Substrates of Spinal Cord Nociceptive Processing
Jennifer J. Deberry
Alan Randich
Timothy J. Ness
The spinal cord and brainstem nuclei are home to second-order neurons, the first step of central nervous system (CNS) processing. As the first site of sensory integration and modulation, second-order neurons are more than a simple relay, and any plan for the treatment of nociception must understand the critical role these neurons play in the formation of painful sensation. The second-order neuron encodes afferent input from multiple sites and often multiple modalities into a message that is sent to other parts of the CNS. Those other parts of the CNS, in turn, modify the second-order neuron through both excitatory and inhibitory mechanisms. These modifying influences are the subject of the next chapter, whereas the present chapter focuses on the neuroanatomical and neurochemical characteristics of these spinal substrates.
Although it seems like a simple statement that pain-related second-order neurons are the neurons which receive primary afferent input related to tissue damage (i.e., from nociceptors), it must be accepted that this statement may or may not be wholly true because in certain pathologic states, pain can be evoked by non-tissue-damaging stimuli (allodynia). It is unfortunate that there has been a tendency in pain-related research to turn common observations into overgeneralizations, and so an attempt will be made in this chapter to be precise when possible. Sometimes, “assumptions” related to neuronal substrates of sensation have been necessarily used as “premises” on which to build scientific logic. The primary premise on which this chapter is based is that all nociceptive second-order neurons receive nociceptive primary afferent input as one of their excitatory modalities. If one can accept that premise, then one can identify where the neurons receiving such input are located and can further identify where these neurons send the information.
Defining Nociceptive Systems
MODELS OF PAIN PROCESSING
Pain is both a sensation and responses to that sensation. The sensory component of pain is described in terms of tissue damage (e.g., cutting, burning, rending) even when tissue damage is not occurring, and so the sensation of pain is defined as nociception. The sensory systems of our body which encode for nociception can be modeled in two main ways: (1) as a system which is specific for pain (specificity theory) or (2) as a system that requires a pattern of neuronal activation to occur for the experience of pain to be generated (pattern theory). The simplest and oldest of the pattern theories is that which suggests pain is due to high intensities of input that are independent of modality (intensity theory). Each of these theories (or the multiple variants thereof) has prominent proponents who can make persuasive arguments that focus on subsets of data that support their particular view. Each knowledgeable person must derive their own model system which is ideally based on characterized human phenomena and which must clearly go beyond simple models.
As is apparent from the preceding chapter, primary afferent neurons with sensory endings have been characterized using functional and neurochemical methods. A subset of these primary afferents with sensory endings in cutaneous tissues is only activated by (and so specific for) pain-producing stimuli. Although these afferents may be polymodal, that is, encode for multiple different stimuli, the stimuli that excite these primary afferents have in common the potential for producing tissue damage. They have therefore been defined as nociceptors. Primary afferent nociceptors are thinly myelinated or nonmyelinated and so fall into the Aδ- and C-fiber classes. Human psychophysical data support that when Aδ- and/or C-fiber function is disrupted by ischemia or pharmacologic agents, then cutaneous sensations associated with immediate (first) or briefly delayed (second) pains are similarly disrupted. Unfortunately (for the sake of easy logic), there are also many primary afferents that do not encode for tissue-damaging stimuli (e.g., “warm” receptors) but which are also of the Aδ- and C-fiber classes. Hence, it is a flawed logic that interprets all Aδ- and C-fiber-related input to second-order neurons as nociceptive. Existent literature constrains further definition of specific nociceptor neurochemical and localization characteristics except on an anecdotal (single unit) basis. With that caveat, there are basic patterns that appear common to most Aδ and C fibers, and generalizations related to these fiber groups have some validity as being representative of nociceptor localization and neurochemical content.
