3 Spinal Cord Regeneration Charles H. Tator There are two broad categories of strategies to promote repair of the injured spinal cord: neuroprotection and regeneration (Table 3.1). To date, neuroprotection has not produced major recovery after spinal cord injury (SCI); treatment with agents such as steroids or antiinflammatory agents has been disappointing, especially for injuries of major severity, such as American Spinal Injury Association (ASIA) grade A injuries, with complete absence of voluntary motor function and sensation below the level of the injury. Even for less severe injuries, neurologic improvement in patients treated with neuroprotective agents has been minimal1 and not without controversy.2 Ideally, clinicians would like to have a variety of effective neuroprotective agents to counteract the many secondary injury mechanisms that are known to cause progressive damage to the injured spinal cord. Furthermore, it would be highly desirable to have effective neuroprotective strategies for patients with injuries of all severities. The secondary injury mechanisms that require treatment include ischemia, inflammation, excitotoxicity, major electrolyte shifts, and apoptosis. Patients with less severe injuries would benefit if these agents prevented further damage to the spinal cord, and patients with the most severe injuries would benefit from limiting the extent of the damage. The pathologic effects of acute SCI spread centrifugally in all directions to cause ventral-dorsal, lateral, and rostrocaudal damage.3 Ideally, effective neuroprotective agents would limit the pathologic spread and shorten the distance that regenerating axons would have to travel rostrocaudally in the cord. It is well known that the end result of this cascade of pathophysiologic events is major necrosis and cavitation, a process that has been termed posttraumatic infarction of the cord.3,4 The cavity that forms can be up to several centimeters long rostrocaudally, and thus large portions of spinal cord tissue would have to be replaced by actual ingrowths from the stumps or replacement by transplantation to improve function. In general, it is highly likely that improvement of neurologic recovery after major cord injuries would require a combination of regenerative strategies designed to produce regeneration or replacement of lost tissue. The regenerative strategy that is most likely to be successful is transplantation of cells or tissues, but it is unlikely that this will be sufficient. The goal of functional recovery will require a combination of strategies that include neurotrophic factors and agents to minimize scarring and to counteract endogenous inhibitors that are known to be plentiful in the injured cord.1 Also, combination therapy must include attention to rehabilitative measures such as gravity-assisted ambulation, and some of these rehabilitative measures have proven effectiveness.5,6 Table 3.1 Two Categories of Strategies Promoting Spinal Cord Repair
Neuroprotection | • Has not produced major recovery after spinal cord injury (SCI) |
• Includes treatments involving agents such as steroids or antiinflammatory agents | |
• Ideally, future use may protect patient from secondary injury such as ischemia, inflammation, excitotoxicity, electrolyte shifts, and apoptosis and prevent necrosis and cavitation | |
• Agents limit pathologic spread of injury and shorten distance for regenerating axons | |
Regeneration | • Likely that regeneration is needed for improvement in neurologic recovery |
• Strategy with greater chance of success is the transplantation of cells or tissues | |
• Combination of strategies likely to be required |
The Treatment Window at Various Stages of Spinal Cord Injury
Neuroprotective strategies such as surgical decompression, are designed to counteract the secondary mechanisms of injury. Each strategy has a specific time course and therapeutic window for effectiveness.7 It is also apparent from experimental studies that regenerative strategies are subject to therapeutic time windows. For example, the acutely injured spinal cord is less amenable to the survival of transplanted cells. There is greater survival of transplanted cells in the subacute stage.8–11 However, the chronic stage may be the most difficult with respect to improving neurologic function because of the long distances that axons would have to regenerate. For example, axons continue to die back for several months after SCI. Also, the classic view is that the astrocytic scarring that develops progressively in the subacute and chronic stages will hinder axonal regeneration, although this is still controversial.
Table 3.2 Specific Mechanisms to Enhance Regeneration after Spinal Cord Injury
Promotion of endogenous regeneration | Includes: |
• Regeneration of axons | |
• Collateral sprouting of axons | |
• Renewal of astrocytic or oligodendrocytic cells | |
• Blood vessels by angiogenesis | |
• Neuronal somata | |
Regeneration by replacement with transplantation | Includes: |
• Oligodendrocytes or Schwann cells for myelination | |
• Astrocytes for axonal guidance | |
• Neurons to act as “relay stations” | |
• Synthetic channels for axonal guidance and prevention of scarring | |
• Hematopoietic cells | |
• Microglia/macrophages |
Types of Regeneration
There are a two main types of regeneration that can be accomplished (Table 3.2). The first depends on regrowth of endogenous cells, and here there are many cell types that need to be considered. The more severe the lesion, the greater the number of cell types that will have to be regrown to accomplish restoration of function. For example, with mild lesions, regeneration of axons or sprouting of collaterals may suffice, whereas more severe lesions may require regeneration of axons, glia, and blood vessels. Regeneration of neuronal cell bodies is possible, but it is extremely unlikely that they would establish useful functional relationships. Similar considerations relate to the specific aims of regeneration, as indicated in Table 3.3. It may be feasible to devise strategies to replace several cell types, and this may be possible with stem/progenitor cell transplantation.
With respect to axonal regeneration and collateral sprouting, there are several anatomical sites where this can occur, as shown in Fig. 3.1. Axonal regeneration is defined as occurring from the injured axon itself, either from the damaged end or from a more proximal location on the damaged axon. In contrast, collateral sprouting occurs from an undamaged axon, possibly a neighboring axon in the same tract or from an axon in a different tract. The collateral may grow at the injury site or from a remote site. As well, a new neuron at the origin of the tract in the cerebral cortex or brainstem may give rise to a replacement axon.
