© Springer International Publishing Switzerland 2017
Davide Chiumello (ed.)Acute Respiratory Distress Syndrome10.1007/978-3-319-41852-0_1717. Stem Cells and Their Immunomodulatory Potential for the Treatment of ARDS
(1)
The Keenan Centre for Biomedical Research, The Li Ka Shing Knowledge Institute and the Interdepartmental Division of Critical Care, University of Toronto, Toronto, ON, Canada
(2)
St. Michael’s Hospital, 30 Bond Street, Bond Wing, Room 4-008, Toronto, ON, Canada
17.1 Introduction
Despite significant advances in supportive ICU care, acute respiratory distress syndrome (ARDS) remains a leading cause of death and disability worldwide [1–4]. Many potential therapies have been found ineffective, including corticosteroids and immunomodulation agents. Reasons behind the failed trials in ARDS have been discussed [5–8]. From the preclinical research perspective, the largely disappointing results from clinical trials can be explained in part by our incomplete understanding of the molecular responses to injury and the mechanisms that promote repair [9–13]. Dysregulated inflammation, inappropriate accumulation and activity of leukocytes, and disruption of the alveolar-capillary barrier remain central to the pathophysiology of ARDS. A therapeutic approach that can successfully target injurious inflammation and restore alveolar-capillary barrier function, while maintaining host defense and augmenting tissue repair, would be an ideal strategy for the treatment of ARDS. Rapid and exciting progresses in the field of cell immunotherapy have recently emerged as a disruptive strategy that can modulate the immune system and overcome the immunological derailment characterizing the pathophysiology of ARDS. This chapter focuses on summarizing current knowledge on the immunomodulatory actions of mesenchymal stromal cell (MSC) as novel immunotherapeutics for the treatment of ARDS and the translation of new knowledge to clinical trials.
17.2 Mesenchymal Stem/Stromal Cell (MSCs)
Although mesenchymal stem/stromal cells can be derived from a variety of different tissue sources, bone marrow-derived mesenchymal stem/stromal cells (BM-MSCs) have been the most extensively studied in the field of acute lung injury (ALI, the pro-clinical correlate of human ARDS) and will be the main focus of this review. Some controversy remains regarding how to name these pluripotent cells [14], stem vs. stromal, and minimal criteria to define them have been established [15]. First, MSC should be adherent to plastic in standard culture conditions. Second, they should express CD105, CD73, and CD90 and lack expression of CD45, CD34, CD14 or CD11b, CD79alpha or CD19, and HLA-DR surface molecules. Finally, they should differentiate to osteoblasts, adipocytes, and chondroblasts in vitro [15]. MSCs possess various favorable biological characteristics underscoring their clinical utility, including their convenient isolation, their rapid expansion in culture, and high clonogenicity while preserving their genetic stability and minimal immunogenicity making them a viable option for allogenic transplantation. The latter has recently come into question as MSCs may be immune evasive rather than immune privileged [16].
MSCs also preferentially home to the wounded or damaged tissue sites [17]. Traffic of MSCs to areas of tissue injury appears to be driven by different interactions between chemokines released from the injured tissue and chemokine receptors expressed on MSCs. For example, stromal cell-derived factor 1/CXCR4 pathway can mediate traffic of MSCs to ischemic or hypoxic tissues [18–20], while CD44 expressed on MSCs may interact with hyaluronic acid where interstitial matrices are exposed following injury [21–23]. Following intravenous administration, MSCs become entrapped in the capillary beds; this occurs primarily in the lung and liver [24]. In the context of ARDS, the relative biological significance of MSC entrapment is unclear since MSCs from bone marrow, adipose, and other tissues stay in the lungs for only about 24–48 h prior to being cleared [25, 26]. The overall opinion is that these cells may exert their beneficial effect through mechanisms that may not require cell-cell contact, and although cell engraftment with differentiation, transdifferentiation, or cell fusion may contribute to reconstitution of normal organ function, these are of uncertain therapeutic significance [27–29]. In contrast to the engraftment hypothesis, several alternative mechanistic explanations have been proposed for how these cells exert their therapeutic effects including:
- (i)
Their potential for enhancing tissue-endogenous stem/progenitor cell activity
- (ii)
Regulation of genes that modulate the response to injury and repair
- (iii)
Transfer of cellular and genomic contents such as mitochondria and microRNAs
- (iv)
Secretion of paracrine factors that target key aspects of the pathophysiology of injury and repair in ARDS
17.3 Performance of MSCs in Preclinical Models of ALI and Translation to Clinical Trials