METHODS OF NEURONAL CHARACTERIZATION
To definitively describe the structure and function of CNS structures is a daunting task. As with nociceptors, standard histologic, ultrastructural, and neurochemical methods used to examine CNS structure have allowed for precise definition of axons, dendrites, and neurotransmitter content, but, unfortunately, they do not allow for the precise definition of function. Studies of neuronal function typically utilize electrophysiologic techniques to measure the real-time electrochemical activity of single neuronal units (e.g., action potentials) which may be evoked by multiple manipulations. These neurophysiologic measures utilize electrodes placed either extracellularly or intracellularly. When the former technique is utilized, correlative anatomic localization is possible but little more. Electrophysiologic techniques such as retrograde activation of axonal extensions can define some of the neuronal anatomy, but true morphology is only certain with the intracellular injection of a dye. Immunohistochemical characterization of intracellularly labeled neurons is methodologically feasible, and so it is possible to quantitatively define sensory elements. However, such studies are sufficiently tedious and subject to interpretive concerns related to sampling error and preparation effects (i.e., anesthesia) that, to date, these types of experiments have only been performed at a rudimentary level. A compromise microscopic analysis technique is that which uses induction of the c-fos gene or phosphorylation of extra cellular signal-related kinase (pERK) in response to neuronal activation to functionally identify neurons excited by a noxious stimulus. A proto-oncogene, c-fos, is activated after potentially tissue-damaging stimuli are applied to most tissues. The expressed product, Fos protein, is immunohistochemically identifiable within hours of stimulation. As a consequence, mapping of gene induction or Fos protein in the nucleus of activated neurons can be used to indirectly
functionally define these neurons as “nociceptive.”1 However, a systematic comparison study of Fos and pERK as markers of spinal nociceptive activation revealed greater specificity for activity in nociceptive pathways (vs. nonnociceptive activation) using pERK.2 Labeled neurons can then be colabeled with antibodies against neurotransmitters or important cell proteins or specific histologic stains to further characterize the neurons. Analgesic pharmacologic manipulations such as systemic morphine reduce both the total number of labeled neurons and the total Fos or pERK content of the spinal cord following a noxious stimulus. Even newer technologies have been able to identify a changed form of receptors following neuronal activation by noxious stimuli. For example, the internalization of neurokinin 1 (NK1) receptors following activation by substance P3 or the phosphorylation of glutamate receptor subtypes4 has been used as surrogates for neuronal excitation. Macroscopic examination of CNS activation sites using magnetic resonance imaging technologies have allowed confirmation of microscopic techniques and further demonstrated the functional complexity of spinal and supraspinal connections. At a microscopic level, “tracer” dyes which are taken up by the terminal endings of axons of neurons and transported back to the neuron somata allow for a histologic identification of axonal projections of spinal neurons that is dependent on the site of dye injections (e.g., spinothalamic neurons are retrogradely labeled to the spinal cord by injections in the thalamus). Such labeling techniques, when coupled together with functional techniques, have made it possible to construct a quantitative but nonspecific “global” neuroanatomic view of spinal cord nociceptive processing that appears to agree with anecdotal, definitive evidence generated by single-unit studies.
functionally define these neurons as “nociceptive.”1 However, a systematic comparison study of Fos and pERK as markers of spinal nociceptive activation revealed greater specificity for activity in nociceptive pathways (vs. nonnociceptive activation) using pERK.2 Labeled neurons can then be colabeled with antibodies against neurotransmitters or important cell proteins or specific histologic stains to further characterize the neurons. Analgesic pharmacologic manipulations such as systemic morphine reduce both the total number of labeled neurons and the total Fos or pERK content of the spinal cord following a noxious stimulus. Even newer technologies have been able to identify a changed form of receptors following neuronal activation by noxious stimuli. For example, the internalization of neurokinin 1 (NK1) receptors following activation by substance P3 or the phosphorylation of glutamate receptor subtypes4 has been used as surrogates for neuronal excitation. Macroscopic examination of CNS activation sites using magnetic resonance imaging technologies have allowed confirmation of microscopic techniques and further demonstrated the functional complexity of spinal and supraspinal connections. At a microscopic level, “tracer” dyes which are taken up by the terminal endings of axons of neurons and transported back to the neuron somata allow for a histologic identification of axonal projections of spinal neurons that is dependent on the site of dye injections (e.g., spinothalamic neurons are retrogradely labeled to the spinal cord by injections in the thalamus). Such labeling techniques, when coupled together with functional techniques, have made it possible to construct a quantitative but nonspecific “global” neuroanatomic view of spinal cord nociceptive processing that appears to agree with anecdotal, definitive evidence generated by single-unit studies.
DEFINING NOCICEPTIVE SECOND-ORDER NEURONS
Strict proponents of specificity theory state that all discussion related to pain should only involve CNS neurons excited exclusively by primary afferent nociceptors. Such specific second-order neurons are a small but an obviously important minority of the total sample of spinal neurons receiving input from primary afferent nociceptors. One can also argue that the presence of primary afferent neurons with specificity for pain-producing stimuli does not necessitate that the secondorder neurons responsible for pain sensation have a similar specificity. For purposes of the present discussion, the primary premise of the rest of this chapter is that such excitatory input is a necessary requirement of pain-related second-order neurons, but it is notable that most second-order neurons receiving such input receive other types of sensory input. Excitation of second-order neurons may come from primary afferent pathways or from segmental (interneurons), propriospinal (nonsegmental intraspinal), and supraspinal sources. Inhibition arises from the same CNS sources and can promote neuronal specificity by selectively reducing responsiveness to nonnociceptive inputs. It is for this reason that proponents of pattern theory argue that all second-order neurons receiving nociceptive inputs should be considered as candidates for inclusion in pain-processing pathways.