Table 3.3 Aims of Regenerative Strategies
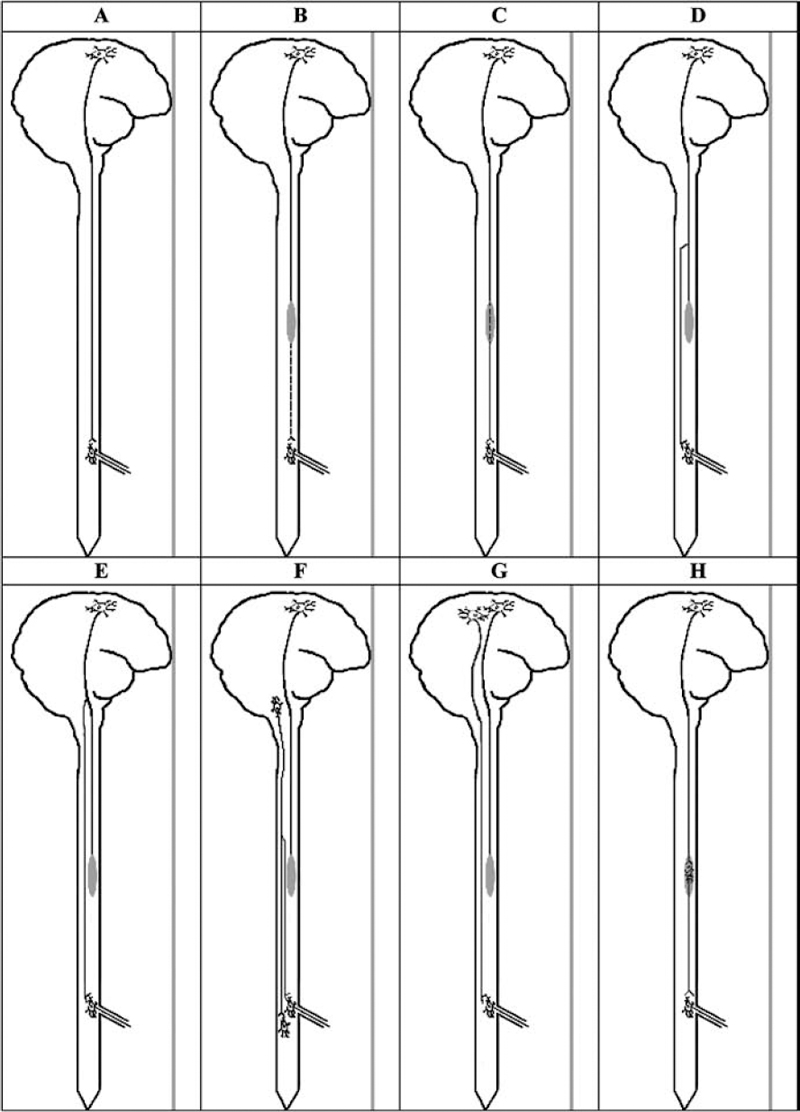
Fig. 3.1 Types of regeneration and regrowth after spinal cord injury (SCI), which may involve regeneration from the original damaged axon, at or remote from the injury site, or from another neuronal soma at the origin of the tract in the cerebral cortex or brainstem. In contrast, collateral sprouting is from a neighboring undamaged axon at or remote from the injury site. (A) Normal brain and spinal cord showing an axon from the corticospinal tract synapsing with an anterior horn cell in the lumbar cord. (B) Thoracic spinal cord injury (shaded area) has completely interrupted the axon, which then shows caudal wallerian degeneration. (C) Axonal regeneration from the original damaged axon. (D) Axonal regeneration from a rostral segment of the originally damaged axon in the thoracic cord. (E) Axonal regeneration from a proximal segment in the brainstem of the originally damaged axon. (F) Collateral sprouting from an adjacent undamaged axon of another tract, such as the rubrospinal tract, which then grows caudally to synapse with the original denervated anterior horn cell. (G) Collateral sprouting from an adjacent neuron in the cerebral cortex giving rise to an axon of the corticospinal tract, which then regenerates to synapse with the original denervated anterior horn cell. (H) Transplantation of a neuron into the injury site, which then gives rise to an axon that synapses with the original denervated anterior horn cell.
Aims of the Regenerative Strategies
The necrotic tissue or cavity left behind after acute and subacute/chronic injuries, respectively, have to be replaced with functioning living tissue, and there are many strategies that have been designed in an attempt to accomplish this. Regrowth of tissue from the surviving stumps has been attempted in a variety of ways (Table 3.3). One such way is the promotion of axonal regeneration, which is described as regrowth from the stump of the original transected or otherwise damaged axon. This can be contrasted with sprouting, which is classified as regrowth of an axon from a nearby intact axon. The former is much more difficult to accomplish but might result in more accurate functional improvement. After very severe injuries that transect all the axons present at the site of injury, regeneration from transected axons and transplantation are the only ways to regenerate axons. Regrowth of the supporting tissue including astrocytes and oligodendrocytes would be possible from the stumps, although these cells do not appear to be very mobile, and even if they multiplied in the stumps, as astrocytes are known to do, it is unlikely that they could migrate the long distances necessary to bridge the two stumps. Thus, restoration of glial tissue may have constraints similar to axons, in that replacement by transplantation may be necessary to bridge the large cavities after major SCI.
It is also necessary to consider revascularization of the restored tissue because SCI causes major ischemia of the injured segment, and therefore it would be highly desirable to be able to stimulate regrowth of the vasculature in the area of injury (Table 3.3). Some agents, such as fibroblast growth factor-1 (FGF-1), promote angiogenesis. Other older, unproven strategies such as omental transplantation to restore blood supply12 are not reviewed in detail here.
Overview and Classification of the Types of Regenerative Strategies for Spinal Cord Injury
Table 3.4 shows the broad variety of focused regenerative strategies that have been studied in experimental models of SCI. Many of these strategies have also been tried in patients, including neurotrophic factors, cell and tissue transplantation, electrical stimulation, and neutralization of inhibitors. Most of the studies in experimental models of SCI have been in the acute and subacute stages, although some also have been in the chronic stage. The studies in patients have been mainly in the subacute and chronic stages and have mostly been phase 1 trials. There have been several comprehensive reviews of the experimental strategies8,13–15 and several reviews of the clinical strategies.1,16–18 Most of the recent clinical trials of regeneration have been phase 1 trials of the transplantation of cells or tissues involving patients in the subacute and chronic stages of SCI. Unfortunately, very few of these trials have been published in peer-review journals and are not available in any other format in the public domain. Issues regarding design of cell transplantation trials for central nervous system (CNS) diseases in general have been critically reviewed by Cesaro19 and commented on by Sagen.14 The need for great care in translation is evident from the study of Hofstetter et al,20 who showed allodynia-like hypersensitivity of the forepaws due to aberrant axonal sprouting in rats after transplantation of adult neural stem cells into the injured thoracic spinal cord. Furthermore, Dobkin et al21 recently issued a warning about poor trial design, complications, and lack of effectiveness of transplanted olfactory ensheathing glia (OEG) cells in seven patients treated in one very active transplant center. The present chapter provides the experimental background for 25 main strategies and a brief description of any clinical trials of specific strategies.
