Key Points
- ▪
Sleep is a dynamic neuronal and behavioral state that can be characterized using specific electroencephalographic, electrophysiologic, and behavioral findings.
- ▪
Characteristics of sleep can be quantified using questionnaires, actigraphy, or respiratory polygraphy. However, polysomnography, including electroencephalogram, electrooculogram, submental electromyogram, and analysis of breathing, is required to describe the cortical characteristics and immediate physiological consequences.
- ▪
Activation of hypothalamic sleep-promoting pathways including the ventrolateral preoptic nucleus and the median preoptic nucleus produces the physiological switch from wakefulness to sleep.
- ▪
Sleep and anesthesia can look similar. Although small doses of anesthetics can induce sleep by activating sleep-promoting pathways, surgical anesthesia and immobilization cannot be induced by sleep-promoting pathways.
- ▪
Control of breathing is altered during sleep and anesthesia, typically leading to a decreased drive to upper airway dilator and respiratory pump muscles.
- ▪
Persistent respiratory depressant effects of anesthetics and neuromuscular blocking drugs increase the risk of postoperative respiratory complications, particularly in patients with obstructive sleep apnea.
- ▪
Anesthesia and surgery, as well as treatment in the intensive care unit and opioids, affect sleep duration and sleep architecture, which may lead to impaired outcome.
Acknowledgment
The editors, publisher, and Drs. Sebastian Zaremba and Matthias Eikermann would like to thank Dr. Nancy L. Chamberlin for her contribution to this chapter in the prior edition of this work. It has served as the foundation for the current chapter.
Probably the earliest mention of “overpowering sleep” as a metaphor describing what possibly characterizes anesthesia can be found in Genesis 2:21: “And the Eternal God caused an overpowering sleep to fall upon the man and he slept. He took one of his ribs and shut in flesh instead thereof.” Although this “overpowering sleep” allegedly occurred by divine intervention and did not represent the drug-induced unconsciousness and immobility that characterizes anesthesia, the basic narrative is an example of antiquarian history that implicates the concept that deep sleep may be the only viable condition that allows a surgical procedure to be completed successfully.
In the 21st century, an increasing body of knowledge has revealed the common ground between sleep and anesthesia, as well as their fundamental differences in clinical picture and underlying mechanisms.
Sleep is required for survival. Rats deprived of sleep will die within 2 to 3 weeks, a time frame similar to death due to starvation. In humans, sleepiness can be deadly. Approximately 100,000 motor vehicle accidents each year result from drivers who are “asleep at the wheel.” In a survey of drivers in New York State, approximately 25% reported they had fallen asleep at the wheel at some time. In addition, sleep deprivation impairs the psychomotor performance of night shift workers, specifically surgeons and residents. In patients, perioperative sleep deprivation occurs frequently, particularly in critically ill patients, and there may be consequences on patients’ outcomes. Among sleep disorders, obstructive sleep apnea (OSA) has probably the most meaningful consequences for perioperative treatment.
Therefore, high-quality physiologic sleep is a key domain of public health that needs to be considered in perioperative medicine from both a provider and a patient perspective.
Definition of Sleep
Sleep is the natural periodic suspension of consciousness during which the powers of the body are restored. A behavioral definition of sleep includes a species-specific posture and behavioral quiescence, as well as elevated arousal thresholds. However, sleep is much more than an absence of activity. The brain is highly active during sleep, especially during rapid eye movement (REM) sleep, during which atonia, phasic muscle movements (driven by different activation levels in distinct areas of the brain), and vivid dreaming occur. Sleep is not a simple electrophysiological phenomenon. At different stages of sleep, brain activities can be as distinct from each other as they are from wakefulness. In humans and other vertebrates (see “Evolution”), sleep has two major stages: REM sleep and non-REM (NREM) sleep, with each of these further divided into substates. Most of sleep time is spent in NREM sleep, which is characterized by relatively low electroencephalographic (EEG) frequencies with higher amplitudes compared with awake EEG frequencies ( Fig. 10.1 ). In the transition from wake to NREM sleep, the fast activity of waking disappears (α–θ transition), and then in the deeper stages of NREM large, slow waves (i.e., δ waves) emerge. Thus deep NREM sleep is also referred to as slow-wave sleep . NREM sleep is associated with waxing and waning muscle tone, as well as decreased body temperature and heart rate. In contrast, REM sleep is characterized by muscle atonia, loss of slow waves in the EEG, and bursts of REMs that give this state its name. Other prominent features of REM include marked irregularities of respiration and heart rate, as well as penile and clitoral erection. REM sleep is associated with the high likelihood of vivid dreaming. A distinct property of REM sleep is the operation of a system that suppresses motor activity, which is responsible for baseline atonia and suppression of motor commands that would otherwise result in acting out of dreams, a phenomenon known as REM sleep behavior disorder . This REM atonia system is not constant, as it periodically allows breakthrough muscle activity that comprises REM sleep and twitching of the extremities. REM sleep has therefore been subdivided into tonic REM sleep (i.e., a period with muscle atonia and without eye movements) and phasic REM sleep , which is interrupted by short phasic events of the eye and other movements. The pattern and amounts of NREM sleep, REM sleep, and wakefulness throughout the night are referred to as sleep architecture , and there are many physiologic and pathophysiologic processes that can affect sleep architecture. For example, many antidepressant medications, benzodiazepines, and opioids selectively suppress REM sleep. A disease example is narcolepsy, in which individuals often transition rapidly from wake to REM, whereas normally REM is nearly always entered from a prolonged episode of NREM state.

Physiology
Evolution
It is unknown why we sleep; however, one can imagine that evolutionary pressure would favor systems that are optimized for nature’s rhythms. In a rhythmic world, organisms must adapt to alternating changes in the environment, such as daily and seasonal rhythms in light intensity, ambient temperature, and humidity.
Circadian Rhythms
Species-dependent temporal behavior can be adapted depending on the environmental conditions: behaviorally, anatomically, and physiologically. Circadian rhythms affect almost every aspect of the body’s function, including activity and rest patterns, cognitive function (e.g., learning and memory), cardiovascular and endocrine physiology (e.g., heart rate, metabolism, and hormone secretion), and gene expression (15% of the genes in the human body show daily rhythms).
Virtually all species show circadian rhythms that regulate periodic changes in behavioral and physiologic parameters within a period of approximately 24 hours. These rhythms can be characterized as a synchronization of activity to the external light-dark cycle in the majority of living creatures. Bacteria, plants, animals, and humans exhibit such a behavior that helps them stay in tune with the environmental light-dark cycles. Our sleep timing preference, or chronotype, is a manifestation of our internal biological clock. Variation in chronotype has been linked to sleep disorders, cognitive and physical performance, and chronic disease.
Clock genes generate endogenous clock pulses in most (if not all) cells of the body, synchronized by regulatory pathways across the body to a superior rhythm generator (a so-called master clock). Desynchronization of these rhythms seems to be involved in the pathogenesis of metabolic, psychiatric, and other disorders. The superior rhythm generator in humans is located in the suprachiasmatic nucleus that receives external inputs about light and darkness from the retinal cells and synchronizes inputs of melatonin levels. While this master clock synchronizes the behavioral and biological rhythms of the human body to the changing demands of the environment during the solar day, sleep itself entrains circadian rhythms, and the temporal organization of circadian rhythms and nocturnal sleep must be preserved to accomplish a restful and refreshing experience that fulfills the sleep needs produced by the second major regulatory factor, sleep homeostasis. During waking, “sleep pressure” continuously increases, leading to an increased likelihood for the transition from wakefulness to sleep. Increasing homeostatic sleep pressure can be partly offset by a sufficient circadian wakening stimulus during the circadian wake phase, but the circadian wake stimulus disappears in the presence of an overwhelming sleep pressure. In this situation, sleep sufficient in quantity and quality is needed to reestablish proper functioning.
