Clinical Uses
The principal use of phentolamine is the treatment of acute hypertensive emergencies, as may accompany intraoperative manipulation of a pheochromocytoma or autonomic nervous system hyperreflexia. Administration of phentolamine, 30 to 70 µg/kg IV (1 to 5 mg), produces a prompt but transient decrease in systemic blood pressure. A continuous infusion of phentolamine (0.1 to 2 mg per minute) may be used to maintain normal blood pressure during the intraoperative resection of a pheochromocytoma. Local infiltration with a phentolamine-containing solution (5 to 15 mg in 10 mL of normal saline) is appropriate when a sympathomimetic is accidentally administered extravascularly.
Phenoxybenzamine
Phenoxybenzamine is a haloalkylamine derivative that acts as a nonselective α-adrenergic antagonist by combining covalently with α-adrenergic receptors (Fig. 19-2). Blockade at postsynaptic α1 receptors is more intense than at α2 receptors.

Pharmacokinetics
Absorption of phenoxybenzamine from the gastrointestinal tract is incomplete. Onset of α-adrenergic blockade is slow, taking up to 60 minutes to reach peak effect even after IV administration. This delay in onset is due to the time required for structural modification of the phenoxybenzamine molecule, which is necessary to render the drug pharmacologically active. The elimination half-time of phenoxybenzamine is about 24 hours, emphasizing the likelihood of cumulative effects with repeated doses.
Cardiovascular Effects
Phenoxybenzamine administered to a supine, normovolemic patient in the absence of increased sympathetic nervous system activity produces little change in systemic blood pressure. Orthostatic hypotension, however, is prominent, especially in the presence of preexisting hypertension or hypovolemia. In addition, impairment of compensatory vasoconstriction results in exaggerated blood pressure decreases in response to blood loss or vasodilating drugs such as volatile anesthetics. Despite decreases in blood pressure, cardiac output is often increased and renal blood flow is not greatly altered unless preexisting renal vasoconstriction is present. Cerebral and coronary vascular resistances are not changed. α-Adrenergic blockade produced by maternal treatment may result in neonatal hypotension and respiratory distress in the first 72 hours of life.2
Noncardiac Effects
Phenoxybenzamine prevents the inhibitory action of epinephrine on the secretion of insulin. Catecholamine-induced glycogenolysis in skeletal muscles or lipolysis is not altered. Stimulation of the radial fibers of the iris is prevented, and miosis is a prominent component of the response to phenoxybenzamine. Sedation may accompany chronic phenoxybenzamine therapy. Nasal stuffiness is due to unopposed vasodilation in mucous membranes in the presence of α-adrenergic blockade.
Clinical Uses
Phenoxybenzamine, 0.5 to 1.0 mg/kg orally (prazosin is an alternative) is administered preoperatively to control blood pressure in patients with pheochromocytoma. Chronic α-adrenergic blockade, by relieving intense peripheral vasoconstriction, permits expansion of intravascular fluid volume as reflected by a decrease in the hematocrit. Excessive vasoconstriction with associated tissue ischemia, as accompanies hemorrhagic shock, may be reversed by phenoxybenzamine but only after intravascular fluid volume has been replenished.
Treatment of peripheral vascular disease characterized by intermittent claudication is not favorably influenced by α-adrenergic blockade because cutaneous rather than skeletal muscle blood flow is increased. The most beneficial clinical responses to α-adrenergic blockade are in diseases with a large component of cutaneous vasoconstriction, such as Raynaud’s disease, where smaller arteries that supply blood to skin narrow, limiting blood circulation to affected areas.
Yohimbine
Yohimbine is a selective antagonist at presynaptic α2 receptors, leading to enhanced release of norepinephrine from nerve endings. As a result, this drug may be useful in the treatment of the rare patient suffering from idiopathic orthostatic hypotension. In the past, impotence had been successfully treated with yohimbine in male patients with vascular, diabetic, and psychogenic origins. Yohimbine readily crosses the blood–brain barrier and may be associated with increased skeletal muscle activity and tremor. Excessive doses of yohimbine may produce tachycardia, hypertension, rhinorrhea, paresthesias, and dissociative states. Observations that α2-adrenergic agonists can decrease anesthetic requirements by actions on presynaptic α2 receptors in the central nervous system (CNS) suggest a possible interaction of yohimbine with volatile anesthetics.
Doxazosin
Doxazosin is approved for both treatment of hypertension and benign prostatic hypertrophy. It is a selective postsynaptic α1 receptor antagonist that is 65% bioavailable with oral administration. Peak levels of doxazosin are seen 2 to 3 hours following oral administration and effectively relaxes prostatic and vascular smooth muscle. Doxazosin is primarily metabolized in the liver by O-demethylation and excreted in the feces. The terminal elimination life of doxazosin is 22 hours and is recommended as a single daily dose in the morning.
Prazosin
Prazosin is a selective postsynaptic α1 receptor antagonist that leaves intact the inhibiting effect of α2 receptor activity on norepinephrine release from nerve endings. As a result, prazosin is less likely than nonselective α-adrenergic antagonists to evoke reflex tachycardia. Prazosin dilates both arterioles and veins. Following oral administration, the onset of action is approximately 30 minutes and the duration of action is about 4 to 6 hours. Elimination of prazosin is principally by hepatic metabolism.
Terazosin
α Blocker therapy of benign prostatic hypertrophy is based on α1-mediated innervation of prostatic smooth muscle that controls contraction of the prostate and obstruction of the bladder outlet. Terazosin is a long-acting orally effective α1-adrenergic antagonist that may be useful in the treatment of benign prostatic hyperplasia by virtue of its ability to relax prostatic smooth muscle.