Table 3.4 Classification of Types of Regenerative Strategies
Neurotrophic factors | • Nerve growth factor (NGF) |
• Brain-derived growth factor (BDGF) | |
• Neurotrophin 3 (NT-3) | |
• Fibroblast growth factor-1 or -2 (FGF-1 or -2) | |
• Epidermal growth factor (EGF) | |
• Many others | |
Matrices and scaffolds | • Collagen |
• Matrigel | |
• Millipore | |
• Synthetic materials (hydrogels, polyesters) | |
• Natural materials (chitosan) | |
Neuronal/axonal growth stimulation | • Cyclic adenosine monophosphate |
• Gangliosides | |
• Inosine | |
Cell and tissue transplantation | • Spinal cord segments |
• Peripheral nerve grafts | |
• Schwann cells | |
• Macrophages | |
• Porcine stem cells | |
• Blood stem cells | |
• Bone marrow stromal stem cells | |
• Umbilical cord/blood stem cells | |
• Olfactory ensheathing glia or bulb stem cells | |
• Human embryonic stem cells | |
• Many others | |
Stimulation of endogenous stem cells | • Growth factors |
• Erythropoietin | |
Electrical stimulation | • Direct current fields |
• Alternating current | |
Reduction of scarring | • Enzymes (chondroitinase ABC) |
Manipulation of extracellular matrix | • Tenascin |
• Many others | |
Neutralization of inhibitors | • Immunotherapy with antibodies |
• Anti-Nogo antibodies | |
• Nogo receptor antagonists | |
• Rho inhibition | |
Fusion molecules | • Polyethylene glycol |
Manipulation of signaling molecules | • Ephrins |
• Semaphorins | |
Rehabilitation strategies | • Exercise |
• Functional electrical stimulation (FES) | |
• Gravity-assisted ambulation | |
Immunomodulation | • Immunization |
• Macrophages |
Space does not permit detailed description of some of the other strategies listed in Table 3.4.
Neurotrophic and Growth Factors (Table 3.5)
Neurotrophic and growth factors have been major strategies in experimental SCI.22,23 Many of these agents have produced remarkable growths of proliferating glial, fibrous, and Schwann cells. In our laboratory, direct injection of brain-derived neurotrophic factor (BDNF) produced the most remarkable proliferation of cells at the injury site, with virtually complete disappearance of the cavitation.24 To date, these agents have not been used in SCI patients, except for the Cheng et al25 application of FGF-1 in patients in whom peripheral nerve grafts were transplanted into the spinal cord. However, two of these agents have been used in patients with amyotrophic lateral sclerosis (ALS). Ciliary neurotrophic factor (CNTF) was delivered intrathecally through an indwelling catheter attached to a pump in a phase 1 trial in four patients with ALS. There was no improvement, but there were complications and side effects.26 An initial study with BDNF delivered subcutaneously in ALS patients was not beneficial, and the subsequent trial of BDNF by intrathecal catheter and pump was a phase 3 randomized prospective controlled trial organized by Amgen,27 but the final result has not been reported.
Table 3.5 Neurotrophic and Growth Factors
Brain-derived neurotrophic factor (BDNF) | • Produced the most remarkable cell proliferation at site of injury |
• Virtually complete disappearance of cavitation | |
• To date, not used in SCI patients (except FGF-1 in Cheng study) | |
• Used in amyotrophic lateral sclerosis (ALS) patients; delivered subcutaneously and no benefits seen | |
Ciliary neurotrophic factor (CNTF) | • Used in ALS patients |
• Delivered intrathecally via catheter | |
• No improvement; complications and side effects present |
Note: These factors have been major strategies in experimental SCI and have shown growth of glia, fibroblastic, and Schwann cells.
Manipulation of Endogenous Spinal Cord Stem Cells (Table 3.6)
The use of endogenous stem cells for regeneration of the spinal cord after SCI is a highly attractive method for enhancing regeneration of the spinal cord that theoretically could result in the regrowth of new glial cells and new neurons. This strategy is based on the relatively recent discovery by Reynolds and Weiss28 in 1996, which noted the presence of endogenous stem cells in the adult mammalian spinal cord. This has now been confirmed in several laboratories, including mine, and has led to extensive experimentation on methods of stimulating these stem cells to proliferate, differentiate, and migrate into the injured area after SCI. One of the major problems is that the most multipotential cells are located in the ependyma region around the central canal, whereas those located in the parenchyma of the cord are progenitors with less potential.29 Central hemorrhagic necrosis and posttraumatic infarction are cardinal features of the pathology of acute SCI and cause the destruction of the stem cells at the epicenter of the lesion and rostrocaudally for a considerable distance.3,30 Most of the efforts to enhance the proliferation and migration of endogenous stem cells have been with growth factors such as epidermal growth factor (EGF) and FGF-2 and have been successful in increasing the proliferation of these cells but have resulted in only minimal improvement in functional recovery in experimental SCI.29 There have also been efforts to enhance survival of these endogenous stem cells after injury, but mainly in brain injury.31
GM1 Ganglioside (Table 3.7)
GM1 ganglioside is present in neuronal membranes and was studied extensively in animal models of SCI and CNS ischemia, as reviewed by Geisler et al.32 It is of interest that this agent also has neuroprotective effects. After an initial positive result in a small series of patients, a subsequent multicenter randomized prospective control trial in approximately 800 patients failed to show an overall significantly improved neurologic recovery, although the authors pointed to some improvement in the ASIA grade B incomplete injuries.33,34 This was a landmark study and provided a great deal of useful information about the epidemiology and natural history of neurologic recovery in patients with SCI.
