in apparent volume of distribution of propofol), but the drugs also have strongly synergistic pharmacodynamic interactions.
The pharmacokinetic model used for TCI administration of sufentanil infusion is the Gepts model with a target concentration at effect site of 0.5 ng ml−1. When used in combination with propofol, it provides similar intraoperative hemodynamics, awakening and extubation times to propofol–remifentanil but with delayed cognitive recovery [50].
Older pharmacokinetic models for DEX (Dyck and Talke) tended to underpredict the plasma concentration at higher concentrations. Hannivoort has recently published a new combined PK model for DEX [51]
Monitoring the Brain and Spinal Cord During Intravenous Anesthesia
Electrophysiological monitoring is applied during cranial and spine surgery for monitoring and for mapping. Monitoring is used to detect early changes in the transmission of the applied or recorded signal that can predict damage to the nervous tissue, whereas mapping is used to guide surgical excision treatment during epilepsy surgery and excision of tumors in important speech or motor function areas.
Electrocorticography (ECoG) is frequently used for brain mapping during epileptic foci resection. When this is performed under general anesthesia, propofol dosage should be carefully titrated (to 1.8–2.0 μg ml−1) to minimize the effect on the EEG , and an association with remifentanil (up to 10–15 ng ml−1) is useful to avoid hypertension during dura opening and foci removal.
Another field of application of TCI is during deep-brain stimulation (DBS ) a procedure increasingly used in treatment of Parkinson’s disease, dystonia, and certain psychiatric disorders such a depression and obsessive–compulsive disorders. An awake technique is often preferred in many institutions although in some patients there is the need for general anesthesia or monitored anesthesia care to avoid severe movements caused by the “off-drug state” in these patients. Propofol and remifentanil are commonly used for electrode insertion for conscious sedation [52]. An alternative is a low-dose infusion (0.3–0.6 μg/kg/h) of dexmedetomidine, which has the advantages that its non-GABA-mediated mechanism of action has minimal effects on microelectrode recordings, and is associated with hemodynamic stability and analgesic properties.
EEG is usually monitored during craniotomy for cerebral aneurysm clipping, during carotid endarterectomy, cardiopulmonary bypass, extracranial–intracranial bypass procedures, and pharmacological depression of the brain for “cerebral protection.” The EEG reflects the metabolic activity of the brain, and any factors adversely affecting the cerebral brain activity including anesthetics can impair the EEG activity. The use of TCI allows a constant level of anesthetic effect which can help to avoid misinterpretation of EEG depression caused by boluses or rapid changes in anesthetic level from true physiologic/pathologic insults to the cortex. A closed-loop communication between anesthesiologist, neurophysiologist, and neurosurgeon can guide the level of anesthesia during the critical phases of a complex surgery.
During spine surgery, somatosensory-evoked potentials (SSEP ) have been introduced for neurological investigation and monitoring of the integrity of the neural pathways during surgical procedures. This technique allows an online surveillance and early diagnosis of spinal cord dysfunction and aims to provide warning signals before any irreversible damage has occurred. Owing to the sensitivity and specificity of SSEP monitoring, the incidence of postoperative paraplegia can be significantly reduced [53, 54]. TCI allows the determination of the amount of administered propofol and the estimation of the plasma propofol concentration. This allows long-duration, stable anesthesia and the possibility of comparable anesthesia. The intraoperative within-case variability of SSEP is of relevance in performing neuromonitoring during surgery. It is of importance to determine the range of SSEP variability, which can be expected due to specific protocols of anesthesia [55].
Motor-evoked potentials (MEP ) are usually monitored to assess the motor pathways and avoid the need for a wake‐up test. A report of paraplegia, despite normal MEP obtained by direct stimulation of the spinal cord, suggests that MEP should be measured using transcranial stimulation of the motor cortex (tcMEP) . However, tcMEP recordings are markedly affected by anesthetics and a TCI infusion of propofol–remifentanil allowed recording and interpretation of tcMEP signals [56].
Auditory and visual-evoked potentials (VEP) are not commonly used during intraoperative neuromonitoring. The use of propofol strongly affects the amplitude of VEP and makes the use of these parameters during anesthesia for neurosurgical procedures unreliable [57].