DEVELOPMENT OF SENSORY SYSTEMS
The embryologic development of the nervous system suggests reasons that differences can exist between peripheral and central phenomena because excitatory systems develop before inhibitory systems. The edges of the neural plate that come together to form the neural tube split off to become the migratory cells of the neural crest. These cells spread to form the sensory components of the peripheral nervous system. At a spinal cord level, substances from the ventrally located notochord induce the formation of motoneurons with axonal extensions extending to the periphery. The dorsal aspect of the spinal cord, lacking effects of the notochord, forms short connections (local connectivity) or develops axonal projections attracted to distant spinal cord and/or brainstem sites. Sensory structures that develop from the neural crest send axonal projections both to the periphery as well as into the dorsal aspect of the spinal cord and contain neurotransmitters that are predominantly excitatory. At birth, sensory systems have very little inhibitory connectivity. This changes during development until inhibitory connections become the predominant form of CNS communication.
In humans, the precise timing of both excitatory and inhibitory system maturation is not fully known, but based on experiments in nonhuman animals, these systems appear highly plastic with cell death processes as important as cell growth processes in relation to the final product.5 Specific transcriptional factor expression has been used to track neuronal subgroup development and has demonstrated a profound role for pathologic modification of nociceptive circuitry.6 The general phenomenon of use-dependent growth (or preservation) appears to hold in multiple sensory systems ranging from taste to vision with the nociceptive systems notwithstanding. Ruda and colleagues7 have demonstrated that injury during critical periods of development, such as the neonatal period, can have profound effects on the subsequent development of nociceptive systems. In humans, critical periods of neuronal outgrowth and myelination occur in childhood, during puberty, and following events that injure nervous system structures.
Targets of Primary Afferent Input
GROSS ANATOMY OF THE SPINAL CORD
The spinal cord is segregated into areas that, on gross examination, appear as white and grey matter and consist of predominantly myelinated nerve fiber tracts and cell bodies, respectively. Wrapped in protective pial, arachnoid, and dural meninges, the spinal cord is continually being penetrated by centrally directed axons of primary afferent neurons whose cell bodies reside within the neighboring dorsal root ganglia. These axons enter as the dorsal roots and may traverse several spinal segments rostrally or caudally in the dorsolaterally located Lissauer’s tract before entering the grey matter for synaptic contact. The spinal cord white matter is divided into multiple subdivisions with component “tracts” consisting of ascending or descending axonal fibers of various origins and destinations. There is significant overlap of these tracts, such that any lesion of white matter is likely to interrupt fibers of passage with multiple origins and multiple sites of termination. The white matter gets larger as one ascends the spinal cord from sacral to cervical levels as additional ascending fibers to the brain add to the white matter and progressive numbers of descending fibers to spinal targets drop out to form synaptic connection. Grey matter is largest at the cervical and lumbar enlargements due to association with sensation and motor control of the limbs. The most notable divisions of the white matter that are important to pain sensation are the dorsal columns, the dorsolateral fasciculus, and the ventrolateral (anterolateral) fasciculus and their associated subdivision into tracts (Fig. 4.1).
SPINAL LAMINAE
The morphology of neurons in the grey matter of the spinal cord differs depending on location. Using Rexed’s cytoarchitecture-based classification system, there are at least 10 different layers or laminae of neurons—of which the first six (I to VI) are termed the dorsal horn of the spinal cord (Fig. 4.2). These laminae, plus the area around the central canal (lamina X), receive a bulk of primary afferent inputs. Spinal dorsal horn neurons receiving excitatory inputs from nociceptive afferents have been demonstrated to be present throughout the dorsal horn but with particular localization to laminae I, II,
V, VI, and X. One must remember that laminar assignment is based on the central location of the neuronal soma. However, dendritic extensions of these neurons may extend throughout numerous laminae such that the immunohistochemical demonstration of primary afferent neuron terminations in specific laminae does not limit connectivity to just neurons of those laminae. Furthermore, although both myelinated and unmyelinated nociceptive afferent fibers project predominately to the laminae described earlier, there exist projections in those laminae from afferent fibers that transmit innocuous sensory information. Mapping of individual C-fiber primary afferents encoding for cutaneous nociception has demonstrated sites of connectivity that are highly localized into tight “baskets” typically located in superficial laminae of a single spinal segment. In contrast, single primary C-fiber afferents from deep, visceral structures have been demonstrated to travel via Lissauer’s tract to multiple spinal segments and multiple laminae (I, V, X, and even contralateral sites) (Fig. 4.3).8 Fine muscle afferents and articular afferents have sites of termination similar to those of visceral afferents. By using intracellular recording and labeling techniques, it has been possible to determine that nociceptive afferents connect with second-order neurons that have many different morphologies—some of which correlate with electrophysiologic characteristics (see Morris et al.9 and Grudt and Perl10). The morphology and neurotransmitter content of each lamina will be briefly discussed.