Although circadian and homeostatic regulation of sleep can be observed in almost all living organisms, differentiation of sleep into NREM and REM sleep (“integrated sleep”) probably has developed during the last 300 to 350 million years because it appears only in birds and the majority of terrestrial mammals ( Fig. 10.2 ).

Sleep Stages and Sleep Cycles
Sleep architecture—a structured temporal order of different sleep stages—is an important determinant of sleep quality. If tired individuals rest in their individual sleeping postures, the increasing level of drowsiness is paralleled by continuous EEG slowing from that seen during attention and cortical activation (16-30 Hz, “β activity”) to slower frequencies, predominantly between (7.5 and 11 Hz, “α activity,” typically observed in subjects with reduced attention and closed eyes). During this state of vigilance, the individuals do not sleep, and a full level of cognitive function can be established easily even without a strong sensory stimulus. The transition from α state to sleep is paralleled by a further decrease in α and β activity and an increase of EEG activity in the θ band (4.5-7.5 Hz; Fig.10.3 ).

This transition from α to θ activity is widely accepted as the EEG correlate of sleep onset. At this transition from wakefulness to NREM sleep stage 1, heart rate and heat production decrease, resulting in a slight decrease in body temperature. Furthermore, respiration is regular and deep. As sleep deepens, EEG activity shows low amplitudes and θ activity with intermittent sleep spindles and K-complexes. The latter represents an activation of brainstem and subcortical brain areas during sleep for selective processing of unexpected sensory inputs (e.g., sounds) that might require complete arousal and restoration of consciousness to address a potential threat. In accordance with that hypothesis, in comatose patients with brain injury, the presence of K-complexes in response to an acoustic stimulus during coma seems to be a marker of a better outcome compared with comatose patients with no evoked K-complexes. With increasing depth of sleep, during NREM sleep, electrical activity of the cortex measured by EEG is dominated by slower frequencies and high amplitudes, also referred to as slow-wave sleep .
REM sleep, or paradoxical sleep, is associated with an alteration of homeostatic regulation, such as increased heart rate variability, irregular respiration, and impaired body temperature control. Brain metabolism increases while EEG recordings show similarities to wakefulness with low-voltage and mixed-frequency power spectra. Prominent θ waves generated by the hippocampus occur. These waves are not apparent in the scalp EEG recording from humans, but in rodents, in which the hippocampus is larger and closer to the brain surface, the EEG is dominated by θ during REM. This sleep state is associated with a decreased tone in skeletal muscles (except for extraocular muscles that control eye movement). Dreaming is a typical experience during REM sleep, but can also happen during NREM sleep. During physiologic sleep, an individual typically switches back and forth across sleep stages, interrupted by occasional arousals from sleep ( Fig. 10.4 ).

Neuroanatomy of Sleep
Sleep-Promoting and Arousal Pathways
Several neuronal pathways have evolved to maintain cortical activation and behavioral arousal during normal waking, and others have evolved to promote and maintain sleep ( Fig. 10.5 ). It is the balance between these two systems that determines whether one is awake or asleep.

Ascending Arousal System
The ascending arousal system (AAS) is the main wakefulness-mediating network in the brain. Main pathways within the network receive cholinergic, monoaminergic, dopaminergic, and glutamatergic inputs. Cholinergic inputs originate from pedunculopontine and laterodorsal tegmental nuclei, innervate the lateral hypothalamus, prefrontal cortex, basal forebrain, and thalamic relay nuclei (i.e., medial and lateral geniculate nuclei; mediodorsal nucleus; pulvinar; anterior, ventral, and lateral thalamic cell groups). Glutamatergic neurons that provide inputs to the AAS are mainly located within a small area just ventral of the locus ceruleus (LC), the preceruleus area, as well as in the parabrachial nuclei that mainly project to the basal forebrain and the lateral hypothalamus. Monoaminergic inputs mainly come from noradrenergic neurons within the LC, histaminergic neurons of the tuberomammillary nuclei (TMN), serotoninergic neurons of the median and dorsal raphe nuclei, as well as dopaminergic neurons adjacent to dorsal raphe nuclei. Beside projections to the basal forebrain, multiple inputs are sent to the thalamus, mainly the interlaminar and reticular nuclei, as well as the amygdala and cerebral cortex. Furthermore, noradrenergic neurons of the LC project mainly to the posterior part of the lateral hypothalamus. In return, the latter area of the lateral hypothalamus projects to the LC and to the TMN.
Some neurons of the posterior hypothalamus produce orexin A and B, also known as hypocretin (HCRT) 1 and 2. These neurons project to the basal forebrain and the amygdala and cerebral cortex and other important arousal areas, and they are essential for stabilizing the waking state. Orexin deficiency causes narcolepsy (severe daytime sleepiness) with cataplexy (abrupt loss of muscle tone), a disorder showing some characteristics of REM sleep (i.e., REM sleep atonia during wakefulness [discussed later]).
Orexin System
Orexin and its cognate receptors (OX 1 R and OX 2 R) play a key role in sleep/wake regulation. This neurotransmitter system has a crucial involvement in the pathogenesis of narcolepsy, a disorder associated with chronic excessive daytime sleepiness, sleep attacks, and rapid transitions between vigilance states and sleep in humans. Studies in mice recapitulating the narcolepsy phenotype by targeted mutagenesis of the HCRT gene encoding the prepropeptide precursor of orexin neuropeptides, or the less pervasive phenotype of OX 2 R knockout mice, not only demonstrated the role of orexin signaling in sleep/wake regulation but also demonstrated biological proof of concept for the development of orexin receptor antagonists for the treatment of insomnia. In contrast, the activation of orexin pathways, either by exogenous administration of orexin receptor agonists or by optogenetic activation of orexinergic neurons in the lateral hypothalamus, increases arousal. Orexinergic neurons are counteracted by inhibitory γ-aminobutyric acid (GABA)-ergic neurons originating from the ventrolateral preoptic nucleus (VLPO). OX 2 R is expressed exclusively in the histaminergic TMN, whereas OX 1 R is expressed preferentially in the noradrenergic LC, and both receptors are present in cholinergic pedunculopontine and laterodorsal tegmental nuclei. Orexinergic tone at these hypothalamic and brainstem nuclei contributes to cortical arousal. TMN, the predominant source of histamine in the brain responsible for cortical arousal, receives a prominent projection from orexinergic neurons mediated predominantly by OX 2 R. Orexin receptor antagonists promote sleep mainly via inhibition of the OX 2 R receptor.
Non-Rapid Eye Movement Sleep-Promoting Pathways
About 100 years ago during the epidemic of encephalitis lethargica, it was observed that lesions in the preoptic region around the rostral end of the third ventricle were associated with profound insomnia, which was confirmed in neuroanatomic experiments (lesion studies) in rats and cats. Neurons in the preoptic area are active during sleep. Two key nuclei within this area are the VLPO as well as the median preoptic nucleus. VLPO neurons fire at a higher frequency during sleep compared with wakefulness. Anatomically, the VLPO consists of a dense core of sleep-active, galanin-positive neurons innervating the TMN (which is part of the AAS) while being surrounded dorsally and medially by a more diffuse population of sleep-active, galanin-positive neurons that project to the dorsal raphe and LC. Physiologically, the VLPO neurons constitute a sleep-promoting pathway that inhibits many components of the arousal system during sleep. Likewise, parts of the arousal system such as the laterodorsal tegmental nucleus and the pedunculopontine tegmental nucleus, as well as the LC, the parabrachial nucleus, the dorsal raphe nucleus, the PC, the ventral periaqueductal gray, and the TMN, are capable of inhibiting the VLPO. The mutually inhibitory relationship of the arousal and sleep-promoting pathways produces the conditions of a flip-flop switch, which can generate rapid and complete transitions between waking and sleeping states (see Fig. 10.5 ). It also makes simultaneous activation of arousal and sleep circuits highly improbable.