Tamsulosin
Tamsulosin is an orally effective α1a-adrenergic antagonist that is indicated for the treatment of the signs and symptoms of benign prostatic hyperplasia. Side effects may include orthostatic hypotension, vertigo, and syncope. The clearance of tamsulosin is decreased in the presence of cimetidine.
Tolazoline
Tolazoline is a competitive nonselective α-adrenergic receptor antagonist. This drug has been used to treat persistent pulmonary hypertension of the newborn but its use for this purpose has been largely replaced by nitric oxide. Side effects of tolazoline include systemic hypotension with reflex tachycardia, cardiac dysrhythmias, and pulmonary and gastrointestinal hemorrhages. Tolazoline is excreted mainly unchanged by the kidneys.
α-Adrenergic Receptor Agonists
α2-Adrenergic receptor agonists bind selectively to presynaptic α2-adrenergic receptors and, by a negative feedback mechanism, decrease the release of norepinephrine from presynaptic nerve terminals and reduce sympathetic outflow with similar decreases in blood pressure as α1 antagonists. Most α2 receptors are found in the central nervous system especially in the brainstem and the locus ceruleus. Peripherial inhibition of α2 receptors can result in inhibition of insulin release and induction of glucagon from the pancreas. Clinical pharmacologic effects include hypotension, bradycardia, and central sedation with some mild effects of analgesia all related to the sympatholytic effects.
Mechanism of Action
α2-Adrenergic receptor agonists have selective affinity for α2-adrenergic receptors and act competitively. Binding of α2 agonists can be displaced from binding sites in the central nervous system resulting in reversal of the CNS effects. Withdrawal after even short-term use can result in a rebound effect with a dramatic increase in sympathetic outflow causing elevations in heart rate and hypertension to even dangerous levels.
Clonidine
Administration results in dose-dependent decreases in heart rate and blood pressure and is used clinically to treat resistant hypertension and tremors from central stimulant medications. Clonidine is a partial agonist of α2 receptors with a 400:1 α2:α1 receptor preference. Clonidine is available in an intravenous, oral, and transdermal preparation and is metabolized in the liver but is excreted mostly unchanged in the urine and to a lesser extent in the bile and feces. Terminal half-life is approximately 12 to 16 hours but can be extremely variable with any liver or kidney dysfunction.
Dexmedetomidine
Dexmedetomidine is a selective α2 agonist with a 1,600:1 preference for α2 receptors. It is intravenously administered as an infusion from 0.1 to 1.5 µg/kg/minute with a terminal elimination half-life of 2 hours. Most often, this α2 agonist is used in the intensive care and operating room settings as a sedative and analgesic due to its central sympatholytic effects. Dexmedetomidine undergoes extensive biotransformation in the liver and is excreted mostly in the urine; liver impairment can dramatically increase plasma levels and duration of action due to significantly decreased metabolism during infusion. It’s potent binding and short half-life can induce physiologic dependence and result in the aforementioned withdrawal phenomenon after only days of administration resulting in tachycardia, hypertension, and anxiety. Interestingly, large intravenous boluses (0.25 to 1 µg/kg over 3 to 5 minutes) result in a paradoxical hypertension with a decrease in heart rate and resembles phenylephrine and is the resultant effect of crossover α1 stimulation.
β-Adrenergic Receptor Antagonists
β-Adrenergic receptor antagonists bind selectively to β-adrenergic receptors and interfere with the ability of catecholamines or other sympathomimetics to provoke β responses. Drug-induced β-adrenergic blockade prevents the effects of catecholamines and sympathomimetics on the heart and smooth muscles of the airways and blood vessels. β Antagonist therapy should be continued throughout the perioperative period to maintain desirable drug effects and to avoid the risk of sympathetic nervous system hyperactivity associated with abrupt discontinuation of these drugs. Propranolol is the standard β-adrenergic antagonist drug to which all other β-adrenergic antagonists are compared.
Mechanism of Action
β-Adrenergic receptor antagonists exhibit selective affinity for β-adrenergic receptors, where they act by competitive inhibition. Binding of antagonist drugs to β-adrenergic receptors is reversible such that the drug can be displaced from the occupied receptors if sufficiently large amounts of agonist become available. Competitive antagonism causes a rightward displacement of the dose-response curve for the agonist, but the slope of the curve remains unchanged, emphasizing that sufficiently large doses of the agonist may still exert a full pharmacologic effect. Chronic administration of β-adrenergic antagonists is associated with an increase in the number of β-adrenergic receptors.
β-Adrenergic receptors are G protein–coupled receptors and their occupancy by agonists (norepinephrine, epinephrine) stimulates G proteins that in turn activate adenylate cyclase to produce cyclic adenosine monophosphate (cAMP). Protein kinases are activated by cAMP, which phosphorylates proteins including L-type voltage-dependent calcium channels and troponin C in a variety of tissues (especially myocardium). The net effect of β-adrenergic stimulation in the heart is to produce positive chronotropic, inotropic, and dromotropic effects and these are the responses that are blunted by β-adrenergic receptor antagonists. It is estimated that about 75% of β receptors in the myocardium are β1, whereas β2 receptors account for about 20% of β receptors.
Structure–Activity Relationships
β-Adrenergic antagonists are derivatives of the β agonist drug isoproterenol (Fig. 19-3). Substitutions on the benzene ring determine whether the drug acts on β-adrenergic receptors as an antagonist or agonist. The levorotatory forms of β antagonists and agonists are more potent than the dextrorotatory forms. For example, the dextrorotatory isomer of propranolol has less than 1% of the potency of the levorotatory isomer for blocking β-adrenergic receptors.