The growing interest in the therapeutic potential of these cells for the treatment of ARDS rests solidly on their robust and reproducible performance in preclinical models of organ injury including lung bleomycin [30], intratracheal [31–33] or intraperitoneal [34, 35] endotoxin, cecal ligation and puncture [36], pseudomonal abdominal sepsis [37], and E. coli pneumonia [38]. In most, if not all models, stem cell administration reduces the odds of death [39, 40]. This is true for overall mortality, as well as mortality at predefined time intervals [39]. MSC administration reduces death even when the initiation of MSC therapy is delayed to 6 h post induction of ALI (or sepsis). In terms of secondary outcomes, MSCs may exert their beneficial effect by reducing inflammation while enhancing bacterial clearance [41], augmenting lung repair [42], and restoring alveolar-capillary barrier function [43, 44]. The dramatic results in preclinical models of ALI has propelled rapid translation to the clinic, with a recent phase I trial completed [45, 46], a phase 2 trial in progress (NCT02097641), and another phase 1/2 randomized, double-blind, placebo-controlled trial (NCT01902082) on the way. The latter targets not only safety but also efficacy outcomes, using allogeneic adipose-derived MSCs. In both studies, cells will be administered intravenously, inclusion criteria are similar, and MSC therapeutic doses vary from 1 to 10 × 106 cells/kg. In parallel, the Canadian Critical Care Trials Group has also initiated the first human trial using stem cell as immunotherapy for the treatment of sepsis (Cellular Immunotherapy for Septic Shock, CISS Trial, NCT02421484).
As we transition from preclinical to clinical studies, many questions remain about how we will translate knowledge acquired on the bench or in animal models to the clinic. We will highlight some of these questions throughout the review, but the issue of potency deserves brief attention. The International Society for Cellular Therapy (ISCT) released in 2015 their perspective on immune functional assays for MSC potency [47]. The proposal springs from a broad consensus in the community that, despite different tissue sourcing and culture expansion protocols, human MSCs likely share fundamental mechanisms of action. Identification of functional markers of potency and harmonization of standard operating procedures to measure biological properties of stem cells intended for therapy is a primary goal of future studies. The preferred analytic methods proposed by the ISCT focus on (a) gene, (b) protein (secretome), and (c) cell marker expression that reflect current knowledge in the field. While safety and tolerability of human MSCs remain the main primary study end points, future studies will aim to address secondary efficacy end points by assessing respiratory, systemic, and biological markers of function. Here, we will briefly discuss the state of knowledge of the immunoregulatory properties of these cells and their potential for future therapeutics in ARDS.
17.3.1 Enhancing Tissue-Endogenous Stem/Progenitor Cell Activity
The adult lung is a tissue with relatively slow cellular turnover. The turnover of airway epithelial cells is less than 1% per day during steady-state conditions, in contrast to the regenerative capacity of a continuously renewing tissue, such as bone marrow, which has the ability to generate approximately 109 hematopoietic cells per day [48]. Repair in the lung in turn depends on the regenerative capacity of over 40 different types of cells for tissue regeneration or replacement. Following severe injury, self-renewing potential of stromal and other progenitor cells of the lung increases significantly [49]. Initially, it was thought that MSCs participated directly in the repair process by incorporating into regenerated tissues and replacing cells [50, 51]. It has been difficult to resolve the fate of grafted MSCs because of the short half-life of engrafted MSCs, lack of sensitive means for isolation and detection, and low targeting efficiency [17]. Cumulative evidence suggests exogenous MSCs are unlikely to replace/regenerate lung tissue. Differentiation and/or transdifferentiation of specific progenitor cells along the trachea, bronchioles, or alveoli depends on distinct progenitor populations (and specific signals) that contribute to lung maintenance and repair [52]. Recent evidence suggests exogenously administered MSCs may enhance resident stem cell “niches,” that in turn repopulate the lung and repair damaged tissue.
The ability of MSCs to stimulate endogenous progenitor cells was first demonstrated in experiments where human MSCs were injected directly into the hippocampus of immune-deficient rats [53] where they enhanced the proliferation of the endogenous neural stem cells increasing their migration and differentiation into neural cells. Similar findings were documented in a model of intracerebral hemorrhage, where the number of endogenous progenitor cells was increased in MSC-treated animals compared to controls [54]. More recently, in a model of excisional wound repair that mimics wound reepithelialization and granulation tissue formation, labeled human MSCs and species-specific markers demonstrated that exogenous MSCs initiate the formation of a niche in the injured environment and promote recruitment of endogenous stem/progenitor cells to the site of injury [55]. Critical signals such as Wnt, vascular endothelial growth factor (VEGF), and platelet-derived growth factor receptor-α (PDGFRA) within these “niches” of repair suggest that the role of MSCs is largely limited to signaling that initiates the recruitment and direction of endogenous (progenitor) cells to sites of injury to promote repair [56].