Because animal experiments showed that even large lesions of the VLPO substantially reduce but do not completely erase sleep, other brain areas beside this nucleus are likely involved in promoting sleep. Several basal forebrain areas, as well as some GABA-ergic interneurons across the cerebral cortex, have been implicated to function as sleep-active neurons. Nevertheless, the role of these brain regions in the promotion or regulation of sleep remains unclear.
Rapid Eye Movement Sleep-Promoting Pathways and Non-Rapid Eye Movement–Rapid Eye Movement Transition
During normal sleep that presents at onset as a sharp α–θ EEG activity transition, a similar sharp transition from NREM to REM sleep can be observed. Two groups of mutually inhibitory neurons located in the pons are involved in mediating the switch between NREM and REM sleep. The first group consists of REM active inhibitory neurons of the sublaterodorsal nucleus and the PC region. These neurons inhibit but are also inhibited by a second group of neurons that are located in ventrolateral periaqueductal gray and adjacent lateral pontine tegmentum. This mutually inhibitory relationship produces a REM-NREM flip-flop switch, promoting rapid and complete transitions between sleep states.
Within the sublaterodorsal nucleus and PC, glutamatergic neurons are mixed within the population of REM-on GABA-ergic neurons. The glutamatergic sublaterodorsal nucleus neurons project to the spinal cord and are important for REM sleep atonia; the glutamatergic PC neurons activate forebrain pathways driving EEG desynchronization and hippocampal θ rhythms—characteristic EEG signs of REM-sleep.
How to Assess Sleep
The complexity of signs and symptoms of sleep suggests that different instruments need to be applied to capture all the important elements of sleep.
Questionnaires
Several questionnaires are used for the assessment of sleep duration, sleep quality, and the related physiologic and pathophysiologic consequences. Quality of sleep is a frequent target of generic health surveys for measuring patient-reported outcomes. Other questionnaires quantify the consequences of sleep deprivation, specific sleep disorders, or both ( Table 10.1 ). The most commonly used instrument in sleep medicine is probably the Epworth Sleepiness Scale, a short questionnaire to assess symptoms of daytime sleepiness, expressed as intolerance to monotony. The questionnaire asks the subject to rate the probability of falling asleep on a scale of increasing probability from 0 to 3 for eight different situations, such as such as watching TV, reading, lying down in the afternoon, and so on. Despite the fact that the Epworth Sleepiness Scale is reliable for detection of daytime sleepiness, the instrument does not determine the mechanism of daytime sleepiness.
Questionnaire ∗ | Focus | References |
---|---|---|
Pittsburgh Sleep Quality Index (PSQI) | Sleep and sleep disorders | |
Sleep Quality Scale (SQS) | Sleep quality | |
Functional Outcome of Sleep Questionnaire (FOSQ) | Impact of daytime sleepiness on daily living | |
Pediatric Sleep Questionnaire | Sleep and SDB in children | |
Child Sleep Habits Questionnaire (CSHQ) | Sleep | |
Epworth Sleepiness Scale (ESS) | Daytime sleepiness | |
Sleep Diaries/Sleep Logs | Sleeping times, sleep duration | |
Morningness-Eveningness Questionnaire | Sleeping times, sleep duration, circadian rhythm | |
Loughborough Occupational Impact of Sleep Scale (LOISS) | Sleep quality | |
Insomnia Sleep Questionnaire (ISQ) | Insomnia | |
Berlin Questionnaire | SDB in surgical patients | |
STOP/STOP-Bang Questionnaire | Obstructive sleep apnea | |
Brief Insomnia Questionnaire (BIQ), | Insomnia | |
International Restless Legs Syndrome Study Group Rating Scale (IRLS) | Restless leg syndrome | |
Clinical Global Impression (CGI) Scale | Restless leg syndrome | |
Richard–Campbell Sleep Questionnaire | Sleep assessment in the ICU |
Other clinically useful questionnaires that focus on the detection of signs and symptoms of sleep disorders are available, which is important because the prevalence of sleep pathologies typically varies in a wide range across populations (see Table 10.1 ).
An effective process for clinical assessment of sleep-related diseases is a stepwise assessment using a screening tool first (e.g., Epworth Sleepiness Scale), followed by a more specific questionnaire that helps identify individual mechanisms or consequences of sleepiness.
A special form of sleep questionnaires comes in the form of sleep diaries and “morningness-eveningness” questionnaires that evaluate daily sleep habits, including sleep time, sleep duration, number of nocturnal awakenings, and subjective sleep quality.
Although questionnaires allow a quick and easy screening for daytime symptoms of sleep disturbances, they do not quantify sleep architecture. Subjective methods of assessing sleep are influenced by the spectrum of disease within a tested group, actual clinical change over time, the testing conditions, and recall bias. Clearly, a questionnaire cannot replace a medical history or objective assessment for sleep disorders. However, sleep questionnaires are important tools for measuring health improvement or decline, predicting medical expenses, assessing treatment effects, or comparing disease burden across populations.
Actigraphy
Actigraphy is used to study sleep-wake patterns by detecting motion of the wrist with linear accelerometers in single or multiple axes. Based on movement-derived data, predictions of the time spent during sleep and wakefulness can be made, and even assumptions on sleep staging are made. Actigraphy can be used conveniently in a patient’s home for several nights, weeks, or longer, but its validity for detecting transitions from wakefulness to sleep has been debated, especially in patients with high levels of sleep fragmentation. In the clinical setting, actigraphy has been used to evaluate sleep patterns in patients with insomnia, for diagnosis of circadian rhythm disorders (including shift work), and in evaluating sleep in individuals who are less likely to tolerate polysomnography (PSG), such as infants and demented elderly. However, its accuracy is limited for patients with reduced mobility, such as nursing home or intensive care unit (ICU) patients. Actigraphy allows for convenient follow-up measurements to evaluate the effects of treatments designed to improve sleep architecture and circadian rhythm disorders. In more recent years, an increasing number of wearable devices for the detection and analysis of activity and rest have been marketed. Although some have claimed to detect sleep accurately, as well as some sleep stages (mainly REM sleep), none of the current models are yet approved or validated for clinical use.
Respiratory Polygraphy
Home respiratory polygraphy (RP) can be a cost-effective alternative to PSG for the diagnosis of sleep apnea-hypopnea syndrome. RP usually calculates airflow through nasal pressure changes taken via nasal cannulae. Thoracic and abdominal movements are measured by piezoelectric bands, which also measure body position and blood oxygen saturation by pulse oximetry. Using these parameters, RP can identify apnea and hypopnea, and categorize the pathophysiology of apnea into obstructive versus central. Furthermore, similar to actigraphy, RP provides some analysis of sleep duration and time spent awake, using its body position and light sensors. However, the quality of the latter can be more precisely evaluated by a combination of sleep diary and actigraphy. Nevertheless, EEG is required to decide whether a subject was awake or sleeping ( Table 10.2 ).