Classification
β-Adrenergic receptor antagonists are classified as nonselective for β1 and β2 receptors (propranolol, nadolol, timolol, pindolol) and cardioselective (metoprolol, atenolol, acebutolol, betaxolol, esmolol, bisoprolol) for β1 receptors (Tables 19-1 and 19-2). It is important to recognize that β receptor selectivity is dose dependent and is lost when large doses of the antagonist are administered. This emphasizes that selectivity should not be interpreted as specificity for a specific type of β-adrenergic receptor. β-Adrenergic antagonists are further classified as partial or pure antagonists on the basis of the presence or absence of intrinsic sympathomimetic activity (see Tables 19-1 and 19-2). Drugs that exhibit cardiac selectivity for β1 receptors (cardioselective) are better suited for administration to patients with asthma and reactive airway disease. Theoretically, cardioselective drugs are better suited for treatment of patients with essential hypertension as these drugs lack inhibition of peripheral β2 receptors that produce vasodilation.


β1 Receptor blockade is associated with slowing of the sinus rate, slowing of conduction of cardiac impulses through the atrioventricular node, and a decrease in inotropy. These effects are relatively greater during activity than during rest. The result is a decrease in myocardial oxygen demand, with a subsequent decrease in the occurrence of myocardial ischemia during exercise. The decrease in heart rate also increases diastolic perfusion time, which may enhance myocardial perfusion. β2 Receptor blockade increases the risk of bronchospasm in patients with reactive airway disease and may worsen the clinical symptoms of peripheral vascular disease.
β-Adrenergic antagonists may produce some degree of membrane stabilization in the heart (inhibition of propagation of action potentials across the cell membrane similar to sodium channel blockers that are class I antiarrhythmics) and thus resemble quinidine. This membrane stabilization effect, however, is detectable only at plasma concentrations that are far higher than needed to produce clinically adequate β-adrenergic blockade.
Pharmacokinetics
The principal difference in pharmacokinetics between all the β-adrenergic receptor antagonists is the elimination half-time ranging from brief for esmolol (about 10 minutes) to hours for the other drugs (see Table 19-1). Elimination half-time is considered in the perioperative period when redosing intervals are being developed or when conversion to another β-adrenergic receptor drug is planned. Among the β-adrenergic receptor antagonists, only propranolol is highly protein bound. The volume of distribution of these drugs is high and they are rapidly distributed following IV administration.
β-Adrenergic receptor antagonists are eliminated by several different pathways and this must be considered in the presence of renal and/or hepatic dysfunction (see Table 19-1). The therapeutic plasma concentration varies greatly among these drugs and between patients (interpatient variability). Explanations for interpatient variability include differences in basal sympathetic nervous system tone, flat dose-response curves for the drug so changes in plasma concentrations evoke minimal changes in pharmacologic effects, impact of active metabolites, and genetic differences in β-adrenergic receptors that influence how an individual patient responds to a given drug and plasma concentration.
Propranolol
Propranolol is a nonselective β-adrenergic receptor antagonist that lacks intrinsic sympathomimetic activity and thus is a pure antagonist (see Table 19-1). Antagonism of β1 and β2 receptors produced by propranolol is about equal. As the first β-adrenergic antagonist introduced clinically, propranolol is the standard drug to which all β-adrenergic antagonists are compared. Typically, propranolol is administered in stepwise increments until physiologic plasma concentrations have been attained, as indicated by a resting heart rate of 55 to 60 beats per minute.
Cardiac Effects
The most important pharmacologic effects of propranolol are on the heart. Because of β1-receptor blockade, propranolol decreases heart rate and myocardial contractility, resulting in decreased cardiac output. These effects on heart rate and cardiac output are especially prominent during exercise or in the presence of increased sympathetic nervous system activity. Heart rate slowing induced by propranolol lasts longer than the negative inotropic effects, suggesting a possible subdivision of β1 receptors. Concomitant blockade of β2 receptors by propranolol increases peripheral vascular resistance, including coronary vascular resistance. Although prolongation of systolic ejection and dilatation of the cardiac ventricles caused by propranolol increases myocardial oxygen requirements, the oxygen-sparing effects of decreased heart rate and myocardial contractility predominate. As a result, propranolol may relieve myocardial ischemia, even though drug-induced increases in coronary vascular resistance oppose coronary blood flow. Sodium retention associated with propranolol therapy most likely results from intrarenal hemodynamic changes that accompany drug-induced decreases in cardiac output.
Pharmacokinetics
Propranolol is rapidly and almost completely absorbed from the gastrointestinal tract, but systemic availability of the drug is limited by extensive hepatic first-pass metabolism, which may account for 90% to 95% of the absorbed dose. There is considerable individual variation in the magnitude of hepatic first-pass metabolism, accounting for up to 20-fold differences in plasma concentrations of propranolol in patients after oral administration of comparable doses.3 Hepatic first-pass metabolism is the reason the oral dose of propranolol (40 to 800 mg per day) must be substantially greater than the IV dose (0.05 mg/kg given in increments of 0.5 to 1.0 mg every 5 minutes). Propranolol is not effective when administered intramuscularly.
Protein Binding
Propranolol is extensively bound (90% to 95%) to plasma proteins. Heparin-induced increases in plasma concentrations of free fatty acids due to increased lipoprotein lipase activity result in decreased plasma protein binding of propranolol (Fig. 19-4).4 In addition, hemodilution that occurs when cardiopulmonary bypass is initiated may alter protein binding of drugs because of the nonphysiologic protein concentration in the pump prime.