V, VI, and X. One must remember that laminar assignment is based on the central location of the neuronal soma. However, dendritic extensions of these neurons may extend throughout numerous laminae such that the immunohistochemical demonstration of primary afferent neuron terminations in specific laminae does not limit connectivity to just neurons of those laminae. Furthermore, although both myelinated and unmyelinated nociceptive afferent fibers project predominately to the laminae described earlier, there exist projections in those laminae from afferent fibers that transmit innocuous sensory information. Mapping of individual C-fiber primary afferents encoding for cutaneous nociception has demonstrated sites of connectivity that are highly localized into tight “baskets” typically located in superficial laminae of a single spinal segment. In contrast, single primary C-fiber afferents from deep, visceral structures have been demonstrated to travel via Lissauer’s tract to multiple spinal segments and multiple laminae (I, V, X, and even contralateral sites) (Fig. 4.3).8 Fine muscle afferents and articular afferents have sites of termination similar to those of visceral afferents. By using intracellular recording and labeling techniques, it has been possible to determine that nociceptive afferents connect with second-order neurons that have many different morphologies—some of which correlate with electrophysiologic characteristics (see Morris et al.9 and Grudt and Perl10). The morphology and neurotransmitter content of each lamina will be briefly discussed.
Lamina I: Termed the marginal zone as it forms the outermost layer of the dorsal horn. This single lamina contains a heterogeneous population of neurons with morphologic studies identifying neurons with pyramidal, fusiform, and multipolar shapes, some with smooth and some with spiny dendrites. Morphology and function are correlated in at least a subset of these neurons. Lamina I and the adjoining lamina II are the predominant location of excitatory neuropeptide input from primary afferent neurons with heavy immunohistochemical labeling for substance P and calcitonin gene-related peptide (CGRP). Axonal projections of a subset of lamina I neurons extend to supraspinal structures such as the medulla, midbrain, and/or thalamus. Fos and pERK induction in response to noxious stimuli have consistently been reported to occur in lamina I with double-labeling noted in association with antibodies to preproenkephalin, dynorphin, glutamate, N-methyl-D-aspartate (NMDA) receptors, γ-aminobutyric acid (GABA), glycine, GABA-B receptors, NK1 receptors, calbindin, glucocorticoid receptors, and estrogen receptor α.1
Lamina II: Called the substantia gelatinosa due to its gross jellylike appearance in fresh cut tissue. The neurons of this lamina are generally small with five distinct morphologies, two of which are viewed as important to the local processing of nociceptive information: stalked and islet cells (using the terminology of Gobel11). Stalked cells have soma at the outer edge of lamina II with centrally arborizing dendrites and axons that synapse with lamina I projection neurons. Islet cells have fusiform cell bodies with extensive dendritic and axonal arborizations containing inhibitory neurotransmitters such as GABA or enkephalin.12,13 Axodendritic, dendrodendritic, and axoaxonic synapses are manifest throughout lamina II on ultrastructural analysis demonstrating a profound potential for neuronal interaction and signal processing. Primary afferent input from both nociceptive and nonnociceptive neurons has been noted; particularly, input from a population of nonpeptidergic, nociceptive primary afferents, is received in a narrow band within lamina II. Descending axonal connections are also present with evidence of serotonergic and noradrenergic inputs to lamina II neurons. A distinction is frequently made between lamina II-outer (IIo) and lamina II-inner (IIi) with IIo commonly combined with lamina I in discussions of the superficial dorsal horn.
Lamina III and lamina IV: Known as the nucleus proprius as both receive highly myelinated low-threshold primary afferent neuronal inputs that include proprioceptors. Whereas lamina III consists mainly of small neurons with morphologies similar to lamina II, lamina IV has a subpopulation of large cells with extensive dendritic extensions that reach superficially. Laminae III and IV also have neurons with axonal projections extending up ascending pathways including the spinothalamic tract and the dorsal columns.