Method | Pros | Cons | Recommendation |
---|---|---|---|
Sleep questionnaire | Low cost | Recall bias | Should be combined with interview |
Good compliance | Limited reliability in some populations | ||
Easy to use | Limited validity | ||
Sleep diary | Less recall bias | Lower compliance compared with other questionnaires | Should be combined with other measurement of sleep duration (e.g., actigraphy) |
Easy to administer | Biased by daily mood and expectations on sleep | ||
Low cost | |||
Documentation of daily variability | |||
Actigraphy | Provides objective information about daily variability and sleep duration | Limited assessment of sleep stages and sleep onset latency | Should be combined with other measurement of sleep duration (e.g., sleep diary) |
Provides information on sleep habits at home | Higher cost than questionnaires | ||
Not influenced by patient expectations, recall bias, or memory impairments | |||
Lower cost than laboratory polysomnography | |||
Polygraphy | Lower cost than laboratory polysomnography | Limited assessment of sleep | Should be accompanied by questionnaires and clinical interview |
Objective assessment of respiratory events | Higher cost than questionnaires | ||
Provide information on sleep habits at home | Limited assessment of sleep disorders other than sleep-disordered breathing | ||
Laboratory polysomnography | Objective assessment of sleep, sleep stages, and sleep disorders | High cost | Should be last step in sleep evaluation with previous questionnaires and ambulatory screening (e.g., polygraphy) |
First-night effect | |||
Limited capacity | |||
No information about sleep habits at home | |||
Out-of-center polysomnography | Objective assessment of sleep, sleep stages, and sleep disorders | Limited observation may lead to decreased recording quality | Good patient education mandatory |
Less first-night effect | If possible, surveillance recording preferred | ||
Lower cost than laboratory polysomnography | |||
Can provide information about sleep habits at home |
Polysomnography
PSG is the only method that can precisely determine the actual sleep stage and is a reference diagnostic tool (i.e., gold standard) that is required for the diagnosis of several sleep disorders. PSG measurements include EEG, electrooculography for the measurement of eye movements, and at least electromyographic measurement of muscle activity of the chin. These three measurements are typically supplemented by other channels for the detection of sleep-disordered breathing (SDB), such as a nasal sensor to detect apneas and hypopneas, oximetry, inductance plethysmography for respiratory effort of the chest and abdomen, and a body position sensor and leg electromyogram to identify periodic limb movement syndrome or REM sleep behavior disorder, respectively. A typical PSG recording is shown in Fig. 10.6 .

Sleep Laboratory Testing
For several decades, PSG was performed only within a sleep laboratory. Sleep laboratory PSG is attended by a trained technician and is analyzed by a clinician trained in sleep medicine according to the published guidelines. However, the high cost and requirement of an overnight stay can present socioeconomic challenges that affect the accessibility of this method. In-center PSG provides only a momentary view of the patient’s sleep, and the measurements themselves can impair patients’ sleep (the so-called first-night effect) and does not provide information about sleep at home. Multiple repeated measurements to tailor treatment over time are challenging or even impossible from a logistic and healthcare economics perspective. Therefore, an increasing number of out-of-center devices are now available, offering the possibility of recording PSG in almost every environment, such as at the patient’s home, within the hospital or nursing home room, and even within the recovery room or ICU.
Out-of-Center Testing
Portable out-of-center PSG sleep monitors are a reasonable, reliable, and effective method for diagnosing OSA compared with PSG recording performed within a sleep laboratory, when applied in preselected population at risk of moderate-to-severe OSA. The Portable Monitoring Task Force of the American Academy of Sleep Medicine (AASM) supports the use of these devices in subjects for clinical and research purposes and indicates a comparable quality of PSG data.
Scoring of Sleep and Sleep Disordered Breathing
More than 40 years ago, Rechtschaffen and Kales standardized the method of scoring PSG recordings—the R&K criteria. Since then, major innovations in technology have transformed the science and clinical practice of sleep medicine. The latest criteria of the AASM capture the full potential of innovations resulting from computerization of data, including automation of sleep scoring, recognition of disorders that occur during sleep, and integration with other procedures, such as positive airway pressure titration. Although the R&K criteria are still sufficient for clinical and research purposes, they are less commonly used in sleep centers around the world.
According to these criteria, the arousal states are categorized based on the EEG activity recorded from three different scalp locations and divided into wakefulness (W), REM sleep (R), and three NREM sleep stages ( Table 10.3 and see Fig. 10.1 ).
EEG and EOG Characteristics | AASM | |
---|---|---|
Wakefulness | α rhythm (8-13 Hz) present in more than 50% of an epoch | W |
Non-REM sleep stage 1 | Lower amplitudes and activity in range of 4-7 Hz (vertex sharp waves, ∗ slow eye movements ∗ ) | N1 |
Non-REM sleep stage 2 | EEG of sleep stage 1 with additional a sleep spindles and K-complexes; slow waves not fulfilling the criteria for sleep stage 3 | N2 |
Non-REM slow wave sleep | 20%-50% of slow wave activity (0.5-2 Hz) | N3 |
REM sleep | EEG of low amplitude and mixed frequencies, low chin electromyogram activity, rapid eye movements | R |
Respiratory events during sleep are defined as follows:
- 1.
Apnea with a decrease in respiratory flow of 90% or more from baseline for at least 10 seconds, whereas a minimum of 90% of the event duration has to meet the criteria of respiratory flow reduction
- 2.
Hypopneas , in which the signal of respiratory flow measurement decreases at least 30%, whereas oxygen saturation (SpO 2 ) decreases by 4% or more compared with pre-event baseline for at least 90% of the duration (minimum of 10 seconds). Hypopneas can also be defined as a decrease in respiratory flow measurement of at least 40% with a decrease in SpO 2 of only 3%.
- 3.
Respiratory event-related arousal is defined as a series of breaths not meeting the criteria for apnea or hypopnea lasting at least 10 seconds characterized by increasing respiratory effort or flattening of the nasal pressure waveform leading to an arousal from sleep.
- 4.
Hypoventilations are defined as an increase of 10% or more in arterial carbon dioxide tension (PaCO 2 ).
Although the AASM 2007 criteria are commonly used in many sleep centers for clinical and research purposes all over the world, questions about whether the new criteria should be used in children and the scoring of some respiratory events are still unanswered.
Sleep and Breathing
Respiratory Regulation During Sleep
Sleep puts breathing at risk. The upper airway dilator muscle activity is decreased during sleep compared with wakefulness, particularly at sleep onset and during REM sleep. Furthermore, the ventilatory response to hypoxia can be impaired, such that critical hypoxia levels can occur during sleep that can be offset only by arousal from sleep.
The major determinant of minute ventilation during wakefulness and sleep is PaCO 2 . In contrast to wakefulness, where PaCO 2 is maintained close to 40 mm Hg, the chemo sensitivity to CO 2 decreases during sleep, as does the chemo sensitivity for oxygen. This leads to PaCO 2 values of 45 mm Hg commonly occurring during stable sleep, while ventilatory demand is decreased and the arousal threshold varies with sleep stages.
Accordingly, the changes in respiratory muscle activity, ventilatory demand, and arousal threshold observed from wakefulness to sleep and across sleep stages challenge ventilatory control and can lead to instability in breathing. A structured approach to evaluating breathing instabilities is the loop gain. This engineering term is used to describe the stability of a feedback-controlled system (in this case, the chemical feedback loop controlling an individual’s ventilation). In the setting of ventilatory control, loop gain reflects the propensity of an individual to develop periodic (unstable) breathing. Patients with a high loop gain caused by a more sensitive respiratory controller (i.e., high controller gain), more effective CO 2 excretion (i.e., high plant gain), or an increased delay because of slowed CO 2 distribution from peripheral tissues to the central chemoreceptors based on, for example, reduced blood circulation (i.e., mixing gain) may be more vulnerable to disturbances of the feedback system, such as slight hypoventilation that occurs as a consequence of decreased upper airway dilator muscle activity during the transition from wakefulness to sleep.
High loop gain may contribute to severity of OSA. Moreover, subjects with a high loop gain have been shown to be more likely to develop breathing instabilities such as Cheyne-Stokes respiration (CSR; see “Central Sleep Apnea”).
Sleep-Disordered Breathing
SDB refers to the respiratory signs and symptoms associated with sleep-associated respiratory dysfunction and is defined by the occurrence of respiratory events, which are cessations in breathing rhythm (apneas) or momentary or sustained reduction in the amplitude of respiratory flow (hypopnea) during the sleeping state, leading to arterial hypoxemia. They are usually caused either by increases in upper-airway resistance due to reduction in intraluminal airway diameter (obstructive event), marked reduction or cessation of brainstem respiratory motor output (central event), or both. A specification of SDB into obstructive or central sleep apneas (CSA) is made based on the predominant type of respiratory events. The severity of SDB is usually quantified by the number of respiratory events per hour of sleep. This is necessary because a low number of short apneas and hypopneas can occur up to about five times per hour in healthy subjects. Two measures can be used for this purpose: the apnea hypopnea index (AHI) and the respiratory disturbance index (RDI). Typically the AHI (number of hypopneas and apneas per hour of sleep; Fig. 10.7 , top ) and RDI (number of hypopneas, apneas, and arousals related to respiratory events per hour of sleep; see Fig. 10.7 , bottom ) are used to quantify the severity of SDB.