Metabolism
Clearance of propranolol from the plasma is by hepatic metabolism. An active metabolite, 4-hydroxypropranolol, is detectable in the plasma after oral administration of propranolol. Indeed, cardiac β-blocking activity after equivalent doses of propranolol is greater after oral than after IV administration, presumably reflecting the effects of this metabolite, which is equivalent in activity to the parent compound. The elimination half-time of propranolol is 2 to 3 hours, whereas that of 4-hydroxypropranolol is even briefer. The plasma concentration of propranolol or the total dose does not correlate with its therapeutic effects. Furthermore, the assay for propranolol may not detect 4-hydroxypropranolol.
Elimination of propranolol is greatly decreased when hepatic blood flow decreases. In this regard, propranolol may decrease its own clearance rate by decreasing cardiac output and hepatic blood flow. Alterations in hepatic enzyme activity may also influence the rate of hepatic metabolism. Renal failure does not alter the elimination half-time of propranolol, but accumulation of metabolites may occur.
Clearance of Local Anesthetics
Propranolol decreases clearance of amide local anesthetics by decreasing hepatic blood flow and inhibiting metabolism in the liver.5 For example, in humans, propranolol causes clearance to be decreased to a much greater extent (46%) than would be predicted from a maximum 25% decrease in hepatic blood flow, implying that drug metabolism in the liver has also been affected.6 Bupivacaine clearance is relatively insensitive to changes in hepatic blood flow (low-extraction drug), suggesting that the 35% decrease in clearance of this local anesthetic reflects propranolol-induced decreases in metabolism (Fig. 19-5).5 Because clearance of drugs with low extraction ratios is inversely related to plasma protein binding, an increase in bupivacaine binding to α1-acid glycoprotein (responsible for 90% binding of bupivacaine) caused by propranolol could explain a decrease in clearance. Nevertheless, propranolol does not alter α1-acid glycoprotein concentrations.6 It is conceivable that systemic toxicity of bupivacaine could be increased by propranolol and presumably other β antagonists that interfere with the clearance of this and other amide local anesthetics.

Clearance of Opioids
Pulmonary first-pass uptake of fentanyl is substantially decreased in patients being treated chronically with propranolol.7 As a result, two to four times as much injected fentanyl enters the systemic circulation in the time period immediately after injection. This response most likely reflects the ability of one basic lipophilic amine (propranolol) to inhibit the pulmonary uptake of a second basic lipophilic amine (fentanyl).
Nadolol and Pindolol
Nadolol and pindolol are nonselective β-adrenergic receptor antagonists; nadolol is unique in that its long duration of action permits once daily administration.
Pharmacokinetics
Nadolol is slowly and incompletely absorbed (an estimated 30%) from the gastrointestinal tract. Metabolism does not occur, with about 75% of the drug being excreted unchanged in urine and the remainder in bile. Therefore, wide individual variations in plasma concentrations that occur with nadolol cannot be attributed to differences in metabolism, as occur with propranolol. The elimination half-time is 20 to 40 hours, accounting for the need to administer this drug only once a day. The elimination half-time of pindolol is 3 to 4 hours, and this is increased to longer than 11 hours in patients with renal failure.
Timolol
Timolol is a nonselective β-adrenergic receptor antagonist that is as effective as propranolol for various therapeutic indications. In addition, timolol is effective in the treatment of glaucoma because of its ability to decrease intraocular pressure, presumably by decreasing the production of aqueous humor. Timolol is administered as eyedrops in the treatment of glaucoma, but systemic absorption may be sufficient to cause resting bradycardia and increased airway resistance. Indeed, bradycardia and hypotension that are refractory to treatment with atropine have been observed during anesthesia in pediatric and adult patients receiving topical timolol with or without pilocarpine.8 Timolol may be associated with impaired control of ventilation in neonates, resulting in unexpected postoperative apnea.9 Immaturity of the neonate’s blood–brain barrier may facilitate access of this drug to the CNS.
Pharmacokinetics
Timolol is rapidly and almost completely absorbed after oral administration. Nevertheless, extensive first-pass hepatic metabolism limits the amount of drug reaching the systemic circulation to about 50% of that absorbed from the gastrointestinal tract. Protein binding of timolol is not extensive. The elimination half-time is about 4 hours.
Metoprolol
Metoprolol is a selective β1-adrenergic receptor antagonist that prevents inotropic and chronotropic responses to β-adrenergic stimulation. Conversely, bronchodilator, vasodilator, and metabolic effects of β2 receptors remain intact such that metoprolol is less likely to cause adverse effects in patients with chronic obstructive airway disease or peripheral vascular disease and in patients vulnerable to hypoglycemia. It is important to recognize, however, that selectivity is dose related, and large doses of metoprolol are likely to become nonselective, exerting antagonist effects at β2 receptors as well as β1 receptors. Indeed, airway resistance may increase in asthmatic patients treated with metoprolol, although the magnitude of increase will be less than that evoked by propranolol. Furthermore, metoprolol-induced increases in airway resistance are more readily reversed with β2-adrenergic agonists such as terbutaline.
Pharmacokinetics
Metoprolol is readily absorbed from the gastrointestinal tract, but this is offset by substantial hepatic first-pass metabolism such that only about 40% of the drug reaches the systemic circulation. Protein binding is low; it is estimated to account for about 10% of the drug. None of the hepatic metabolites have been identified as active. A small amount (<10%) of the drug appears unchanged in urine. There are two available oral formulation of metoprolol: metoprolol tartrate and metoprolol succinate. The elimination half-time of metoprolol tartrate is 2 to 3 hours and correlates to a need for at least a twice daily dosing strategy with a thrice daily or four times daily strategy providing a more reliable control of heart rate. Metoprolol succinate results in a significantly extended time to peak concentrations and overall decreased plasma concentrations compared to metoprolol tartrate at equal daily doses. Metoprolol succinate elimination half-time is 5 to 7 hours and can be used in once daily dosing regimens but in some patients can still result in β-blocker withdrawal tachycardia at 24 hours necessitating twice daily dosing. Overall, plasma concentrations of metoprolol do not correlate with therapeutic effects of the drug.