Table 3.6 Endogenous Stem Cell Usage for Regeneration
Logic | Theoretically, can enhance regrowth of glial cells and new neurons, as precursors of these stem cells (progenitors) were discovered in adult mammalian spinal cord |
Problems | Multipotential cells are located in ependyma regions around central canal (those in parenchyma of cord have less potential); necrosis and infarction associated with SCI will kill these cells at injury site, with some spread rostrocaudally |
Growth factors | EGF and FGF-2 have been used successfully to increase proliferation of stem cells; however, functional recovery minimal |
Transplantation of Whole Spinal Cord Segments (Table 3.8)
Reier et al35 pioneered the implantation of fetal spinal cord in experimental SCI, and there have been some remarkable examples of the potential for this method in experimental studies. One of the best examples was produced by Iwashita et al,36 who transplanted fetal spinal cord into the completely transected spinal cord of newborn rats and showed impressive fusion of the transplant with the adjacent spinal cord stumps and excellent neurologic recovery. Patients with syringomyelia in Sweden37 and the United States38 were treated with implants of human fetal spinal cord in an attempt to obliterate the cavities and stop the progression of neurologic deterioration. It appears that the procedure can be performed without major complications, but the survival of the grafts and long-term benefit have not been proven.
Table 3.7 GM1 Ganglioside in Regeneration
Logic | • May be useful as it is located in neuronal membranes |
• May have neuroprotective effects as well | |
Geisler et al’s randomized prospective controlled trial (RPCT) | • No overall improvement in neurologic recovery |
• Some improvements in ASIA grade B, incomplete SCI patients | |
Relevance | This study provided information on epidemiology and natural history of neurorecovery in SCI patients |
Transplantation of Autologous Activated Macrophages (Table 3.9)
Schwartz et al39 in Israel studied the effect of transplanting into spinal cords, and found homologous macrophages from the peripheral blood that had been incubated with peripheral nerves and found improved recovery in rats with experimental SCI. In a phase 1 trial in patients, autologous activated macrophages prepared from the peripheral blood of patients with SCI were incubated in tissue culture conditions in the presence of autologous skin, then injected at surgery into the injured spinal cord just caudal to the epicenter of the injury within 14 days of injury. Three of eight ASIA grade A thoracic cases showed improved neurologic recovery to ASIA grade C,40 and a second publication about this strategy in 2005 reported that 5 of 14 patients treated had neurologic improvement.41 A further trial is in progress.
Table 3.8 Fetal Spinal Cord Implantation Method for Regeneration
Washita et al study | • Transplanted fetal spinal cord into completely transected stem cells of infant rats |
• Results: | |
1. Fusion with adjacent stumps | |
2. Excellent neurorecovery | |
Treatment for syringomyelia | • Goal: obliterate cavities and halt neurologic deterioration with transplants of human fetal spinal cord |
• Results: | |
1. Procedure done without complications | |
2. Survival of grafts and long-term benefits not yet proven |
Peripheral Nerve Grafts (Table 3.10)
Several clinical applications of peripheral nerve grafts have been reported based on the initial animal experiments of Richardson et al’s group.42 Cheng et al43 then showed remarkable recovery in rats after complete transection of the thoracic spinal cord. In Olson’s laboratory in Sweden, this group inserted peripheral nerves and FGF-1 in fibrin glue to bridge from white to gray matter.43 Cheng et al25 then applied this strategy to a patient in Taiwan with a chronic SCI from a stab wound at 4 years after injury and reported recovery of neurologic function; other patients have also been treated with this strategy in Taiwan (Cheng, personal communication). Peripheral nerve grafts have also been used in eight patients from Brazil without apparent benefit.44 In one patient, Brunelli and von Wild45,46 in Italy inserted autologous sciatic nerves into the corticospinal tract of the spinal cord rostral to the injury site and then anastomosed them to the femoral nerves to restore quadriceps function and directly to the gluteus muscles several months after injury; then reported improvement. Tadie et al47 in France reported a similar case in which peripheral nerve grafts bridged from the cord rostral to the injury site to the L2-L4 spinal roots. Several years earlier, Carlstedt et al48 in Sweden reported recovery of upper limb function after brachial plexus injuries treated with implantation of the avulsed nerve roots directly into the spinal cord. In 10 patients with root reinsertion from 10 days to 9 months after injury, the results were beneficial in at least three cases. Thus, there is some evidence that peripheral nerve bridging is worth pursuing.
Table 3.9 Autologous Activated Macrophages in Regeneration
Autologous activated macrophages | • Autologous macrophages from peripheral blood, incubated with peripheral nerves of skin |
Phase I trial (phase 2 trial started and then discontinued) | • Autologous macrophages injected caudal to injury site within 14 days of injury |
• Results: | |
1. Three of eight ASIA grade A thoracic cases showed improved neurologic recovery | |
2. Five of 14 (in another publication) patients had neurologic improvements |
Transplantation of Bone Marrow Stem Cells (Table 3.11)
Whole bone marrow contains a mixture of hematopoietic cells, various mononuclear cells such as macrophages, and marrow stromal cells. The initial reports in animal studies showed that populations of bone marrow-derived stem cells showed neuronal and glial differentiation and improved neurologic recovery after transplantation into the injury site after SCI.49 These studies generated a large number of other animal trials in experimental SCI models,50 and now also in patients in several countries. The major attractions of this strategy are the ease of obtaining autologous tissue for transplantation and the possibility that bone marrow cells have a “homing instinct” so that they may be effective after administration remote from the injury site, through either intravascular or intrathecal routes. In 2005, Park et al51 in South Korea reported that whole bone marrow transplantation into the injury site produced improvement in ASIA grades in all five patients in a phase 1 trial who received whole bone marrow transplants into the injured spinal cord within 7 to 14 days of injury. All five patients also received intravenous injections of granulocyte-macrophage colony-stimulating factor (GM-CSF), whereas a sixth patient who also recovered received only the latter. There are other trials of this strategy in China, the Czech Republic, and Russia, as noted in my recent review.1
Transplantation of Peripheral Blood Stem Cells (Table 3.11)
For reasons similar to those underlying the use of bone marrow-derived stem cells, peripheral blood-derived stem cells have been used in patients with spinal cord diseases. Janson et al52 reported a trial of intrathecal administration in patients with ALS, but there were few details. There are also unreported trials of this strategy in Brazil and Russia.