Although it is now evident that the lung harbors a diverse population of progenitor cells [57, 58], direct evidence demonstrating the role of exogenous stem cells in enhancing the recruitment and repair activity of endogenous progenitors after lung injury is scant. Gotts et al. recently reviewed the various lines of evidence in support for an endogenous cell-based pathway for recovery in the lung [44], highlighting the diversity of lung epithelial/endothelial progenitor cell populations and their regulation [44]. In a murine model of cigarette smoke-induced COPD, MSCs transplantation can relieve lung injury by promoting proliferation of endogenous lung stem cells [59] although there is immense controversy about how to demonstrate the presence and activity of true lung progenitors [44, 60]. Bronchoalveolar stem cells increase after MSC treatment in a mouse model of bronchopulmonary dysplasia [61, 62]. A recent report suggests that MSCs may also promote repair through activation of endogenous distal lung airway progenitor cell populations in mouse models [61]. Further indirect evidence in support for exogenous stem cells as coordinators of an endogenous progenitor cell response comes from pneumonectomy experiments that demonstrate VEGF signaling in lung endothelium promotes matrix metalloproteinase 14 expression, which in turn releases EGF receptor ligands that drive epithelial progenitor proliferation and alveologenesis. Moreover, there are MSCs that reside in the lung. Resident lung MSCs niches can be found along the proximal-distal axis of the airway tree. Lung MSCs secrete FGF-10, a critical factor necessary for directing lung differentiation [63]. How these resident MSCs respond to exogenous MSC administration and their contribution to lung repair remains to be elucidated.
17.3.2 Secretion of Paracrine Factors
Diffusion of autocrine and paracrine signaling molecules enables cells to communicate in the absence of physical contact. MSCs possess strong immunoregulatory properties, affecting both innate and adaptive immune systems [14]. Specific factors secreted by stem cells have various functional properties such as being antiapoptotic, immunomodulatory, and angiogenic, playing a role in cell mobilization, evoking responses from resident cells in the extracellular environment, and facilitating collateral remodeling [64]. The activity of various immune cells, including T cells, B cells, neutrophils, NK cells, and dendritic cells, is modulated in the presence of MSCs. For example, MSCs suppress T-cell activation and proliferation, inhibit monocyte differentiation, and block proliferation of B cells [65–68]. Interestingly, the immunosuppressive ability of MSCs may be dose dependent, where a low dose (below a threshold which is yet to be determined) elicits pronounced lymphocyte expansion [69], while a sufficient MSC “dose” suppresses T-lymphocyte proliferation, resulting in anergy of activated T lymphocytes [68, 70]. MSCs also inhibit B-lymphocyte proliferation in vitro by arresting the G0/G1 phase of the cell cycle rather than inducing apoptosis [67, 71], thus reducing antibody production [72]. Of note, in the absence of an inflammatory stimulus, naıve MSCs are unable to influence B-lymphocyte proliferation [73] underscoring the importance of the “inflammatory milieu” in guiding MSC responses: a phenomenon which is actively being exploited to engineer MSCs with specific biotherapeutic properties.
The immunosuppressive properties of MSCs are thought to depend on secretion of various soluble factors, including cytokines, indoleamine 2,3- dioxygenase (IDO), nitric oxide (NO), prostaglandin E2 (PGE2), and tumor necrosis factor-α-induced protein 6 (TSG-6) [52]. Although most studies rely on in vitro data to define specific mechanisms of action, in vivo, the interaction between endogenous and exogenous MSCs may lead to a complex interplay between specific factors: (i) multiple paracrine factors are involved in MSC-mediated modulation of the immune response, (ii) various factors share redundant functions, and (iii) many factors affect multiple processes (perhaps even simultaneously). For example, IL-1 receptor antagonist mediates both the anti-inflammatory and anti-fibrotic effects of MSCs after lung injury [74]. A further layer of complexity is provided by the existence of resident MSCs [75, 76]. The ability of tissue-resident MSCs to physiologically modulate the immune system (or be modulated by exogenous MSC administration) is unknown [52].