Atenolol
Atenolol is the most selective β1-adrenergic antagonist that may have specific value in patients in whom the continued presence of β2 receptor activity is desirable. In patients at risk for coronary artery disease who must undergo noncardiac surgery, treatment with IV atenolol before and immediately after surgery, followed by oral therapy during the remainder of the hospitalization, decreases mortality and the incidence of cardiovascular complications for as long as 2 years.10 Perioperative administration of atenolol to patients at high risk for coronary artery disease significantly decreases the incidence of postoperative myocardial ischemia.11
The antihypertensive effect of atenolol is prolonged, permitting this drug to be administered once daily for the treatment of hypertension. Like nadolol, atenolol does not enter the CNS in large amounts, but fatigue and mental depression still occur. Unlike nonselective β-adrenergic antagonists, atenolol does not appear to potentiate insulin-induced hypoglycemia and can thus be administered with caution to patients with diabetes mellitus whose hypertension is not controlled by other antihypertensives.
Pharmacokinetics
About 50% of an orally administered dose of atenolol is absorbed from the gastrointestinal tract, with peak concentrations occurring 1 to 2 hours after oral administration. Atenolol undergoes little or no hepatic metabolism and is eliminated principally by renal excretion. The elimination half-time is 6 to 7 hours; this may increase to more than 24 hours in patients with renal failure. Therapeutic plasma concentrations of atenolol are 200 to 500 ng/mL.
Betaxolol
Betaxolol is a cardioselective β1-adrenergic antagonist with no intrinsic sympathomimetic activity and weak membrane-stabilizing activity. High doses can be expected to produce some β2-adrenergic antagonist effects on bronchial and vascular smooth muscle. Absorption after an oral dose is nearly complete. Its elimination half-time is 11 to 22 hours, making it one of the longest acting β-adrenergic antagonists. Clearance is primarily by metabolism, with renal elimination contributing less to overall removal of the drug from the plasma. A single oral dose daily is useful for the treatment of hypertension. A topical preparation is used as an alternative to timolol for treatment of chronic open-angle glaucoma. The risk of bronchoconstriction in patients with airway hyperreactivity may be less with betaxolol than with timolol.
Bisoprolol
Bisoprolol is a β1 selective antagonist drug without significant intrinsic agonist activity. The elimination half-time is 9 to 12 hours. Bisoprolol is eliminated equally by renal and nonrenal mechanisms. Metabolites are pharmacologically inactive. The most prominent pharmacologic effect of bisoprolol is a negative chronotropic effect. Bisoprolol is useful in the treatment of essential hypertension and has been shown to improve survival in patients with mild to moderate congestive heart failure (see Table 19-2).
Nebivolol
Nebivolol is a very potent and selective β1-antagonist drug12 and is 3.5 times more selective than bisoprolol13 without significant intrinsic agonist activity. Above doses of 10 mg, or in those patients with certain CYP polymorphisms, Nebivolol can exhibit low β2 antagonism as well. With an elimination half-time of 12 to 19 hours, it is a once daily regimen that allows accidental delay in subsequent doses without withdrawal. Nebivolol is equally eliminated in the urine unchanged and in the feces as a inactive metabolite. The most prominent pharmacologic effect of bisoprolol is a negative chronotropic effect and is currently approved and useful in the treatment of essential hypertension.
Esmolol
Esmolol is a rapid-onset and short-acting selective β1-adrenergic receptor antagonist that is administered only IV (see Fig. 19-3). After a typical initial dose of 0.5 mg/kg IV over about 60 seconds, the full therapeutic effect is evident within 5 minutes, and its action ceases within 10 to 30 minutes after administration is discontinued. These characteristics make esmolol a useful drug for preventing or treating adverse systemic blood pressure and heart rate increases that occur intraoperatively in response to noxious stimulation, as during tracheal intubation. For example, esmolol, 150 mg IV, administered about 2 minutes before direct laryngoscopy and tracheal intubation provides reliable protection against increases in both heart rate and systolic blood pressure, which predictably accompanies tracheal intubation (Fig. 19-6).14

Lidocaine or fentanyl is effective in blunting the increase in systolic blood pressure associated with laryngoscopy and tracheal intubation, but heart rate is not influenced (see Fig. 19-6).14 Other reports describe prevention of perioperative tachycardia and hypertension with esmolol, 100 to 200 mg IV, administered over 15 seconds before the induction of anesthesia.15,16 Prior administration of esmolol, 500 µg/kg/minute IV, to patients undergoing electroconvulsive therapy with anesthesia induced by methohexital plus succinylcholine results in attenuation of the heart rate increase and a decrease in the length of the electrically induced seizures.17 Esmolol has been used during resection of pheochromocytoma and may be useful in the perioperative management of thyrotoxicosis, pregnancy-induced hypertension, and epinephrine- or cocaine-induced cardiovascular toxicity.18–22 Conversely, treatment of excessive sympathetic nervous system activity produced by cocaine or systemic absorption of topical or subcutaneous epinephrine with β-adrenergic receptor antagonists has been associated with fulminant pulmonary edema and irreversible cardiovascular collapse.23 It is possible that acute drug-induced β-receptor antagonism removes the ability of the heart to increase heart rate and myocardial contractility to compensate for catecholamine-induced increases in left ventricular afterload. In this regard, persistent symptomatic systemic hypertension owing to catecholamine-induced sympathetic nervous system stimulation is more safely treated with a peripheral vasodilator drug such as sodium nitroprusside or nitroglycerin. Detrimental effects of catecholamine release during anesthesia in patients with hypertrophic obstructive cardiomyopathy and in patients experiencing hypercyanotic spells associated with tetralogy of Fallot may be blunted by administration of esmolol.24
The β1 selectivity of esmolol may unmask β2-mediated vasodilation by epinephrine-secreting tumors. Administration of esmolol to patients chronically treated with β-adrenergic antagonists has not been observed to produce additional negative inotropic effects.25 The presumed reason for this observation is that esmolol, in the dose used, does not occupy sufficient additional β receptors to produce detectable increases in β blockade. Likewise, esmolol infused during cardiopulmonary bypass is not associated with adverse effects after discontinuation of cardiopulmonary bypass.26
Esmolol (1 mg/kg IV followed by 250 µg/kg/minute V) significantly decreases the plasma concentration of propofol required to prevent patient movement in response to a surgical skin incision.27 This effect does not seem to be explained by a pharmacokinetic interaction between the two drugs.