Table 3.10 Peripheral Nerve Graft Studies
Cheng et al43 | • Inserted peripheral nerves and FGF-1 in fibrin glue, bridging white to gray matter in rats with neurorecovery |
• Neurologic recovery after stab wound in one patient with peripheral nerve grafts into cord | |
Barros et al44 | • Nerve grafts into spinal cord showed no benefits |
Brunelli and von Wild | • Autologous sciatic nerve inserted in corticospinal tract and anastomosed to femoral nerve and gluteus muscles; showed improved muscle function |
Tadie et al47 | • Peripheral nerve graft bridging from spinal cord rostral to injury to L2-L4 roots; improved muscle function |
Carlstedt et al48 | • Implantation of avulsed nerve roots directly into spinal cord; recovery of upper limb function following brachial plexus injury |
Umbilical Cord Stem Cells (Table 3.11)
Currently, homologous umbilical cord blood stem cells are being transplanted into patients with chronic SCI in China. The transplantations are performed intravenously, intrathecally, or directly into the spinal cord. There are some published trials of the use of human umbilical cord stem cells in experimental SCI that have shown some benefit.53,54
Table 3.11 Stem Cell Transplantation
Bone marrow stem cells | Animal study reports | • Neuronal and glial differentiation and neurologic recovery following bone marrow stem cell implants into SCI site |
Advantages | • Ease of obtaining autologous tissue | |
• “Homing instinct” of marrow cells | ||
• Administer intravascularly or intrathecally | ||
Park et al study | • Improvements in ASIA grades in patients receiving marrow stem cell transplants within 7 to 14 days of injury | |
• Patients also received granulocyte-macrophage colony-stimulating factor (GM-CSF) | ||
Peripheral blood stem cells | Janson et al study | • Intrathecal administration to ALS patients |
• Few details of study found | ||
Umbilical cord stem cells | China study | • Homologous umbilical cord stem cell transplants in chronic SCI patients |
• Performed intravenously, intrathecally, or directly into spinal cord | ||
• Reportedly showed benefits in some published trials; no definite proof | ||
Fetal porcine stem cell xenotransplantation | • Diacrin Company performed trial, injecting pig stem sells directly into human spinal cord; no publication of results | |
Human embryonic stem cells | • At least one study in United States with no reports yet; one planned trial of particular interest involves differentiation in vitro of human embryonic cell line to an oligodendrocytic phenotype with functional competence for myelination, which could be very beneficial in SCI patients where demyelination is extensive |
Transplantation of Olfactory Ensheathing Glia and Olfactory Bulb (Table 3.12)
Table 3.12 Olfactory Ensheathing Glia and Olfactory Bulb Transplantation
Advantages | • Autologous tissue obtained easily for use in SCI patients |
• Has shown benefits in experimental models | |
Huang et al57 studies | • Use of human fetal olfactory ensheathing glia (OEG) or olfactory bulb transplanted into spinal cord at open surgery |
• Guest et al58 reported rapid recovery of one case | |
• Dobkin et al21 criticized trial design and recorded subsequent cases of serious complications, including meningitis | |
• More trials in progress; incomplete reporting of results and poor trial design |
Transplantation of OEG is currently being performed in patients with SCI in several countries, including China, Portugal, Russia, and Australia, and is based on many favorable reports in experimental models.55,56 The strategy is attractive because of the ease with which autologous tissue can be obtained for transplantation in patients with SCI. Huang et al57 in China has had the largest experience with this technique, and in 2003, they reported the results in 171 patients, mostly in the chronic phase, in a case series report. They transplanted OEGs or olfactory bulb tissue from human fetuses into the spinal cord at open surgery. Unfortunately, there was no blinding of examiners and no randomization, and neurologic improvement was reported after follow-up of only 8 weeks. Recently, Huang reported that he has performed transplantation of autologous OEG cells into the spinal cord in more than 300 SCI patients (personal communication). Guest et al58 reported rapid recovery of one of Huang’s cases but could not determine the exact mechanism. As noted above, Dobkin et al21 criticized this trial on the basis of poor trial design, and they recorded complications, including meningitis. These authors also recorded the absence of neurologic improvement in all seven patients who had objective neurologic assessment before and after transplantation of the OEG cells. Other investigators in China have also been performing this treatment (personal communication), and a similar large trial of OEG transplantation is in progress in Lisbon, Portugal, by Lima et al,59 who reported the results in seven ASIA grade A patients, all of whom improved in a pilot study that was not a randomized control trial.59 An Australian phase 1 trial of OEG transplants in three patients was reported in 2005,60 and a larger trial is being planned for Australia and New Zealand.
Table 3.13 Schwann Cell Transplantation
Miami Project | Has studied Schwann cell strategy for years in experimental animals |
Zhu study | Injection of Schwann cells from peripheral nerves into spinal cord of SCI patients showed improvements in ASIA and sensory scores |
Feng study | Injection of Schwann cells from sural nerve into spinal cord of SCI patients showed some recovery |
Schwann Cell Transplantation (Table 3.13)
The Schwann cell strategy has been pursued in experimental SCI for several decades by many investigators, especially those at the Miami Project. Based on animal studies with human Schwann cells transplanted into the spinal cord of rats with demyelination,61 there have been plans to transplant Schwann cells into the spinal cord of patients with conditions such as multiple sclerosis, but no clinical reports have been published in peer-reviewed journals. Recently, Zhu and colleagues in China injected human fetal Schwann cells harvested from peripheral nerves into the spinal cord of 47 patients with SCI and found improvement in ASIA motor and sensory scores (personal communication). Feng and colleagues in Tianjin, China, injected autologous Schwann cells from sural nerve into the spinal cord of nine SCI patients with apparent recovery in some (personal communication). There is no indication that the examiners were independent or blinded in these apparently phase 1 trials.
Fetal Porcine Stem Cell Xenotransplantation
In the United States, the Diacrin Company trial of fetal stem cells injected directly into the spinal cord involved 10 patients with SCI in two centers, St. Louis and Albany, New York (personal communication), but no report has appeared in the literature.