In specific reference to models of ALI, MSC regulation of angiopoietin1 (ANGPT1) has been identified as a critical event to promote survival of endothelial cells, protect against vascular inflammation and leakage, and reduce loss of barrier function [32]. MSCs engineered to produce ANGPT1 significantly reduced ALI in preclinical models indicating that the beneficial effects of MSCs can be further enhanced by gene therapy approaches [33]. Another mediator that has gained much attention in lung research is keratinocyte growth factor (KGF). In an ex vivo human lung injury model, KGF was shown to be essential for the beneficial effect of human MSCs on alveolar epithelial fluid transport [77]. In addition, hepatocyte growth factor (HGF) has been implicated in ameliorating lung injury in models of both emphysema and pulmonary fibrosis [77–79]. In vitro data suggests HGF acts on endothelial cells, restoring integrity and permeability [80]. Recently, it has been suggested that the therapeutic effects of MSCs can be reproduced, at least in part, by components of MSC secretome including KGF [77, 81] and ANGPT1 [32]. An intriguing property of MSCs is their ability to enhance bacterial phagocytosis and killing [36, 82, 83]. Bonfield et al. reported that systemic administration of MSCs significantly reduced weight loss, chronic infection, circulating neutrophils relative to macrophages, and lung pathologies in a murine cystic fibrosis infection and inflammation model [84]. In our own work, we demonstrated that treatment with MSCs significantly enhances gene expression pathways related to antigen presentation and bacterial killing [36, 85]. How MSCs enhance antibacterial clearance is an active area of research. Stimulated MSCs, in vitro and in vivo, synthesize antimicrobial peptide LL-37 and β-defensins. Increased β-defensin 2 levels and antibacterial effects of MSCs are abolished by specific antagonist or by siRNA-mediated knockdown of TLR4, but not TLR2, and restored by β-defensin 2 supplementation [86]. This discovery suggests that MSCs are a potential therapeutic agent for acute and systemic infections; this will be tested in further trials looking at the role of stem cell therapy for the treatment of sepsis.
Moreover, MSCs have been implicated in “educating” professional phagocytes such as macrophages. Recent studies have suggested an emerging role for macrophages in the reduction of inflammation and promotion of tissue repair [87, 88]. Coculture or stimulation of immune cells with MSCs alters the expression of a number of pattern recognition receptors, which appear to play a role in their immunoregulatory actions [83, 89]. Human MSCs cocultured with macrophages induce macrophage M1/M2 phenotype transformation. The M2 macrophages have high phagocytosis capacity [90], increased migratory and proliferative capacity to produce extracellular matrix (ECM) components, angiogenic and chemotactic factors, increased levels of IL-10, decreased TNF-α and IL-12, and low co-stimulatory molecule CD86 and HLA class II [91]. In addition to the pathogen defense, M2 macrophages clear apoptotic cells, can mitigate inflammatory response, and promote wound healing. Also, M2 macrophages have complex roles outside the context of inflammation, such as organ morphogenesis, tissue turnover, and endocrine signaling. This effect of MSC is at least partially mediated by soluble mechanisms and PGE-2 has been indicated to be one of the factors involved [90, 91]. The current classification of macrophage immune activation is challenging because two very distinct aspects are considered: the in vitro effects of selected immune-related ligands on the phenotype of macrophages and the in vivo. The biological relevance of MSCs in the reciprocal regulation of M1/M2 macrophages is evidenced in studies where after injection of MSCs into myocardium and adjacent macrophages adopted an M2 phenotype characterized by strong expression of arginase-1 [92]. Inhibiting M2 macrophages abrogated myocardial repair and regeneration post-injury [87, 93].
The cellular and inflammatory microenvironment determines MSC phenotype and their effects on the immune system. MSCs may be induced to adopt either pro- or an anti-inflammatory phenotype (reviewed in detail in [94]). MSCs with a predominantly pro-inflammatory signature are associated with early stage infection and inflammation. For example, stimulation of Toll-like receptor 4 (TLR4) with LPS or TLR2 (peptidoglycan) results in a pro-inflammatory, “MSC1” phenotype (analogous to the M1 macrophage), whereas priming of TLR3 with poly I:C or ds DNA results in a MSC2 phenotype with secretion of immunosuppressive mediators [95]. It has been recently suggested that in vitro MSC expansion media can affect this phenotype, with platelet lysate-supplemented media favoring a pro-inflammatory MSC phenotype [96], highlighting the fundamental importance of the culture expansion conditions for the success of future trials.