Pharmacokinetics
Esmolol is available for IV administration only. The only other β-adrenergic antagonists that may be administered IV are propranolol and metoprolol. The commercial preparation of esmolol is buffered to pH 4.5 to 5.5, which may be one of the factors responsible for pain on injection. The drug is compatible with commonly used IV solutions and nondepolarizing neuromuscular blocking drugs. The elimination half-time of esmolol is about 9 minutes, reflecting its rapid hydrolysis in the blood by plasma esterases that is independent of renal and hepatic function.28 Less than 1% of the drug is excreted unchanged in urine, and about 75% is recovered as an inactive acid metabolite. Clinically insignificant amounts of methanol also occur from the hydrolysis of esmolol. Plasma esterases responsible for the hydrolysis of esmolol are distinct from plasma cholinesterase, and the duration of action of succinylcholine is not predictably prolonged in patients treated with esmolol.29 Evidence of the short duration of action of esmolol is return of the heart rate to predrug levels within 15 minutes after discontinuing the drug. Indeed, plasma concentrations of esmolol are usually not detectable 15 minutes after discontinuing the drug. Poor lipid solubility limits transfer of esmolol into the CNS or across the placenta.19
Side Effects
The side effects of β-adrenergic antagonists are similar for all available drugs, although the magnitude may differ depending on their selectivity and the presence or absence of intrinsic sympathomimetic activity. β-Adrenergic antagonists exert their most prominent pharmacologic effects as well as side effects on the cardiovascular system. These drugs may also alter airway resistance, carbohydrate and lipid metabolism, and the distribution of extracellular ions. β-Adrenergic antagonists may cause hypoglycemia.30 Additive effects between drugs used for anesthesia and β-adrenergic antagonists may occur. β-Adrenergic antagonists penetrate the blood–brain barrier and cross the placenta. Gastrointestinal side effects include nausea, vomiting, and diarrhea. Fever, rash, myopathy, alopecia, and thrombocytopenia have been associated with chronic β-adrenergic antagonist treatment. β-Adrenergic antagonists have been reported to decrease plasma concentrations of high-density lipoproteins and to increase triglyceride and uric acid levels.
The principal contraindication to administration of β-adrenergic antagonists is preexisting atrioventricular heart block or cardiac failure not caused by tachycardia. Administration of β-adrenergic antagonists to hypovolemic patients with compensatory tachycardia may produce profound hypotension.31 Nonselective β-adrenergic antagonists or high doses of selective β-adrenergic antagonists are not recommended for administration to patients with chronic obstructive airway disease. In patients with diabetes mellitus, there is the risk that β-adrenergic blockade may mask the signs of hyperglycemia and thus delay its clinical recognition.
Cardiovascular System
β-Adrenergic antagonists produce negative inotropic and chronotropic effects. In addition, the conduction speed of cardiac impulses through the atrioventricular node is slowed and the rate of spontaneous phase 4 depolarization is decreased. Preexisting atrioventricular heart block due to any cause may be accentuated by β-adrenergic antagonists.
The cardiovascular effects of β-adrenergic blockade reflect removal of sympathetic nervous system innervation to the heart (β1 blockade) and not membrane stabilization, which occurs only at high plasma concentrations of the antagonist drug. In addition, nonselective β-adrenergic blockade resulting in β2-adrenergic receptor antagonism may impede left ventricular ejection due to unopposed α-adrenergic receptor–mediated peripheral vasoconstriction. The magnitude of cardiovascular effects produced by β-adrenergic antagonists is greatest when preexisting sympathetic nervous system activity is increased, as during exercise or in patients in cardiac failure. Indeed, the tachycardia of exercise is consistently attenuated by β-adrenergic antagonists. Furthermore, administration of a β antagonist may precipitate cardiac failure in a patient who was previously compensated. Resting bradycardia is minimized and cardiac failure is less likely to occur when a partial β-adrenergic antagonist with intrinsic sympathomimetic activity is administered. Acute cardiac failure is rare with oral administration of β-adrenergic antagonists.