Obstructive Sleep Apnea
Definition
OSA is the most common type of SDB and is diagnosed in patients with more than 15 predominantly obstructive events per hour of sleep, or with fewer respiratory events (i.e., 5-15 per hour) if daytime symptoms (i.e., sleepiness) or comorbidities such as hypertension or atrial fibrillation are present. These cutoff values have been used as an indication for treatment by clinicians based on the clinical guidelines and the international classification of sleep disorders. The severity of sleep apnea is further characterized as mild in patients with less than 15 events per hour of sleep, as moderate with 15 to 30 or less events per hour of sleep, and as severe sleep apnea with 30 or more events per hour of sleep ( Table 10.4 ).
RDI (Events Per Hour) | AHI (Events Per Hour) | |
---|---|---|
No sleep apnea | <5 | <5 |
Mild sleep apnea ∗ | ≥5 to <15 | ≥5 to <15 |
Moderate sleep apnea | ≥15 to <30 | ≥15 to <30 |
Severe sleep apnea | ≥30 | ≥30 |
∗ Only diagnosed if comorbidities like hypertension, atrial fibrillation of daytime sleepiness are present.
Epidemiology
OSA with daytime symptoms affects 0.3% to 5% of the general population. As obesity is one of the major risk factors for OSA, the prevalence might continue to increase with higher rates of obesity in the general population. The prevalence of SDB without daytime symptoms is even higher, with rates of up to 9% in women and 24% in men between the ages of 30 and 60 years, and is often unrecognized.
Furthermore, the prevalence of OSA varies widely between different populations. Individuals with a high susceptibility to OSA include the obese, the elderly, and those with specific comorbidities (e.g., stroke, myocardial infarction). Depending on methodology, the rates of OSA in patients following surgery is between 45% and 75%. A recent study in patients undergoing bariatric surgery revealed an incidence of OSA of as high as 77.5% in this population, while another study showed OSA to be prevalent in 50% of women undergoing gynecologic oncology surgery
Clinical Symptoms
Approximately one-third of patients with OSA complain of typical signs and symptoms occurring during wakefulness. Waking with dry mouth or headache in the morning, daytime sleepiness, falling asleep during monotonous situations (e.g., watching television), as well as subjective impairment of cognitive function are frequently reported. This combination of OSA with daytime symptoms is usually referred to as OSA syndrome.
Sleep-associated signs and symptoms of OSA include witnessed pauses in breathing or snoring, and a high number of nocturnal awakenings, mostly reported as pseudo-nocturia. Patients with OSA occasionally complain of tachycardia or respiratory distress upon nocturnal awakening (choking sensation) and other symptoms ( Box 10.1 ).
Nighttime Symptoms
- ▪
Frequent awakening during the night (e.g., pseudo-nocturia)
- ▪
Awaking from own snoring with choking sensation
- ▪
Tachycardia
- ▪
Sleep that is not restorative
Daytime Symptoms
- ▪
Awaking with dry mouth
- ▪
Dull headache in the morning
- ▪
Daytime sleepiness
- ▪
Falling asleep during monotonic situations (e.g., watching television)
- ▪
Subjective impairment of cognitive function
Symptoms Reported by Bed Partner
- ▪
Snoring, especially when loud and arrhythmic
- ▪
Observed pauses in breathing during sleep
Consequences and Comorbidities
OSA is associated with serious medical consequences, such as hypertension, myocardial infarction, stroke, diabetes, diabetic neuropathy, as well as cognitive dysfunction resulting in occupational difficulties and motor vehicle accidents. The development of cognitive impairment in patients with OSA is associated with atrophy of brain structures relevant for cognition and memory (hippocampal areas) that can be partially reversed by adequate treatment. Whether the negative effects of OSA are due to impaired sleep architecture or effects of intermittent hypoxia remain unclear, because data investigating the effect of intermittent hypoxia without other symptoms in OSA patients are limited. Nevertheless, recent findings suggest that even asymptomatic OSA might be associated with cardiovascular effects, such as altered daytime autonomic regulation (i.e., heart rate variability).
Given the frequent rate of complications associated with OSA, it is not surprising that OSA leads to increased socioeconomic costs because of significantly more frequent rates of health-related contacts and medication use, and even higher rates of unemployment.
Risk Factors
Predisposing factors for OSA are obesity, age, male sex, factors leading to swelling of the superficial tissue of the upper airway (smoking), allergic rhinitis, and decreased muscle tone of upper airway dilator muscles (e.g., central nervous system) caused by respiratory depressants.
Pathophysiology
Respiratory events during OSA are characterized by decreased respiratory flow with persisting respiratory effort due to reduced intraluminal diameter of the upper airway up to complete pharyngeal airway collapse (see Fig. 10.6 ). The muscles involved in respiration are morphologically and functionally skeletal muscles and can be classified into two groups: upper airway dilator muscles and respiratory pump muscles.
Upper airway dilator muscles counterbalance the negative inspiratory pressure generated by the pump muscle to permit airflow during inspiration. Respiratory pump muscles are the collection of muscles responsible for generating inspiratory and expiratory forces in the thorax across the breathing cycle ( Fig. 10.8 ). The patency of the upper airway is maintained by balancing dilating forces (generated by the upper airway dilator muscles) and collapsing forces (i.e., negative intraluminal pressure generated by the respiratory pump during inspiration and compressive extraluminal forces from the surrounding tissues).

Upper airway dilator muscles
The most extensively studied of the upper airway dilating muscles are the genioglossus and the tensor palatini ( Table 10.5 ). The genioglossus receives a variety of inputs, including phasic (inspiratory) and tonic (noninspiratory) drives, which are distributed differentially across the hypoglossal motoneurons. In response to negative pharyngeal pressure created by the respiratory pump during inspiration, the genioglossus reflexively stabilizes the upper airway in animals and humans. This reflex is likely a product of signaling from inspiratory modulated motor units. Whereas the genioglossus responds to phasic input on top of its tonic activation, the tensor palatini is considered a tonic muscle with consistent tone throughout the respiratory cycle.
Muscle | Tonic Activity | Phasic Activity | |
---|---|---|---|
Insp. | Exp. | ||
Tensor palatini | + | + | – |
Levator palatine | + | + | – |
Genioglossus | + | + | – |
Geniohyoid | + | – | + |
Thyrohyoid | X | X | X |
Anatomic vulnerability to collapsing forces
The soft tissues of the pharynx are enclosed and stabilized by bony structures, such as the mandible and the spine, and complete collapse of the pharyngeal airway ordinarily requires extraluminal forces, such as hematoma, edema, peripharyngeal masses, or an airway trauma, for instance, as a consequence of prolonged endotracheal intubation. Pharyngeal manifestation of obesity compresses the airway. Craniofacial abnormalities can further increase the collapsing effects of excessive pharyngeal extraluminal soft tissue in obese patients. The extraluminal soft tissue, as well as size and shape of the bony enclosure, are determinants of the extraluminal pressure that need to be antagonized by the upper airway dilator muscle contraction during inspiration, to avoid an upper airway obstruction–related apnea. In addition, the upper airway is more vulnerable to collapse in the supine position than in the lateral or sitting positions, due to gravitational effects.