Human Embryonic Stem Cells
There are a large number of promising preclinical trials of various human embryonic stem cells, some of which have been differentiated toward a neural phenotype.62,63 Some of the initial experimental paradigms in this field were not relevant to humans, such as mouse embryonic stem cells transplanted into rats with SCI.64 However, there has been at least one phase 1 study of human embryonic stem cells in SCI patients in the United States (personal communication), but no published report has appeared. One of the most interesting has been a human embryonic cell line differentiated in vitro to favor an oligodendrocytic phenotype with functional competence for myelination in rats.65–67 There is extensive demyelination associated with human SCI, and thus this strategy is very attractive. It is being prepared for a phase 1 trial in SCI (personal communication).
Immunomodulation (Table 3.14)
Immunomodulation | • Alter immune response in spinal cord to enhance regeneration |
• Done via vaccination or depletion of circulating macrophages by liposome-encapsulated clodronate | |
• Both cell-mediated and antibody-mediated immune responses can help in axonal regeneration | |
Fusion molecules (polyethylene glycol) | • Evidence that polyethylene glycol can repair neuronal membranes in vitro via “fusion” |
• Clinical trial being considered | |
Cyclic AMP | • Injected directly as dibutyryl cAMP or inhibition of cAMP hydrolysis by a phosphodiesterase IV inhibitor (rolipram); can enhance axonal regeneration following SCI |
Rho antagonist | • Inactivation of Rho via Rho kinase inhibition can promote axonal regeneration after experimental SCI |
• Cethrin, a Rho inhibitor applied extradurally at time of surgery; phase 1 trial in patients has been completed with promising results |
Some of the strategies already discussed, such as transplantation of autologous macrophages, may have an immunomodulation basis, but there have been other specific attempts at altering the immune response in the spinal cord designed to enhance regeneration by vaccination68 or by depletion of circulating macrophages by measures such as liposome-encapsulated clodronate.69 As discussed below, myelin contains molecules that inhibit regeneration of axons, and neutralizing the activity of these inhibitors can enhance axon regeneration. Thus, there are complex CNS-immune system interactions after SCI, and both cell-mediated and antibody-mediated immune responses can help in promoting axonal regeneration. The above efforts to develop an effective vaccine or cytokine treatment for experimental SCI have not yet been examined in human SCI.
Table 3.15 Bioengineering in Neuroprotection and Regeneration
Examples | • Axonal guidance channels |
• Subarachnoid drug delivery systems | |
Advantages | • Opportunity to counteract secondary injury |
• Provides scaffolds to replace lost tissue and enhance regrowth of axons | |
• Synaptic plasticity | |
• Inhibition of astrocytosis | |
• Structural and chemical versatility | |
Clinical trials | None yet |
Polyethylene Glycol Application
There is evidence that polyethylene glycol can repair neuronal membranes in vitro by “fusion,” and there has been support for this concept in experimental SCI models.70,71 A clinical trial is being considered (personal communication).
Scaffolds, Matrices, and Other Bioengineering Strategies (Table 3.15)
Bioengineering has been of major importance for exploring new methods of spinal cord repair, and our group has recently reviewed the field, including our experiences with some of these strategies, such as axonal guidance channels and subarachnoid drug delivery systems.72 As stated above, the pathophysiology of SCI is multifactorial and multiphasic; therefore, it is likely that effective treatments will require combinations of neuroprotection and regeneration strategies. Bioengineering offers opportunities to counteract secondary injury, provide scaffolds to replace lost tissue, and enhance axonal regrowth, synaptic plasticity, and inhibition of astrocytosis.73 Biomaterials have major advantages for spinal cord repair because of their structural and chemical versatility. For example, bioengineering technology can facilitate cellular treatment strategies, including Schwann cells,74 olfactory ensheathing glia, or neural stem cells for repair of the injured spinal cord. Currently, the emphasis in our laboratory is on the use of naturally occurring agents, such as chitosan, and to make them biodegradable. To date, none of these strategies has been applied to humans for spinal cord regeneration.
Anti-Nogo-A Inhibition and Nogo-66-Receptor Inhibition (Table 3.16)
Table 3.16 Myelin-Based Protein Inhibitors
Function of myelin-based proteins | • Serve as powerful inhibitors of neurite outgrowth in vitro and axonal regeneration in vivo |
Examples | • Nogo-A, Nogo-66 receptor |
Anti-Nogo-A antibody | • Counteracts Nogo-A inhibitory protein in vitro and in vivo in rodent and primate studies; phase 1 trial in humans is under way |
It is now known that there are several myelin-based proteins that are powerful inhibitors of neurite outgrowth in vitro and axonal regeneration in vivo. The most extensively studied of these inhibitors is known as Nogo-A, and it has been cloned and characterized by Schwab and colleagues in Zurich. Nogo-A is a protein present in oligodendroglial myelin and is one of at least three myelin-based inhibitors of central axonal regeneration identified in the CNS. Schwab and colleagues have prepared an antibody to Nogo-A, and this anti-Nogo-A antibody counteracts the Nogo-A inhibitory protein in vitro and in vivo in several experimental models of SCI in various species, including rodents and monkeys.75,76 A “humanized” form of the anti-Nogo-A antibody is currently being administered intrathecally to patients with SCI in a phase 1 trial in Germany and Switzerland in the centers of the European Consortium (see below; personal communication).
Table 3.17 Reducing Glial Scar Formation
Glial scar | • Contains extracellular matrix (ECM) molecules, including chondroitin sulfate proteoglycans (CSPGs), which are inhibitors of axonal growth in vitro |
Chondroitinase ABC | • Administered intrathecally |
• Counteracts inhibitory proteoglycans of ECM | |
Reports | • Several reports from experimental SCI studies show effectiveness |
• Some reports show enzyme may even enhance collateral axonal sprouting in central nervous system (CNS) remote from injury | |
Clinical trials | • Likely will be subjected to clinical trials in humans with SCI |
Other recently described efforts in animal studies to overcome inhibitory factors include antibodies or inhibitors of the Nogo-66 receptor protein.77,78 These agents might be more effective than anti-Nogo-A antibody, which may neutralize only Nogo and not the other myelin-based inhibitors.