Classically, β-adrenergic antagonists prevent inotropic and chronotropic effects of isoproterenol as well as baroreceptor-mediated increases in heart rate evoked by decreases in systemic blood pressure in response to peripheral vasodilator drugs. Conversely, the influence of β-adrenergic antagonists on the cardiac-stimulating effects of calcium, glucagon, and digitalis preparations is not detectable. Likewise, β-adrenergic antagonists do not alter the response to α-adrenergic agonists such as epinephrine or phenylephrine. Indeed, the pressor effect of epinephrine is enhanced because the nonselective β antagonists prevent the β2 vasodilating effect of epinephrine and leave unopposed its α-adrenergic effect. The presence of unopposed α-adrenergic–induced vasoconstriction may provoke paradoxical hypertension and may even precipitate cardiac failure in the presence of diseased myocardium that cannot respond to sympathetic nervous system stimulation because of β-adrenergic blockade. Unexpected hypertension has occurred in patients receiving clonidine who subsequently receive a nonselective β-adrenergic antagonist.32 Presumably, blockade of the vasodilating effect normally produced by activity of β2 receptors leaves unopposed α-adrenergic effects to provoke peripheral vasoconstriction with resulting hypertension.
Patients with peripheral vascular disease do not tolerate well the peripheral vasoconstriction associated with β2 receptor blockade produced by nonselective β-adrenergic antagonists. Indeed, the development of cold hands and feet is a common side effect of β blockade. Vasospasm associated with Raynaud’s disease is accentuated by propranolol.
The principal antidysrhythmic effect of β-adrenergic blockade is to prevent the dysrhythmogenic effect of endogenous or exogenous catecholamines or sympathomimetics. This reflects a decrease in sympathetic nervous system activity. Membrane stabilization is probably of little importance in the antidysrhythmic effects produced by usual doses of β-adrenergic antagonists.
Treatment of Excess Myocardial Depression
The usual clinical manifestations of excessive myocardial depression produced by β-adrenergic blockade include bradycardia, low cardiac output, hypotension, and cardiogenic shock.33 Bronchospasm and depression of ventilation may also be associated with an overdose of β-adrenergic antagonist drugs. Seizures and prolonged intraventricular conduction of cardiac impulses are thought to be the result of local anesthetic properties of certain β-adrenergic antagonists (see Table 19-1). Hypoglycemia is a rare manifestation of β-adrenergic antagonist overdose.
Excessive bradycardia and/or decreases in cardiac output due to drug-induced β blockade should be treated initially with atropine in incremental doses of 7 µg/kg IV. Atropine is likely to be effective by blocking vagal effects on the heart and thus unmasking any residual sympathetic nervous system innervation. If atropine is ineffective, drugs to produce direct positive chronotropic and inotropic effects are indicated. For example, continuous infusion of the nonselective β-adrenergic agonist isoproterenol, in doses sufficient to overcome competitive β blockade, is appropriate. The necessary dose of isoproterenol may be 2 to 25 µg per minute IV (60 µg per minute IV was not effective in one report), which is 5 to 20 times the necessary dose in the absence of β blockade.33 When a pure β-adrenergic antagonist is responsible for excessive cardiovascular depression, a pure β1-adrenergic agonist such as dobutamine is recommended because isoproterenol, with β1– and β2-adrenergic agonist effects, could produce vasodilation before its inotropic effect develops. Dopamine is not recommended because α-adrenergic–induced vasoconstriction is likely to occur with the high doses required to overcome β blockade.
Glucagon administered to adults, 1 to 10 mg IV followed by 5 mg per hour IV, effectively reverses myocardial depression produced by β-adrenergic antagonists at normal doses because these drugs do not exert their effects by means of β-adrenergic receptors. For example, glucagon stimulates adenylate cyclase and increases intracellular cAMP concentrations independent of β-adrenergic receptors.33 Calcium chloride, 250 to 1,000 mg IV, may also act independent of β-adrenergic receptors to offset excessive cardiovascular depression produced by β-adrenergic antagonists. Glucagon appears to be particularly effective in the presence of life-threatening bradycardia and has been described as the drug of choice to treat massive β-adrenergic antagonist overdose.33
In the presence of bradycardia that is unresponsive to pharmacologic therapy, it may be necessary to place a transvenous artificial cardiac pacemaker. Hemodialysis should be reserved to remove minimally protein-bound, renally excreted β-adrenergic antagonists in patients refractory to pharmacologic therapy.
Airway Resistance
Nonselective β-adrenergic antagonists such as propranolol consistently increase airway resistance as a manifestation of bronchoconstriction due to blockade of β2 receptors. These airway resistance effects are exaggerated in patients with preexisting obstructive airway disease. Because bronchodilation is a β2-adrenergic agonist response, selective β1-adrenergic antagonists such as metoprolol and esmolol are less likely than propranolol to increase airway resistance.
Metabolism
β-Adrenergic antagonists alter carbohydrate and fat metabolism. For example, nonselective β-adrenergic antagonists such as propranolol interfere with glycogenolysis that ordinarily occurs in response to release of epinephrine during hypoglycemia. This emphasizes the need for β2 receptor activity in glycogenolysis. Furthermore, tachycardia, which is an important warning sign of hypoglycemia in insulin-treated diabetics, is blunted by β-adrenergic antagonists. For this reason, nonselective β-adrenergic antagonists are not recommended for administration to patients with diabetes mellitus who may be at risk for developing hypoglycemia because of treatment with insulin or oral hypoglycemics. Altered fat metabolism is evidenced by failure of sympathomimetics or sympathetic nervous system stimulation to increase plasma concentrations of fatty-free acids in the presence of β-adrenergic blockade.