Excessive intravenous fluid administration can affect upper airway patency. In awake, healthy volunteers, the inflation of antishock trousers displaced fluid from the lower extremities to increase neck circumference, so that the upper airway had a lower threshold to collapse. This concept is reinforced in studies of subjects with lower extremity venous insufficiency and congestive heart failure (CHF). These studies have shown nocturnal redistribution of fluid from the lower extremities into the neck increases upper airway collapsibility and the severity of central apnea and OSA. Similar effects may increase the risk for OSA during pregnancy and early after delivery.
Another important component of airway patency is the interplay between lung volume and upper airway collapsibility. Higher end-expiratory lung volumes are associated with a decrease in upper airway resistance to airflow in awake healthy humans, and an increase in upper airway lumen dimensions in subjects with and without OSA. The mechanism for the interaction between upper airway patency and lung volume is thought to lie in the generation of longitudinal traction forces in the trachea. Upon inspiration, the lung inflates and effectively forces the carina into a more caudal position, creating stretching forces on the fixed trachea. These forces are transferred to the side walls of the upper airway. Effectively, tracheal traction allows the respiratory pump muscles to contribute to upper airway opening.
Collapsing intraluminal pressure
The respiratory pump is the motorized action driving inspiration and expiration. It is the force that broadens the thoracic cavity and creates negative intrathoracic pressure to draw a breath in, and when needed, positive intrathoracic pressure to exhale rapidly. Inspiratory pump muscles are an anatomically diverse group, with the most studied being the intercostal muscles and the diaphragm. The diaphragm accounts for 60% to 70% of lung volume change during quiet respiration. During inspiration, the volume of the thoracic cavity increases as a result of contraction of the diaphragm and the external intercostal muscles. The lungs expand secondarily to the increased negative intrathoracic pressure generated by these phasic actions of the inspiratory pump muscles. The negative intrathoracic pressure translates into a negative intraluminal pressure in the upper airway, forcing the airway to collapse as soon as this pressure drops below a critical value.
Although this critical airway pressure (Pcrit) is typically negative (approximately −5 cm H 2 O) in healthy controls, the upper airway in patients with OSA during sleep may even collapse at a positive Pcrit. Positive intraluminal pressure is required for reopening the paralyzed airway in patients with OSA. Under normal conditions, the two compressing factors, (1) negative intraluminal pressure and (2) positive extraluminal pressure, need to be actively compensated by muscular activity of the upper airway dilator muscles to maintain airway patency.
Wakefulness stimulus and sleep
Excitatory inputs to the upper airway motoneurons (e.g., hypoglossal motoneurons) include those from serotoninergic and noradrenergic neurons that fire predominantly during wakefulness, resulting in a “wakefulness stimulus” that increases the activity of upper airway dilator muscles during this arousal state. With onset of sleep, this arousal-dependent neuronal input (active while awake versus less active, or inactive when asleep) disappears, leading to a decrease in upper airway muscle activity and causing increased upper airway resistance in healthy controls and airway collapse in patients with OSA. The resulting retropalatal obstruction of the upper airway is reported to be the most common pathophysiological mechanism in OSA.
One way valves in the upper airway
Observing the respiratory flow tracings in patients with OSA, one can find some respiratory events that do not show the characteristic progressive flow limitation ultimately resulting in complete upper airway occlusion, typically seen as a result of “collapsible tube pathophysiology” with variable compliance. In these cases, the flow tracings show normal expiratory flow and sudden reduction/cessation before reaching maximum inspiratory flow. This “rapid obstruction” only during the inspiratory phase occurred in approximately 20% to 30% of OSA patients. Recent findings suggest that there are two one-way valves in the pharynx. While the epiglottic valve closes during inspiration, the soft palate may function as a second one-way valve limiting flow during expiration.
Similar to the epiglottis, the soft palate hangs from the hard palate like a peninsula in the oropharynx and might occlude the upper airway during expiration. A recent study found that sudden reduction of expiratory airflow immediately after peak expiratory flow occurs more often in OSA patients under positive pressure ventilation. Similar expiratory flow limitation pattern was also reported in spontaneously breathing OSA patients, suggesting that this phenomenon is not unique to positive pressure ventilation.
These findings may point to a second, slightly different mechanism underlying OSA than the pure collapsible tube pathophysiology in some OSA patients, which should be particularly important during anesthesia. Correct identification of the closure site in each OSA patient will enable the clinician to provide an individualized OSA treatment.
Respiratory arousal
Respiratory arousal is arousal from sleep owing to cumulative and progressive increases in stimuli related to breathing (hypoxia, hypercapnia, and respiratory effort).
Three primary inputs contribute to arousal-related restoration of breathing following an apnea ( Fig. 10.9 ):
- 1.
Peripheral and central chemoreceptors sensitive to partial pressures of oxygen and carbon dioxide
- 2.
Sensors in the upper airway responsive to negative pressure generated by the respiratory pump
- 3.
Cortical inputs directly related to state of consciousness or wakefulness

Any of these inputs can restore respiratory muscle tone if the magnitude of stimulation is sufficient. Cortical awakening from sleep, identified by EEG signs of wakefulness, is an adequate stimulus for ventilation. However, obstructive apneas, such as upper airway collapse in OSA, can be terminated by an increased drive to the respiratory muscles not involving cortical arousal. For example, hypercarbia resulting from sustained hypopnea and elevated upper airway negative pressure can independently restore tone to the respiratory muscles. The level of drive provided to the respiratory muscles depends on the summation of stimuli in the central respiratory pattern generator output, including peripheral and central chemo-responsiveness, reflex responsiveness to the negative airway pressure, and strength of the wakefulness drive.
Treatment
Adequate treatment of OSA improves nocturnal oxygen saturation as well as duration and quality of sleep, which translates to reduced daytime sleepiness, improvement of daytime functions, and improved quality of life. Successful treatment of OSA reduces cardiovascular risk, improves insulin sensitivity, and increases neurobehavioral performance. Therefore, all patients with a diagnosis of OSA should be offered treatment as soon as the diagnosis of OSA has been established and its severity has been determined by objective testing (i.e., PSG). Although different treatment options for OSA have been developed during the last decades ( Table 10.6 ), continuous positive airway pressure (CPAP) dose dependently increases upper airway diameter and is still the most effective treatment for OSA of all severities. However, a recent study has questioned if CPAP treatment is able to prevent the long-term adverse cardiovascular events in OSA.
Treatment | Procedure/Device | Recommendation | References |
---|---|---|---|
Weight reduction | Reduction of body weight Weight-loss surgery (improves success of weight loss) | Medium to high, SU | |
Medication | Drug-based treatment (e.g., tricyclic antidepressants, serotonin reuptake inhibitors, cholinergic agonists, carbonic anhydrase inhibitors) | NR, ID | |
Surgical | Nasal surgery | Low, SU | |
Palatal surgery and implants | Low, MC | ||
Tongue base surgery | Low, MC | ||
Increase of muscle activity | Muscle training | ID | |
Hypoglossal nerve stimulation | ID | ||
Nonsurgical | Oral appliance Positive airway pressure | High, AT High, GS |
Positive airway pressure treatment
CPAP as a treatment for SDB is typically applied continuously with a nasal or oronasal mask, and can dose dependently reverse any sleep-associated upper airway obstruction, as outlined in Fig. 10.10 . The CPAP level needed for treatment of OSA varies mostly between 5 and 20 cm H 2 O. However, home RP and titration of CPAP might be similarly effective in some but not all patients with suspected OSA. After titration, the prescribed treatment pressure is applied continuously throughout the night. Although this treatment is efficient in reversing the underlying pathology, the impact of CPAP on outcome is limited by patient adherence, mostly because of local side-effects at the nose or face, or discomfort caused by the mask. When applied with high pressure, the amount of airflow can prevent the patient from falling asleep while using the treatment device. Some treatment devices offer a ramp or delay function that gradually increases treatment pressure from a low starting pressure to the prescribed pressure over a period of 5 to 45 minutes, allowing the patient to fall asleep more easily. Some patients report difficulties exhaling against high CPAP. To avoid this problem, bilevel treatment with reduced expiratory positive airway pressure and a sufficiently high inspiratory positive airway pressure can be used.