Lamina V: Neurons receive input from Aδ- and C-fiber nociceptors as well as myelinated afferents carrying low-threshold information. Cell bodies are frequently moderately sized pyramidal cells and may have dendritic extensions through the entire dorsal horn reaching to lamina I and II sites of synaptic contact as well as laterally. Neurons of this lamina have been demonstrated to receive input from all somatic, muscle, and visceral afferent types. Axonal projections of lamina V neurons to supraspinal sites are common.
Lamina VI: Neurons are small neurons present in the cervical and lumbar enlargements but largely missing in the rest of the spinal cord. Low-threshold muscle afferents and both low- and high-threshold cutaneous afferents reach to this lamina.
Laminae VII to IX: Represent neurons of the ventral horn.
Lamina X: Neurons are arranged around the central canal of the spinal cord. They receive bilateral inputs of unmyelinated, poorly myelinated, and highly myelinated afferents. Cell bodies are of moderate size with local dendritic arborizations. Some neurons in the dorsal aspects of lamina X have axonal projections extending up the dorsal columns to reach medullary targets. Peptidergic inputs are extensive and immunohistochemical localization of noradrenergic and serotonergic inputs
have been identified. Inhibitory neurons with immunohistochemical identification of glycinergic and GABAergic enzymes are locally present.
have been identified. Inhibitory neurons with immunohistochemical identification of glycinergic and GABAergic enzymes are locally present.
FUNCTIONAL CHARACTERIZATION OF NOCICEPTIVE NEURONS
In addition to morphologic heterogeneity, the multiple laminae of the spinal cord also have functional heterogeneity. Second-order neurons have been characterized electrophysiologically according to their responsiveness to cutaneous and deep tissue stimuli. Most commonly, a distinction is made between excitatory responses that are produced by noxious (potentially tissue-damaging) stimuli such as high-intensity mechanical or thermal stimuli and those produced by innocuous stimuli such as hair movement or vibration. Using these two criteria, the simplest nomenclature defines neurons as Class 1 if excited only by innocuous stimuli, Class 2 if excited by both innocuous and noxious stimuli, and Class 3 if excited only by noxious stimuli. Similar nomenclatures, but with additional subtle meanings implied by their original descriptions, include the use of terms such as low threshold for Class 1 neurons, wide-dynamic-range14 or convergent15 for Class 2 neurons, and high threshold or nociceptive-specific for Class 3 neurons. A fourth nonresponder group also must be factored in for neurons that fail to be excited by any of the employed stimuli. Despite the “clean” nature of this categorization, there unfortunately appears to be a spectrum of responses to all afferent input modalities with varying overlap that is somewhat dependent on the precise stimuli and definitions employed. The definition of neurons according to excitatory stimuli also appears to be preparation-dependent, as extracellular dorsal horn recordings of spinal neurons in cats have demonstrated that the classification of an individual neuron may change with the administration of anesthesia.16
The number of neuronal subgroups in any classification system is a function of the number of criteria employed for that classification. Despite this, it has been suggested that the use of inhibitory inputs as part of a classification criterion might actually simplify overall schema. Some neurons are inhibited following primary afferent activation. Inhibitory neurotransmitters are uncommon, if not absent, in primary afferents and so inhibition has generally been interpreted as due to a “modulatory” influence.
One such modulatory inhibitory influence used in classification is known as diffuse noxious inhibitory controls (DNIC) which is proposed to be an endogenous inhibitory system activated by a nonsegmental noxious stimulus. DNIC produces inhibition of ongoing or evoked dorsal horn neuronal activity, and according to its original description,17,18 DNIC is specific for Class 2 (wide dynamic range [WDR], convergent) neurons and has no effect on Class 3 (nociceptive-specific [NS]) neurons. Subsequent studies of DNIC would support the general statement that DNIC effects are highly selective for Class 2 neurons with lesser effect on Class 3 neurons. Nomenclatures using a combination of responses to excitatory and inhibitory inputs have not been universally accepted.
One such modulatory inhibitory influence used in classification is known as diffuse noxious inhibitory controls (DNIC) which is proposed to be an endogenous inhibitory system activated by a nonsegmental noxious stimulus. DNIC produces inhibition of ongoing or evoked dorsal horn neuronal activity, and according to its original description,17,18 DNIC is specific for Class 2 (wide dynamic range [WDR], convergent) neurons and has no effect on Class 3 (nociceptive-specific [NS]) neurons. Subsequent studies of DNIC would support the general statement that DNIC effects are highly selective for Class 2 neurons with lesser effect on Class 3 neurons. Nomenclatures using a combination of responses to excitatory and inhibitory inputs have not been universally accepted.