In addition to targeting inflammation, MSC administration may enhance active biochemical and metabolic repair. Specifically, MSCs secrete lipoxins, derived by the sequential actions of lipoxygenases and other enzymes to produce bioactive trihydroxytetraenes, structures that are found in all eicosanoids of this class, e.g., lipoxin A4 (LXA4). LXA4 is a specialized proresolving lipid mediator that dampens excessive inflammation, promotes resolution, and protects from leukocyte-mediated tissue damage. Although the mechanisms of action are not fully understood, previous studies suggested that aspirin-triggered 15-epi-LXA4 induces apoptosis in neutrophils in situ and facilitates resolution of myeloperoxidase-sustained neutrophil-dependent pulmonary inflammation [42]. Coculture of human MSCs with alveolar epithelial type II cells in the presence of cytomix significantly increased the production of LXA4. In vivo, blocking the LXA4 receptor with WRW4, a LXA4 receptor antagonist, in a murine model of LPS-induced lung injury significantly reversed the protective effect of MSCs on both survival and the accumulation of pulmonary edema [42].
17.3.3 Regulation of Genes That Modulate the Response to Injury and Repair
Studies looking at changes in gene expression in recipients following MSC administration point to the role of MSCs in “reprogramming” the inflammatory response network [97]. MSC administration results in a coordinated modulation of the host transcriptional response characterized by an overall downregulation of inflammation and inflammation-related genes and a shift toward upregulation of genes involved in reducing inflammation, phagocytosis, and bacterial killing. MSC administration results in transcriptional reprogramming of multiple genes involved in the host response to injury and repair. As well, MSC administration alters the expression of genes responsible for the pro-inflammatory M1 macrophage phenotype together with an increase in anti-inflammatory M2 macrophage determinants. Our own group employed a genome-wide expression microarray approach to perform an “unsupervised” network analysis in major organs commonly affected by sepsis following MSC administration. In addition to the expected changes in pathways associated with inflammation and tissue repair, we found that treatment with MSCs returned transcriptional pathways responsible for maintaining mitochondrial function and consequently cellular bioenergetics to control levels. This finding is consistent with the possibility of mitochondrial transfer between MSCs and cells of the host organs as proposed by Islam et al. [31]. Moreover, reduction in innate immune pro-inflammatory transcription responses was also observed concurrently with upregulation of genes tasked with maintaining microvasculature integrity [98]. This data indicates that the paradigm that a single MSC-derived paracrine mediator is responsible for the global pleotropic effect of MSCs on transcriptional and network reprogramming is absurd. A much more plausible explanation is that MSC-conferred protection from acute injury involves a range of complementary activities, resulting in mitigation of the innate and acquired immune and inflammatory responses while also affecting complex networks of host cell-cell, cell-matrix, metabolism and bioenergy substrate utilization, and functional pathways. The dramatic effect of stem cells on gene transcription suggests MSCs may generate a coordinated genetic response by regulating gene expression at a higher gene organizational level that depends on complex network of transcription factors and histone modifiers, in concert with specific transcriptional co-activators and co-repressors that activate or repress MSCs [99, 100].
Regulation of gene expression at the epigenetic level occurs via modifications of chromatin architecture by facilitating the opening of DNA (euchromatin) to permit transcription or the condensing of DNA (heterochromatin) to repress transcription [101]. Accordingly, the architecture of chromatin is essential for the regulation of various chromatin-based cellular processes and is dynamically modulated through the orchestration of multiple mechanisms including histone modification, DNA methylation, chromatin remodeling, and noncoding RNA. At present, a large number of studies are focusing on identifying extrinsic regulators and their intrinsic target transcription factors that regulate MSC properties and functions. So far we know that a variety of histone-modifying enzymes are involved in the dynamic regulation of MSC trilineage differentiation [100]. In addition, epigenetic changes seem to play a critical role in the ability of MSCs to cross oligolineage boundaries and differentiate into different cell types, such as neurons, cardiomyocytes, hepatocytes, and endothelial cells, under appropriate culture conditions, indicating that these cells are extremely plastic. Importantly, various chemical genetic approaches, specific combination of small cell-permeable biological active compounds that are involved in the regulation of chromatin structure and function, and interfere with specific cell signaling pathways, may be used for reprogramming MSCs [102]. For example, histone modifiers alter the differentiation of MSCs into neuronal [103, 104] or cardiomyocyte [102, 105] cells. Moreover, the epigenetic-modifying drug such as BIX-01294 (a histone G9a methyltransferase inhibitor) can enhance endothelial differentiation of adipose tissue-derived MSCs through upregulation of several endothelial markers and factors associated with blood vessel formation [106]. The relative biological significance of the role of epigenetic regulation to the therapeutic potential of MSCs comes from the diabetes and the senescence literature. MSCs are responsive to metabolic cues including circulating glucose levels. Long-term exposure of undifferentiated human adipose tissue stromal cells to high glucose upregulates a subset of inflammation response genes by altering their promoter histone methylation patterns in a manner consistent with transcriptional derepression [107]. Genetic alteration studies demonstrate that DNA methyltransferases are responsible for maintaining methylation, and their loss results in significant myeloid skewing and self-renewal defects (reviewed in detail in [108]). Evidence that MSCs can be primed to express epigenetic “marks” provides an argument for priming cells in an effort to engineer MSCs that can impart specific biological properties to recipients [109, 110].