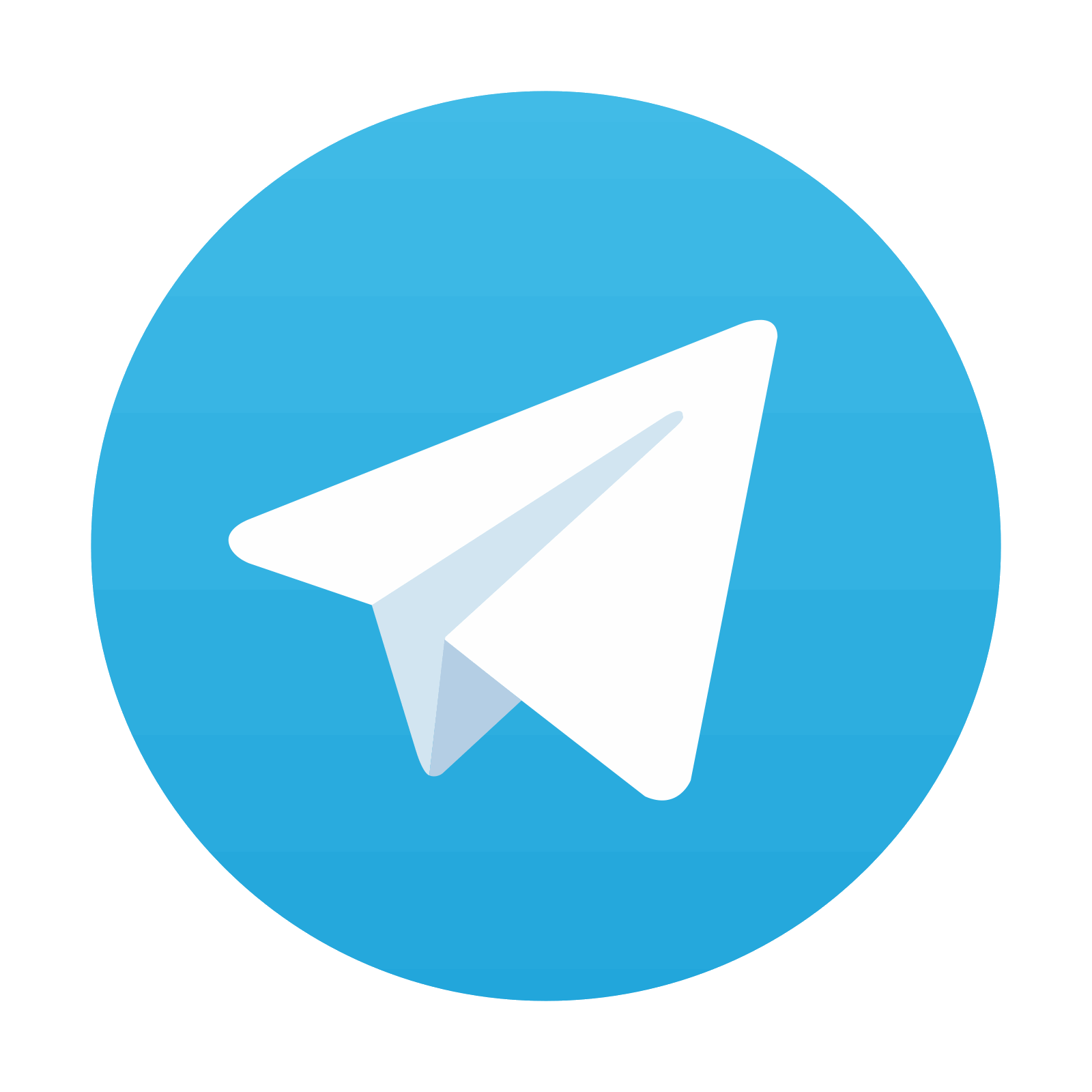
Stay updated, free articles. Join our Telegram channel
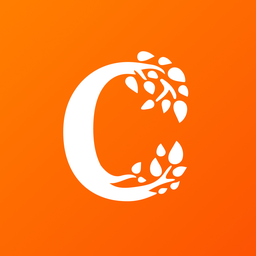
Full access? Get Clinical Tree
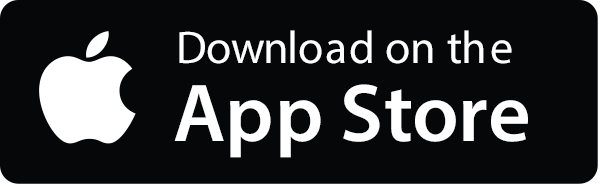
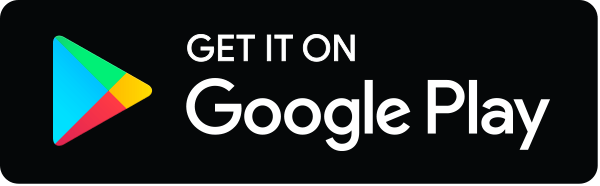