CLASSIFICATION ACCORDING TO SITE OF PROJECTION
Second-order nociceptive spinal dorsal horn neurons frequently have axonal extensions projecting to rostral (and caudal) sites of termination. These sites include other segments of the spinal cord and supraspinal structures such as the thalamus, the hypothalamus, the midbrain, the pons, and the medulla. These axons travel predominantly within the white matter of the spinal cord in two main sites: the ventrolateral quadrants and the dorsal midline. Ascending fiber tracts in the dorsolateral funiculus have also been described. Decussation of fibers to the contralateral ventrolateral white matter occurs for axons projecting to the thalamus and most other brainstem sites, although many axons with sites of termination in “reticular” structures remain in the ipsilateral ventrolateral white matter. Dorsal column pathways have sites of termination in the gracile or cuneatus nucleus of the medulla. Intraspinal pathways that may stay within the grey matter have also been demonstrated as well as extensive collateralization of axons with multiple sites of termination at supraspinal and intraspinal sites.
Targets of Axonal Projections
INTRASPINAL PATHWAYS
Multiple interconnections occur between spinal neurons. On a segmental level, this is referred to as interneuron connectivity. When connections are more distant, the pathways of connection are termed propriospinal based on the initial demonstration of a coordinating connectivity between the cervical and lumbar enlargements of quadrupeds that allowed for coordinated motion. Intraspinal connectivity has also been demonstrated in the case of neurons receiving afferent input from pelvic structures with a dual innervation through thoracolumbar sympathetic and sacral pelvic nerves. These intraspinal connections appear to coordinate autonomic functions related to the pelvic organs.19,20 A precise white matter localization of the axonal extensions of propriospinal nociceptive neurons has not been performed, but they are presumed to follow the paths of other propriospinal neurons which include dorsally located white matter paths and some within grey matter extensions. Collateral intraspinal extensions of ascending axons located within the ventrolateral white matter have also been demonstrated.
A separate system of intraspinal connections, the multisynaptic ascending system, may have particular relevance to chronic pain. In concepts championed by Noordenbos21 but first proposed by Goldschneider, long chains or “webs” of neuronal connections extend through the length of the spinal cord and carry nociceptive information in a slow but progressive fashion to the brain by short “hops.” Support for this concept is given by animal experiments, in which opposing hemisections performed at differing spinal levels fail to block nociceptive behaviors, but total transections at a single level are effective.22 Traditionally described as an ascending pathway, bidirectionality of signaling is possible with a potential for the generation of “reverberatory” circuits.
SPINOTHALAMIC TRACT
Ventrolateral (Anterolateral) Axonal Pathways
The most studied spinal projection pathway is the spinothalamic tract which is located within the ventrolateral white matter of the spinal cord. Both ipsilateral and contralateral localization of axon pathways have been demonstrated en route to supraspinal sites, but the predominant path for axonal projections traveling to the ventrobasal thalamus resides on the contralateral side. Experiments utilizing lesions of the ventrolateral spinal white matter have demonstrated reduction or abolition of ventrobasal thalamic neuronal responses to most somatic noxious stimuli. Consistent with this observation, surgical or traumatic interruption of ventrolateral fiber pathways results in the lack of sensation to noxious cutaneous stimuli (i.e., pinprick) applied to the contralateral side of the body at spinal segments below the level of the lesion. The second-order neurons which project to the thalamus have been identified in rodent, feline, and primate models using neuroanatomical (retrograde dye labeling or chromatolytic responses to axonal section) and electrophysiologic (antidromic activation) methods. Neurophysiologic experiments have not always proven that the neurons of study had axonal projections that actually reach the thalamus but have always demonstrated that neurons of interest have axons present within the ventrolateral spinal white matter and so the term spinothalamic tract (STT) neurons is generally employed to describe the neurons rather than the more specific term spinothalamic.
Neospinothalamic versus Paleospinothalamic
There exist two different components of the spinothalamic tract—the neospinothalamic tract (nSTT) and the paleospinothalamic tract (pSTT), the former of which forms a direct, dedicated relay to the ventrobasal group of the thalamus and the latter of which has many neurons with one or more axonal bifurcations that form dichotomizing fibers ending in synaptic contact with medullary, pontine, midbrain, and medial thalamic structures. Ascending information transmitted through the pSTT produces activation of numerous limbic structures and has therefore been viewed as important to affective and motivational aspects of pain, whereas the nSTT, through relays in the ventrobasal and posterior thalamus, activates the somatosensory cortex and so has been viewed as important to localization and intensity coding of pain-related sensations. Axonal collateral branchings of pSTT neurons have been identified that correspond to multiple areas of limbic and autonomic activation which include medullary, pontine, mesencephalic, hypothalamic, and medial thalamic targets.