17.3.4 Transfer of Cellular and Genomic Contents such as Mitochondria and MicroRNAs
Recently, a novel mechanism for cellular communication involving long-distance intercellular connections called tunneling nanotubes (TNTs) has come to light [111]. TNTs are actin-based cytoplasmic extensions that not only facilitate direct communication between distant cells and intercellular trafficking [112] but also are associated with the transfer of biomolecular cargos such as organelles, Golgi vesicles, plasma membrane components, and even pathogens [113–115]. BM-derived MSCs have been shown to have the ability to transfer mitochondria to other cell types via these TNTs, thus restoring aerobic respiratory function in cells with defective mitochondria [116]. Mitochondrial transfer is thought to augment macrophage function by improving mitochondrial bioenergetics. As reported for alveolar epithelial cells, recovery of the energetic function of macrophages is characterized by an increased ability to generate ATP under conditions in which the cells exhibit mitochondrial uncoupling or an enhanced proton leak and involves protection of the macrophage by reducing mitochondrial ROS generation [117, 118]. In a mouse model of ALI, intratracheal delivery of BM-derived MSCs resulted in mitochondrial transfer from MSCs to alveolar epithelial cells via Connexin-43- containing gap junctions [31]. Following lung injury, human mitochondrial DNA was detected in mouse lungs, and human MSCs and their exosomes were shown to prevent accumulation of inflammatory (Ly6Chigh) monocytes and reduce the production of inflammatory and pro-fibrotic cytokines [119]. Importantly, MSCs carrying defective mitochondria did not ameliorate lung injury, and increased mouse survival was only evident following administration of wild-type MSCs. More recent studies demonstrate that mitochondrial Rho GTPase 1 (MIRO1), a Rho GTPase, functions as a calcium-sensitive adaptor protein that drives mitochondria along microtubules [120].
Cells can also communicate with each other by secreting small vesicles into the extracellular milieu, known as extracellular vesicles (EVs) [121, 122]. EVs are submicron vesicles, which, based on their size, origin, morphology, and mode of release, can be categorized into exosomes (40–200 nm), microvesicles (50–1000 nm), apoptotic bodies (50–5000 nm), or Golgi vesicles [123]. EVs are secreted by a multitude of cell and can be isolated via several conventional as well as high-throughput technologies. Importantly, these vesicles carry a repertoire of mRNAs, miRNAs, DNA, proteins, and lipids that can be transferred to neighboring cells, modifying their phenotype as well as the microenvironment [123–125]. The molecular signatures of EVs are selective to each cell/tissue type, which makes them ideal source for clinical applications [126]. Various studies have shown stem cell-EVs traffic stem cell-associated transcription factors associated with self-renewal of stem cells such as Nanog, Oct-4, HoxB4, and Rex-1 [127] key factors in the maintenance of pluripotency. They have also been shown to express MSC markers such as CD105 [128], prominin-1/CD133 [129–131], and KIT [132]. More direct effectors of the stem cell phenotype such as WNT [133], β-catenin [134], and Hedgehog [135] have also been identified on stem cell-EVs. Moreover, key biological features of stem cells, such as self-renewal, differentiation, and maturation, could be mimicked by stem cell-associated EVs [136–138].
Targeting of proteins into shedding vesicles appears to be entirely selective [139]. Specific proteins may be included or excluded from membrane EVs, leading to the expression of proteins arrays that differ from those present on the surface of the cells from which they originated. The most comprehensive proteomic characterization of MSCs and exosomes to date detected 580 membrane-associated proteins including those required to meet the minimal criteria for MSC classification (CD73, CD90, CD105) across various MSC samples [139]. Precondition alters the proteins in exosomes [140, 141]. Exposure to ischemic tissue conditions enhanced the proangiogenic properties of exosomes, facilitating localized tissue healing. A total of 1927 proteins were identified in MSC-derived exosomes from three different donors following exposure to ischemic conditions, of which 457 were not expressed under baseline conditions, suggesting MSCs can be made to generate “tailored” exosomes [142].