In some cases, one CPAP pressure level is not sufficient to treat sleep apnea. CPAP devices with dynamic pressure levels can improve treatment success, particularly in patients with a variable severity of sleep disordered breathing during different sleep stages. These automatic positive-airway pressure or auto-titrating devices measure different variables associated with hypopnea, such as oropharyngeal wall vibration, snoring, and inspiratory flow limitation, and increase the airway pressure until these signs and symptoms of hypopnea disappear. In addition, it is not entirely clear whether CPAP treatment is able to prevent the increased cardiovascular risk inherited by OSA.
CPAP is sufficient in the treatment of most patients with OSA. However, some patients may need different treatment methods. For example, patients with mixed apneas (obstructive and central) or predominantly central apneas need a more controlled (frequency or time controlled), noninvasive ventilation (NIV) with predefined minimal respiratory frequency or respiratory timing that automatically induces the next inspiration (ventilation) if the patient does not induce the next breath within preset parameters.
Alternative treatment options
Oral appliances (OAs) are possible treatment options in patients with mild to moderate OSA who do not tolerate CPAP therapy. Two major designs are currently used clinically: (1) mandibular repositioning appliance, which holds the mandible in an advanced position (a protrusion of at least 50% of the maximal possible extension is recommended for effective treatment), and (2) tongue-retaining devices, leading to repositioning of the tongue into a forward position without any protrusion of the mandible. A multidisciplinary approach including a sleep physician and a dental technician experienced in OAs has been recommended for this purpose, because both factors are crucial for patient adherence and outcome from OA treatment. OAs are recommended in patients with mild to moderate OSA who do not tolerate, do not respond to, fail, or are not appropriate candidates for CPAP treatment.
Historically, surgical treatment methods were the only approach to treatment of OSA, but the effectiveness of most of the nasopharyngeal surgical approaches for severe OSA remains unclear. However, tonsillectomy is beneficial in adult patients with OSA from tonsillar hypertrophy, and adenotonsillectomy is recommended in children with OSA and adenotonsillar hypertrophy. Repeated sleep testing before and following surgery is recommended to ensure sufficient long-lasting therapeutic effects.
Electrical stimulation of the upper airway muscles has been explored recently as a new approach to treatment of OSA. Hypoglossal nerve stimulation induces genioglossus muscle contractions, which dose dependently increase inspiratory airflow during OSA, but the invasiveness of the procedure, the cost of the device, and the requirement for preimplantation endoscopy during anesthesia limit its use as a first-line treatment of OSA.
While pharyngeal fat deposits led to a decrease in pharyngeal patency, underlining the risk factor of obesity, weight loss leads to a reduction in Pcrit and the severity of OSA and is recommended as adjunctive treatment in all overweight patients with OSA. Because long-term weight reduction is more effective when accompanied by weight loss surgery, bariatric surgery may be considered as an adjunctive therapy in very obese patients (body mass index [BMI] ≥ 40 kg/m 2 ), as well as in those with important comorbidities and BMI of 35 kg/m 2 or greater and in whom dietary attempts at weight control have been ineffective.
Oxygen is currently not recommended as primary treatment of OSA, but may be used as an adjunctive treatment in some patients, particularly in the postoperative period.
Although OSA is more common in the older population, it occurs in children with a peak in the incidence between 2 and 5 years of age. Obesity predicts snoring and other signs of obstructive respiratory events in children. Tonsillar and adenoidal hypertrophy is another important cause of OSA in children and can be treated surgically.
Central Sleep Apnea
CSA impairs quality of life and is associated with adverse outcome in heart failure patients. CSA is defined as cessation of air flow without respiratory effort, which separates it from OSA, where respiratory effort is maintained or even increased during an apnea. In clinical sleep apnea, considerable overlap between OSA and CSA occur that need to be identified and subsequently treated.
CSA can be found in older patients and in patients with severe comorbidities such as CHF, stroke, or other neurologic disorders (e.g., amyotrophic lateral sclerosis). In a southern Pennsylvania cohort, CSA (AHI ≥ 20/hour) was found in 5% of men aged 65 years and older, but it was not found in younger men or in women of any age. For an AHI of 2.5 per hour and greater, the prevalence estimates in men younger than 45 years remains negligible, while CSA was found in 1.7% of men between 45 and 64 years, and 12% in men older than 65 years, or 9% in individuals between 40 and 97 years, respectively.
CSA mechanisms can be categorized into those with high and low loop gain. The most common subtype of CSA with an increased loop gain is CSR and is commonly seen in patients with CHF and left-ventricular systolic dysfunction. CSR is defined as a crescendo-decrescendo pattern of hyperventilation between 20 and 30 seconds in duration, followed by 10 to 40 seconds of hypopneas or apneas ( Fig. 10.11 ), usually occurring during NREM sleep stage 1 and 2. Nevertheless, CSR can also occur during exercise or wakefulness. Nearly one out of two patients with CHF shows CSR. CSR is more common in men and worse in the supine body position.

Potential respiratory therapies of CSR include oxygen, respiratory stimulants (e.g., CO 2 , theophylline, and acetazolamide), and NIV such as bilevel positive airway pressure. The effectiveness of CPAP as therapy is controversial. Optimization of medical therapy is the best treatment, because CSR will often resolve with adequate treatment of CHF (cardiac resynchronization therapy and surgical treatment, such as heart transplant).
Other Forms of Central Breathing Disorders
The term periodic breathing refers to altitude-induced breathing instability that occurs in subjects transferred to a high altitude, where ambient hypoxia caused by low barometric pressure leads to an increase in controller gain. Idiopathic CSA, which is a relatively uncommon disorder at sea level, occurs more likely in individuals with an elevated hypercapnic ventilatory response (high controller gain) that leads to hypocapnia and respiratory control instability during sleep. Patients with idiopathic CSA tend to have low PaCO 2 levels, even during wakefulness.
Obesity Hypoventilation Syndrome
Alveolar hypoventilation is defined as insufficient ventilation leading to hypercapnia (increased PaCO 2 ). Mechanisms of alveolar hypoventilation include central hypoventilation, chest wall deformities, neuromuscular disorders, chronic obstructive pulmonary disease, as well as severe obesity (obesity hypoventilation syndrome [OHS]). OHS is defined as the combination of nocturnal and daytime hypoventilation, usually leading to hypercapnia in obese subjects (BMI ≥ 30 kg/m 2 ), in the absence of other causes of hypoventilation.
The prevalence of OHS is estimated to be up to 50% in obese patients with OSA. It can occur in up to 50% of patients with a BMI of 50 kg/m 2 or more, compared with 0.15% to 0.3% in the general adult population. Ninety percent of patients with OHS also suffer from OSA. OHS often remains undiagnosed, and the true prevalence remains unclear.
Severe obesity is associated with an increase in respiratory drive that helps maintain eucapnia in the presence of the abnormal chest wall mechanics and high work of breathing. In OHS, this compensatory mechanism is abolished, which in part might be explained by leptin resistance. Typically, OHS manifests as a reduced lung capacity, vital and functional residual capacity, expiratory reserve volume, respiratory system compliance, and inspiratory muscle strength, whereas response to CO 2 might be reduced or normal. In addition, there is an increase in serum bicarbonate and alveolar PCO 2 , as well as in the work of breathing and leptin levels.
Impairment of the effectiveness of respiratory pump muscle function in patients with OHS can be explained by the effects of low lung volume because of central fat distribution, leading to a cranial displacement of the diaphragm when lying supine. In addition, diaphragmatic myopathy might be a contributing factor of OHS. Treatment options include weight loss and NIV.