Laminar Distribution of Spinothalamic Tract Neurons
The cells of origin of the STT reside within all laminae of the spinal cord except motoneuronal layers and therefore can have multiple different morphologies. At lumbar levels in primates, the greatest number of nSTT neurons not only reside in laminae I and V but also are highly represented in laminae IV, VI, IX, and X with additional representation in III, VII, and VIII. In contrast, the neurons of the pSTT have soma in deeper laminae (VI to VIII) with a lesser representation in I, IV, and V. The axons of STT neurons typically decussate to the contralateral side via the dorsal commissure within one to two spinal segments of the neuronal soma, but at sacral and upper cervical levels, a significant number (up to 26%) of axons of STT neurons may remain ipsilateral.23 A general somatotopic organization of the ascending fibers within the STT has been noted with an inner-to-outer progression of layers of fibers to the white matter from cervical to sacral levels (Fig. 4.4). A similar somatotopy is noted at medullary levels. The STT splits into two parts through the rostral medulla and pons to merge again at mesencephalic regions where an anterior-to-posterior somatotopic distribution
is noted. Differences in conduction velocity of subsets of STT neurons has been identified (lamina I STT neurons have slower conduction velocities than lamina V STT neurons) suggesting differences in axonal diameter and myelination processes. That, in turn, suggests a potential for temporally different delivery of sensory information to brain structures and differential susceptibility to pathologic processes.
is noted. Differences in conduction velocity of subsets of STT neurons has been identified (lamina I STT neurons have slower conduction velocities than lamina V STT neurons) suggesting differences in axonal diameter and myelination processes. That, in turn, suggests a potential for temporally different delivery of sensory information to brain structures and differential susceptibility to pathologic processes.
Functional Characterization of Spinothalamic Tract Neurons
Quantitative electrophysiologic characterization of STT neurons has demonstrated a predominance for neurons processing nociceptive information. However, approximately 20% of STT neurons encode exclusively for nonnoxious light touch sensations,13 and a small subset encodes for proprioceptive information. Multiple subsets of nociceptive neurons have been identified that have selective laminar localization. NS STT neurons, with slow adapting responses to noxious pinch, heat, or chemical stimulation, are commonly located in lamina I but are also present in deeper laminae. Noxious cold has been used as an additional characterizing stimulus24 and allowed for the identification of additional subsets of lamina I NS STT neurons. WDR STT neurons, which demonstrate excitatory responses to multimodal sensory inputs (including both noxious and nonnoxious stimuli), are found extensively in lamina V but can also be found in all other laminae. Convergence of visceral, myofascial, articular, and cutaneous inputs is the rule rather than the exception when examining WDR STT neurons,25 but similar convergence has been noted in NS STT neurons. Overall, with the possible exception of noxious cold inputs, the presence or absence of a particular group of sensory inputs has been of limited value in identifying lamina-specific, morphologic, functional, or projection-related neuronal subsets. However, there are important generalities that are apparent in quantitative analyses of neuronal subsets26: Lamina I STT neurons tend to be NS neurons, lamina V (and other deep laminae) STT neurons tend to be WDR neurons, STT neurons with projections to the medial thalamus (pSTT) are more likely to be NS rather than WDR neurons and frequently have large receptive fields, STT neurons with projections to the lateral thalamus (nSTT) are more likely to be WDR rather than NS neurons and often have smaller receptive fields, and STT neurons with projections to both medial and lateral thalamus appear similar to neurons with projections only to the lateral thalamus.