Exosomes derived from MSCs may have the capability to mediate much of the functionality traditionally associated with canonical secretory proteins such as growth factors of the MSC secretome [143–147]. Exosomes, in particular, are small lipid-bound, cellularly secreted vesicles [148] that mediate some of the tissue healing properties of MSCs [149]. In addition, EVs from lung cells have been shown to induce bone marrow cells to express mRNAs coding for lung-specific proteins such as Clara cell protein, aquaporin-5, and surfactants. Furthermore, this phenomenon is enhanced significantly during lung injury [150]. Of importance to the management of ARDS, KGF mRNA is present in microvesicles, suggesting that the reparative function might stem from boosting certain growth factors in the recipient. Administration of MVs derived from human MSCs can improve survival in part through KGF secretion and decreased the influx of inflammatory cells, cytokines, protein, and bacteria in mice injured with bacterial pneumonia. In primary cultures of human monocytes or alveolar type 2 cells, the uptake of MVs may be mediated by CD44 receptors. MVs enhance monocyte phagocytosis of bacteria while decreasing inflammatory cytokine secretion and increased intracellular ATP levels in injured alveolar epithelial type 2 cells. Prestimulation of MSCs with TLR3 agonist further enhances the therapeutic effects of the released MVs [151].
Of specific interest is the transfer of microRNAs in exosomal vesicles. Until recently it was thought that stem cells can rejuvenate damaged cells by mitochondrial transfer, but recently it has been shown that mitochondrial transfer by MSCs may not be an altruistic event but rather may serve to enhance the survival of MSCs. By unloading partially depolarized mitochondria, MSCs can obviate death. MSCs manage intracellular oxidative stress by targeting depolarized mitochondria to the plasma membrane via arrestin, a domain-containing protein 1 (ARRDC1)- mediated microvesicles. Vesicles containing these depolarized mitochondria are in turn engulfed by macrophages. Elimination of depolarized mitochondria is a priority for MSCs that experience high mitochondrial ROS generation when cultured under stressful conditions (such as in the presence of atmospheric oxygen tension) [152]. In parallel, however, MSCs also shed microRNA-containing exosomes that inhibit macrophage activation by suppressing TLR signaling, thereby desensitizing macrophages to the ingested mitochondria [119]. Exosomes modulate TLR signaling and cytokine secretion in macrophages, in part, by transfer of regulatory microRNAs. A microRNA contained within MSC-derived exosomes is miR-451, known to suppress TNF, and macrophage migration inhibitory factor [153, 154]. Based on this new evidence, it seems that transfer of mitochondria that have escaped mitophagy may be pro-inflammatory and pro-injurious [155, 156]. Therefore, silencing TLR responses in macrophages is likely necessary to induce tolerance to transferred mitochondria. Circumstances would have it that the immunomodulatory activities of MSC-derived exosomes may have evolved, in part, as a mechanism by which MSCs survive oxidative stress and serendipitously confer on cells the ability to suppress inflammation, in lung injury models [119].
17.4 Summary and Future Directions
Although this is an exciting time for immunotherapy for ARDS, the issue of safety remains a lingering concern in the field. A recent meta-analysis has comprehensively summarized the safety of systemic MSC administration in humans. Lalu et al. were unable to detect associations between MSC treatment and the development of acute infusional toxicity, organ system complications, infection, death, or malignancy. Importantly, this group also showed that there was no evidence of increased susceptibility to infection with MSC administration. Although MSC immunomodulatory effects may be beneficial in pro-inflammatory diseases, these same effects may leave a patient susceptible to infection. Development of novel stem cell or stem cell-like products with wide pleotropic effects that target competing pathological processes in ARDS offers a unique opportunity for advancement in the field. Many questions remain including identifying specific mechanisms of action, potency, dose, frequency, and markers of biological efficacy and response to therapy. As we move toward large-scale multicenter randomized clinical trials, it will be important to define specific mechanisms of action, the potential for engineering performance enhanced cell-products, and the development of cell-free adjuncts that may perhaps replace cell therapy.