Chondroitinase ABC (Table 3.17)
At sites of CNS injury, a glial scar develops, containing extracellular matrix (ECM) molecules, including chondroitin sulfate proteoglycans (CSPGs). CSPGs are inhibitory to axon growth in vitro, and regenerating axons stop at CSPG-rich regions in vivo. Chondroitinase ABC has been administered intrathecally to counteract these inhibitory proteoglycans in the ECM in experimental SCI.79,80 Recently, several other reports have appeared showing the effectiveness of this enzyme on recovery from experimental SCI, including combination strategies with scaffolds and various cellular transplants.81–86 Also, it is interesting that this enzyme may enhance collateral axonal sprouting in the CNS remote from the injury site, which may confer additional benefits.87 It is highly likely that this agent will be subjected to clinical trials in humans with SCI.
Cyclic Adenosine Monophosphate
It was first reported in 1991 that cyclic adenosine monophosphate (cAMP) enhances neurite outgrowth in vitro,88,89 and recently it has been shown that this agent can enhance axonal regeneration after experimental SCI.90–92 It can be injected directly as dibutyryl cAMP, or cAMP levels can be increased by inhibition of cAMP hydrolysis by the phosphodiesterase IV inhibitor rolipram. This strategy has also been used in combination therapy with neurotrophic factors and with cellular transplants.92 Clinical trials are likely to appear.
Rho Antagonist
McKerracher and colleagues93,94 showed that inactivation of Rho by Rho kinase inhibition can promote axonal regeneration after experimental SCI. Cethrin is a Rho inhibitor and is now undergoing a multicenter phase 1 trial. The agent is applied extradurally at surgery following surgical treatment for decompression or fusion within 14 days of SCI in ASIA grade A cervical or thoracic patients. It is uncertain whether this type of downstream intracellular inactivation of the inhibitory pathways is more effective than inhibition at the axonal membrane as afforded by anti-Nogo-A.
Electrical Stimulation with Direct Current Fields (Table 3.18)
It has been well established that electrical stimulation with direct current (DC) fields enhances the growth and alters the direction of neurite outgrowth in vitro.95–97 Many studies of this modality were performed in experimental SCI by Borgens et al98 in a variety of animal models of SCI and with several different forms of electrical stimulation. For many years this strategy was actively pursued in my laboratory but was ultimately abandoned because the therapeutic index was so unfavorable.99,100 A phase 1 trial of pulsed oscillating current in 10 patients was reported by Shapiro et al101 in cervical and thoracic ASIA grade A cases. Stimulation began within 18 days of injury with surgically implanted electrodes, and some patients showed neurologic improvement. Pulsed electrical stimulation of the spinal cord after SCI in humans has also been performed by Xu and Liu in Beijing, China, on more than 100 patients (personal communication).
Table 3.18 Technology and Rehabilitation in Regeneration Enhancement
Electrical stimulation with direct current fields | • Enhances growth and alters direction of neurite outgrowth in vitro |
• Many trials done | |
• Has unfavorable therapeutic index | |
Functional electrical stimulation (FES) | • Stimulation of muscles to augment intact neurologic input or serve as the sole stimuli to produce movements |
• Remains questionable whether it plays a role in neurologic recovery | |
• Treatments require much labor and are expensive | |
Gravity-assisted ambulation and automated locomotor training | • Gravity-assisted ambulation (body weight support) embodies treadmill training |
• Can improve walking, especially in incomplete SCIs | |
• Great need for randomized prospective controlled trial but effectiveness is proven | |
Electrical stimulation with alternating currents | • Some evidence that this can assist in motor recovery; unproven value |
• Unknown relation to neurologic recovery | |
Rehabilitation and exercise strategies | • Exercise enhances recovery via regeneration (e.g., treadmill training) |
• Does so through elaboration of neurotrophic factors | |
• Also provides muscle strength and joint mobility |
Rehabilitation-Exercise Strategies Enhance Regeneration (Table 3.18)
It has been shown that certain physical rehabilitation measures can enhance neurologic recovery by enhancing regeneration, and it is likely that these measures should begin immediately after SCI to derive the maximum benefit. It is now known that physical exercise enhances regeneration of the CNS through the elaboration of neurotrophic factors. Cotman’s group102–104 has been at the forefront in defining the physiologic basis of exercise through the elaboration from the CNS of neurotrophic factors such as BDNF. In experimental SCI, the effect of exercise on neurologic function has been firmly established.105,106 Thus, physiotherapy and occupational therapy in patients with SCI not only enhance muscle strength and preserve joint mobility, they also increase neurotrophic factors capable of promoting axonal regeneration. These findings have prompted clinical SCI trials to include early, vigorous, and consistent rehabilitation practices to maximize neurologic recovery. Also, investigators must ensure that defined rehabilitation programs are applied equally across treatment and control groups. In experimental SCI, these goals of enhanced and consistent physical activity are extremely difficult to achieve, but excellent studies have been done in experimental SCI, showing that measures such as treadmill training likely enhance regeneration.107,108
Functional Electrical Stimulation and “Patterned Neural Activity”
Functional electrical stimulation (FES) is accomplished through stimulation of the muscles to augment any intact neurologic input or to provide the sole stimuli to produce muscle contraction to perform a patterned, sequential function, such as walking or peddling a bicycle. The current question being examined is whether patterned neural activity such as provided by FES can play a role in improving neurologic recovery. Also, it is important to examine the mechanism of this modality in experimental models. It is unknown whether recovery is based on axonal regeneration. This treatment modality was applied to Christopher Reeve in a much publicized report purporting to show that his neurologic recovery was attributable to the treatment.109 These treatments are labor intensive and involve large expenditures for equipment, training, and personnel, and thus, they impact significantly on clinical trials. Phase 1 trials of this modality have been reported.110 There is a need for intensive examination of this strategy in experimental SCI.
Gravity-Assisted Ambulation and Automated Locomotor Training
This new modality of treatment of SCI patients has been termed gravity-assisted ambulation or body weight support and generally embodies treadmill training.111 It has been shown that this method of rehabilitation can improve walking after SCI, especially in patients with incomplete injuries.5 A multicenter trial to evaluate this modality has shown that walking is improved in ASIA grade C and D patients.6 Further study by a randomized prospective controlled trial is essential for the same reasons noted above for FES. The precise mechanism for the improvements is not known, but one study with transcranial magnetic stimulation in patients with chronic SCI provided evidence that treadmill training increased locomotor function through an increase in function of the corticospinal tract.112 A multicenter randomized trial commenced in 2003 and has not yet been reported.113 It is conceivable that there is increased axonal sprouting or regeneration, even in chronic cases. Thus, there is a great need for further clinical and experimental studies of this modality.