Distribution of Extracellular Potassium
Distribution of potassium across cell membranes is influenced by sympathetic nervous system activity as well as insulin. Specifically, stimulation of β2-adrenergic receptors seems to facilitate movement of potassium intracellularly. As a result, β-adrenergic blockade inhibits uptake of potassium into skeletal muscles, and the plasma concentration of potassium may be increased. Indeed, increases in the plasma concentration of potassium associated with infusion of this ion are greater in the presence of β-adrenergic blockade produced by propranolol (Fig. 19-7).34 In animals, increases in the plasma concentration of potassium after administration of succinylcholine last longer when β-adrenergic blockade is present.35 In view of the speculated role of β2 receptors in regulating plasma concentrations of potassium, it is likely that selective β1-adrenergic antagonists would impair skeletal muscle uptake of potassium less than nonselective β-adrenergic antagonists.

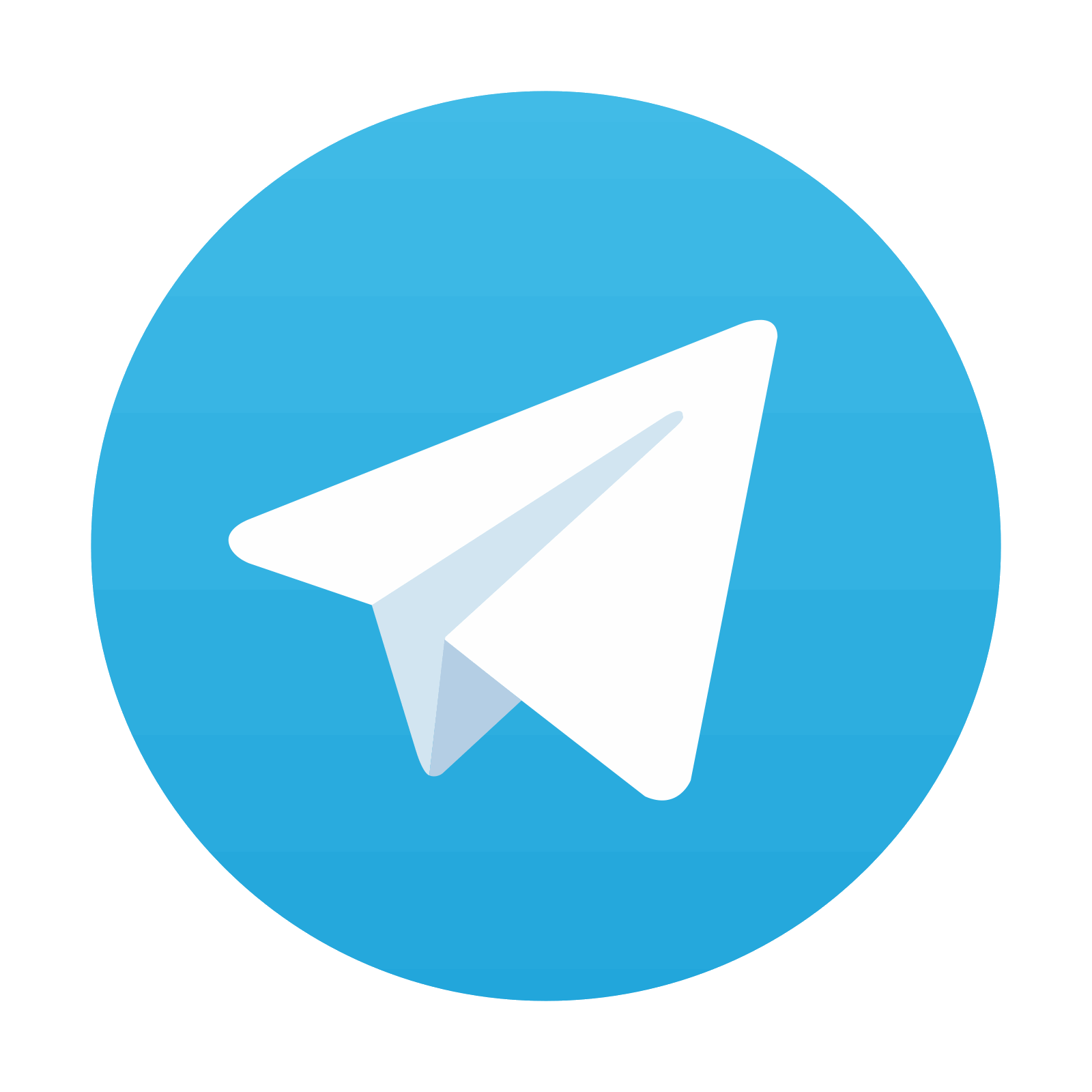
Stay updated, free articles. Join our Telegram channel
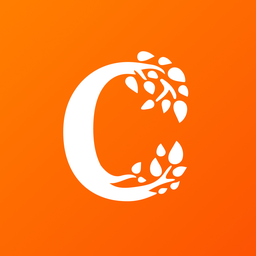
Full access? Get Clinical Tree
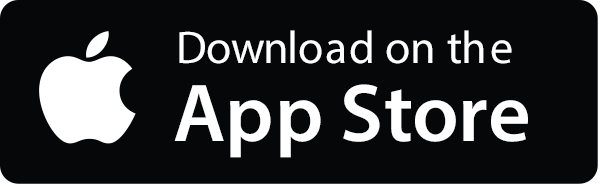
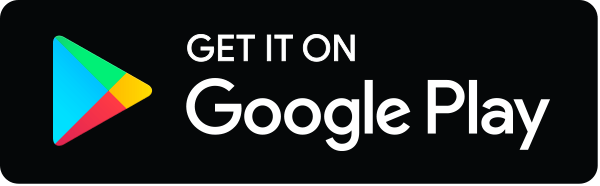