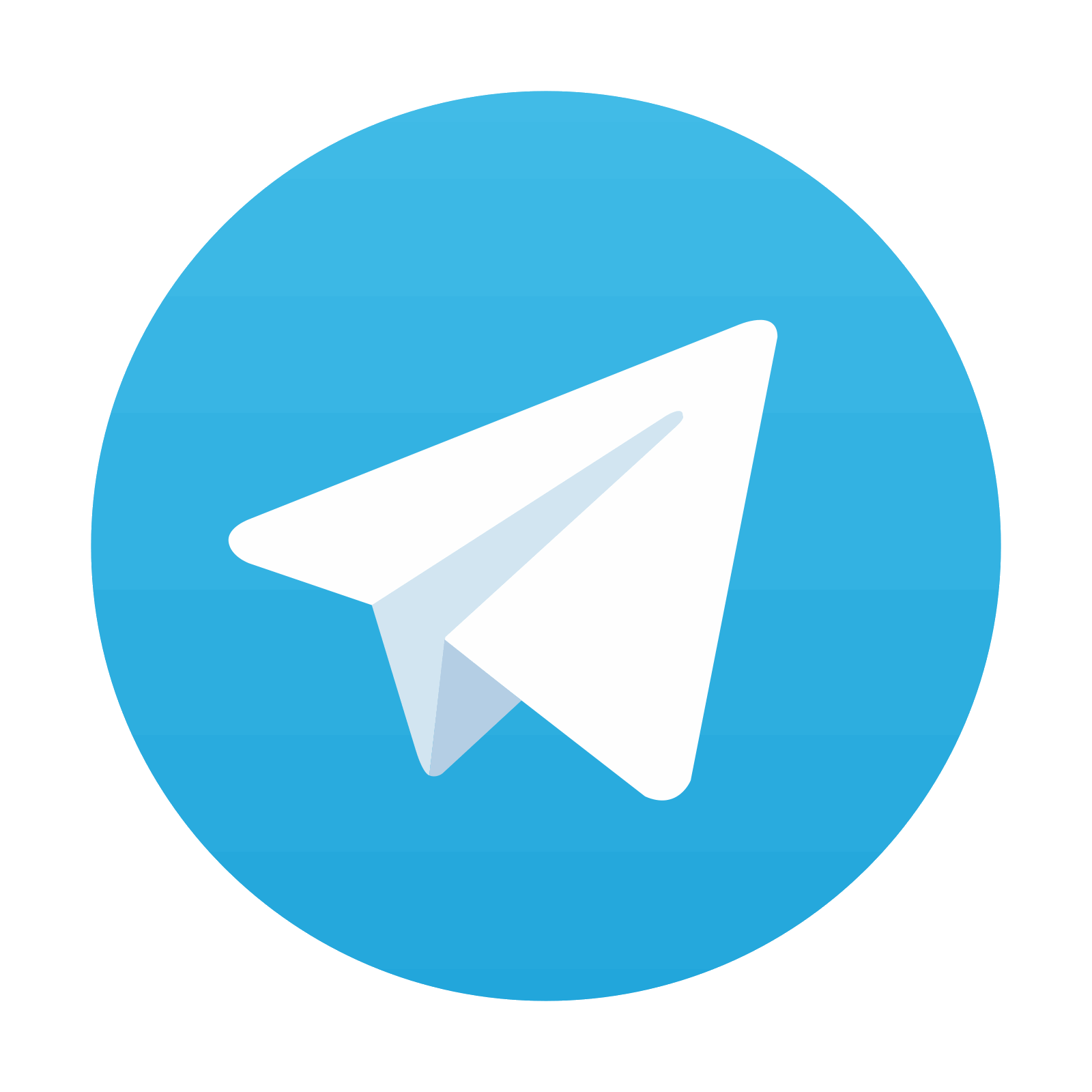
Stay updated, free articles. Join our Telegram channel
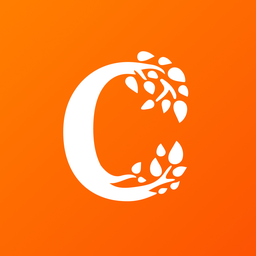
Full access? Get Clinical Tree
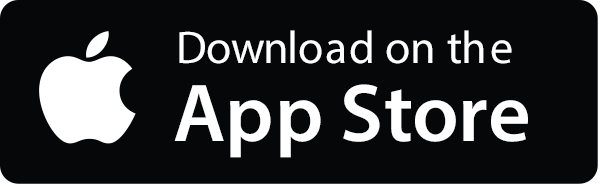
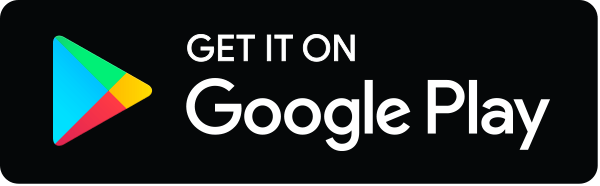