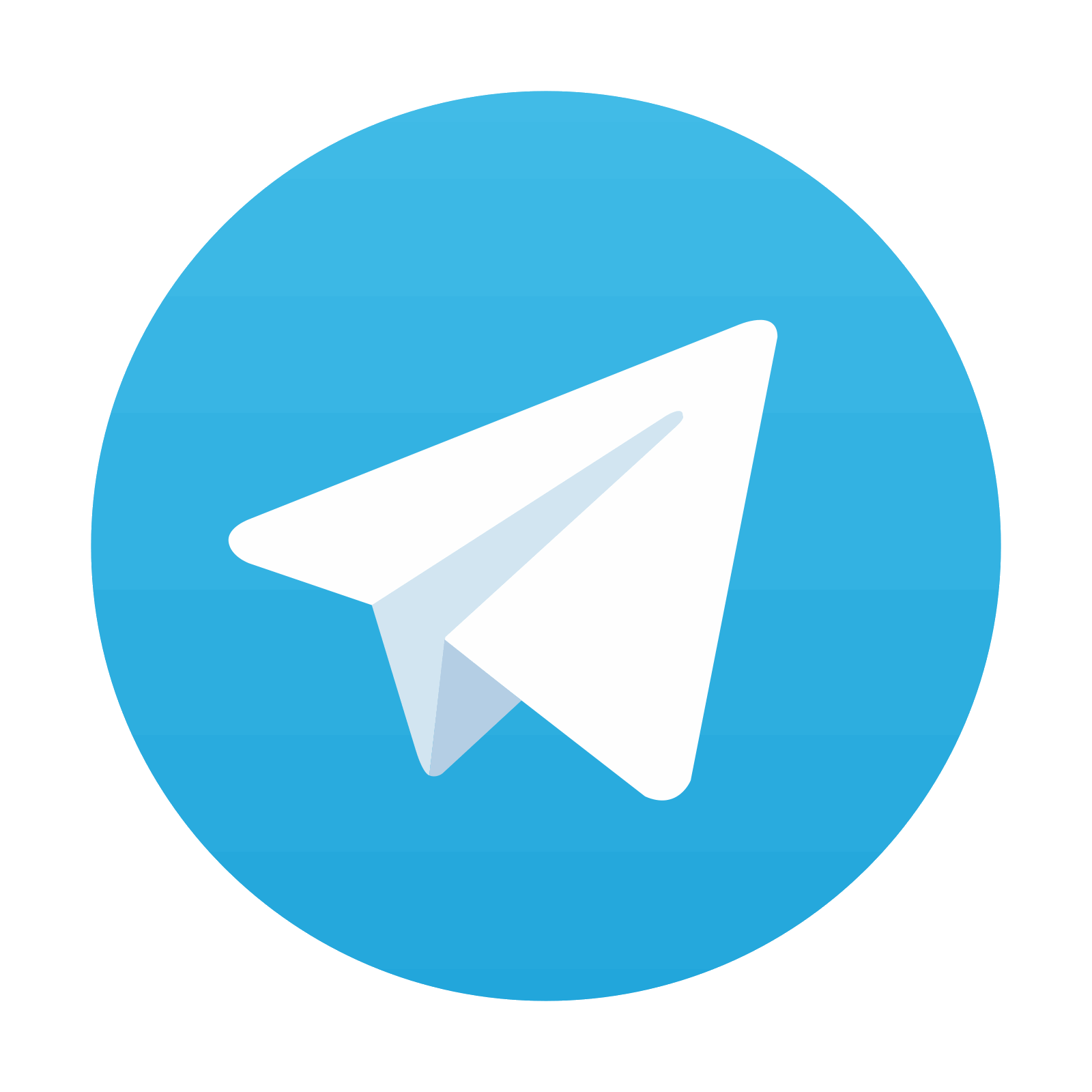
Stay updated, free articles. Join our Telegram channel
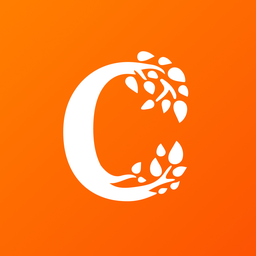
Full access? Get Clinical Tree
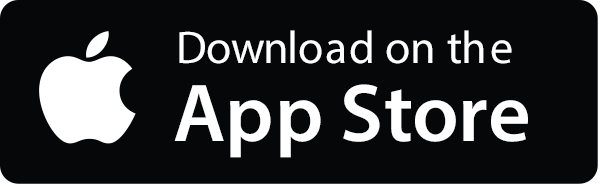
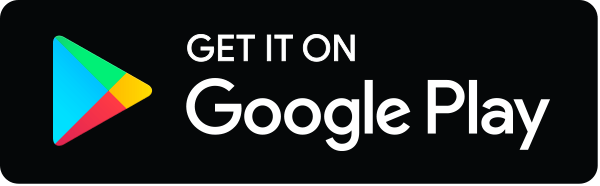
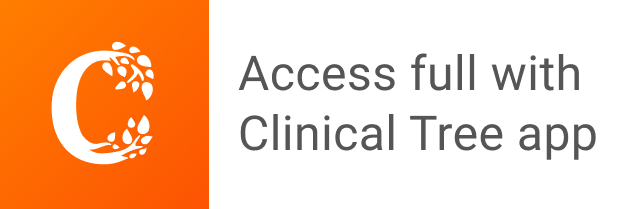