Electrical Stimulation of the Spinal Cord by Alternating Current
There is a long history of the use of various forms of alternating current for stimulation of the spinal cord in experimental SCI to improve function,114 and there is some evidence that electrical stimulation can assist motor recovery in patients. There is one report of improvement in walking in an ASIA grade C patient with an implanted extradural spinal cord stimulator.115 It is unknown whether the functional improvement is related to neurologic recovery.
Conclusion
A large number of strategies have been proven to enhance regenerative processes in experimental in vitro and in vivo studies related to regeneration of the spinal cord. Many of these involve elaboration or administration of growth factors, and many involve stimulation of the body’s endogenous regenerative mechanisms. Transplantation of various cells, especially a large variety of stem cells, has shown impressive effects on the pathologic picture. For example, it is possible to produce enough new tissue to completely fill the major cavities left behind after severe SCI. However, functional restoration of complex activities such as grasping and locomotion continue to elude investigators at both the experimental and clinical levels. In clinical SCI, a large number of trials of strategies to enhance regeneration are in progress in many countries, and several hundred SCI patients have been treated with cellular transplantation techniques. Most of the trials have been phase 1 with no controls, no independent examiners, and no reports in peer-reviewed literature or other sources in the public domain. Lack of rigorous attention to trial design and lack of detailed reporting make scientific evaluation impossible. Further, well-designed trials are necessary based on sound experimental SCI study of these strategies in clinically relevant experimental models.
References
21. Dobkin BH, Curt A, Guest J. Cellular transplants in China: observational study from the largest human experiment in chronic spinal cord injury. Neurorehabil Neural Repair 2006;20:5–13
58. Guest J, Herrera LP, Qian T. Rapid recovery of segmental neurological function in a tetraplegic patient following transplantation of fetal olfactory bulb-derived cells. Spinal Cord 2006;44:135–142
66. Keirstead HS. Stem cells for the treatment of myelin loss. Trends Neurosci 2005;28:677–683
76. Schwab ME. Nogo and axon regeneration. Curr Opin Neurobiol 2004;14:118–124
95. Jaffe LF, Poo MM. Neurites grow faster towards the cathode than the anode in a steady field. J Exp Zool 1979;209:115–128
< div class='tao-gold-member'>
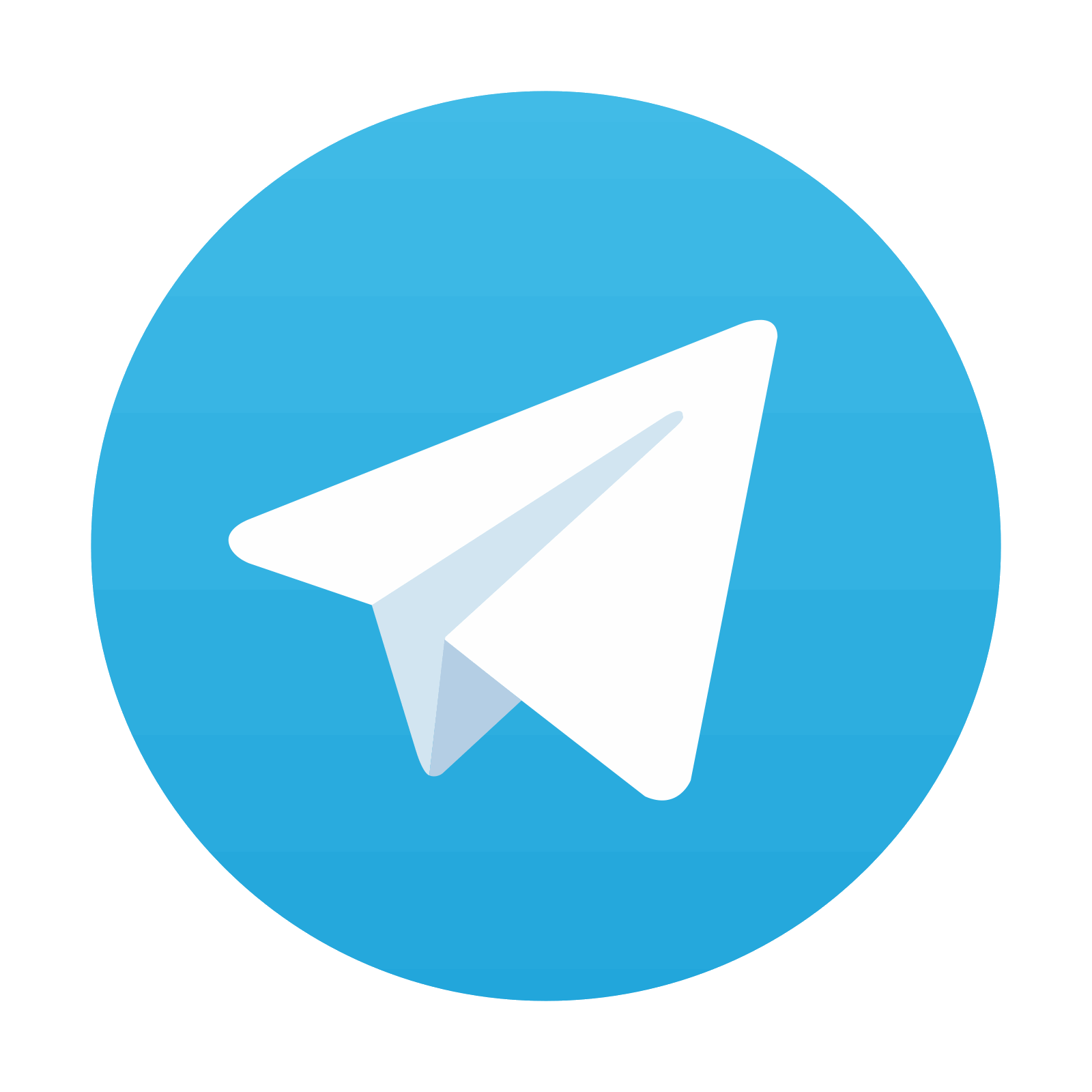