Sleep and Anesthesia: Two Unequal Twins Influencing Perioperative Medicine
Clinical Picture of Sleep and Anesthesia
Although physiologic sleep and anesthesia share some clinical features (loss of consciousness and a modulation of brainstem autonomic function), major differences can be found when closer observations of both behavioral states are undertaken. In contrast to anesthesia, sleep shows spontaneous generation and termination, ready reversibility by noxious stimuli, and homeostatic regulation. Anesthesia does not share the stage-wise structure seen during physiologic sleep. In addition, functional imaging studies underline the fundamental differences during onset of anesthesia and wakefulness-sleep transition.
Although there are similarities in the EEG patterns between slow-wave sleep and anesthesia-induced unconsciousness, the EEG patterns during sleep and anesthesia are different, with different frequencies and types of activation in EEG recorded during physiologic sleep and the uniform EEG picture observed during anesthesia-induced unconsciousness ( Fig. 10.12 ).

During anesthesia induction, the level of consciousness continuously decreases from fully awake through states of reduced response to external stimuli up to a completely unresponsive state. This is in contrast to the sharp α–θ EEG transitions seen while an individual proceeds from wakefulness to sleep without any transient stages. Individuals in a steady state of physiologic sleep can be aroused with sufficient stimulation, whereas drug-induced unconsciousness needs at least some drug elimination to occur before individuals can be aroused.
Activation of Sleep-Promoting Pathways During Anesthesia
The role of the endogenous sleep-promoting system in the mechanism of action of general anesthetics has recently received much attention. This hypothesis is attractive because of the similarities of sleep and anesthesia and some evidence that anesthetically induced sleep could meet some of the homeostatic need for sleep. It is even more attractive because, despite the large database of the molecular effects of anesthetics, how loss of consciousness occurs is still not explained. An important discovery is that some of the neurons that are active during sleep in the VLPO are also activated by certain anesthetics. A second key observation is the inhibition of the arousal nuclei, the TMN, contributes to anesthesia. This effect also implicates activation of VLPO because it provides the major source of inhibition of the TMN. The idea has emerged that anesthesia-induced loss of consciousness is mediated by effects of the anesthetics on the flip-flop switch centered in the VLPO that is responsible for the sharp transition from wakefulness to sleep and back (see earlier section). However, there are a number of problems with this theory, including that rats and mice with complete lesions of the VLPO can still be anesthetized. Although lesions of VLPO lead to a transient resistance to volatile anesthetics, if some time elapses following a VLPO lesion, the animals show increased sensitivity to isoflurane anesthesia attributable to increased homeostatic sleep drive. Another problem with the VLPO-TMN circuit hypothesis of anesthesia is that direct inhibition of the TMN produces sedation but not anesthesia. Animals without VLPO neurons have profound insomnia. Therefore, interaction of anesthetics with these sleep-promoting nuclei alone is not sufficient to produce unconsciousness during anesthesia. During recent years, several studies provided evidence to extend the scientific framework of general anesthetics modulating sleep-promoting regions by the anesthetic depression of arousal-promoting nuclei, including the LC, GABAergic neurons of the pontine reticular formation, the pedunculopontine and laterodorsal tegmentum, the ventral tegmental area, as well as the perifornical area, the tuberomammillary nucleus, and the basal forebrain. This “bottom-up” hypothesis of anesthesia-induced unconsciousness may be the result of the simultaneous interaction with the brainstem and diencephalic regions, including the AAS and endogenous sleep circuitry. Although, these mechanisms show similarities with sleep-promoting neuronal interactions, they are unlikely to be the only correlate of anesthesia-induced unconsciousness. Sleep, but not anesthesia, is easily reversed or prevented by real or perceived environmental threats. While interaction with sleep-promoting neurons work by inhibiting these arousal pathways, other neuronal networks crucial for consciousness (e.g., thalamocortical networks) remain active during sleep. However, the finding that many anesthetics engage sleep pathways is still important and meaningful, as this is undoubtedly an important mechanism by which these classes of anesthetics promote sleep. Nevertheless, this effect does not and cannot prevent arousal to sufficient external stimuli. The unique property of anesthesia to do so has to be mediated by an additional mechanism.
Perioperative Interactions Between Anesthesia and Sleep
Anesthesia and painful surgical procedures affect sleep and circadian rhythms for as long as 6 months, depending on the complexity of the procedure performed. A substantial decrease in REM sleep occurs on the first night after surgery and anesthesia, followed by a profound REM rebound phenomenon on the second to fourth postoperative nights, when REM sleep increases in both intensity and amount. Although most anesthetics can lead to an impairment of sleep architecture, such as REM depression and reduced sleep quality during the early postoperative period, the extent of this impairment probably depends on the pharmacokinetics and pharmacodynamics of the anesthetics and opioids used, which affect the duration of REM sleep, as well as surgery-induced stress.
The effect of propofol on sleep architecture and REM sleep is complex and dose dependent. In long-term ventilated, critically ill patients, propofol sedation abolishes REM sleep and diminishes sleep quality, whereas with low-dose propofol, REM sleep is possible. The effects of ketamine sedation on sleep architecture have not been studied in detail, although it may have rather mild effects on REM duration.
Anesthetics have strong effects on GABA and NMDA receptors, both of which are linked with circadian control. As a result, anesthetics may interfere with entrainment of the circadian rhythm; however, preclinical and human data show inconsistent results. A recent trial in honeybees suggested an anesthetically induced circadian shift. Inside the laboratory with no outdoor cues, daytime anesthesia disturbed the usual activity patterns in the hive for the next several days. In addition, cycles of clock gene activity showed a delay, and inhibition of the period circadian protein (per)-2 gene expression (one of the core clock genes) was observed during anesthesia. Of note, psychotropic nonanesthetics, such as opioids, have been shown to affect melatonin secretion indirectly, independent of the behavioral state anesthesia in a pig model. Previous work in humans has shown that 3 hours of anesthetic exposure does not affect the circadian phase of the body temperature rhythm.
In summary, the specific mechanisms of anesthetics effects on circadian rhythm are unclear. The ability to distinguish between effects occurring directly on the circadian pacemaker and those occurring downstream from the pacemaker on other physiologic control systems requires additional research under rigorous experimental conditions.
Surgical treatment itself impairs sleep, expressed as reduced REM sleep duration even in the absence of general anesthesia. Pain, inflammation, stress immobility, and anxiety seem to be contributing factors. After surgery, patients show a significant impairment of sleep quality and duration. Eventually, it will be known whether specific anesthetics are preferable to avoid the adverse effects of sleep deprivation.
Sleep-Disordered Breathing and Airway Patency During Anesthesia
Perioperative complications occur more often in patients with OSA than in healthy controls, but the reasons for this observation are unclear. It is challenging to isolate the effect of OSA from the known adverse effects on perioperative outcome of typical OSA comorbidities, such as hypertension, diabetes, coronary artery disease, neurovascular vulnerability, and obesity. Fortunately, severe postoperative complications are uncommon. As a consequence, large trials are needed to isolate the causal relationship between SDB and perioperative complications, such as severe respiratory failure, thromboembolic complications, increased hospital length of stay, and mortality.
Independent of obesity, OSA is not associated with difficult tracheal intubation or mask ventilation. However, recent metaanalyses reported a significantly higher risk of postoperative major cardiac and cerebrovascular events, newly detected postoperative atrial fibrillation and acute postoperative respiratory failure, compared with control subjects. These findings need to be confirmed in future prospective studies with a larger sample size and a more homogenous population such that confounding factors can be controlled. OSA is associated with postoperative delirium, which is an important perioperative complication associated with increased costs, morbidity, and mortality. However, it is currently unclear if this is due to repetitive hypoxia or sleep fragmentation. In contrast, a recent study using the Nationwide Inpatient Sample database analyzed data from 1,058,710 patients undergoing elective surgery. These authors showed that patients previously diagnosed with SDB had significantly higher rates of emergent mechanical ventilation, use of NIV, and CPAP, as well as increased rates of respiratory failure. Nevertheless, the study also showed that patients with SDB had better outcomes after emergent intubation and had lower care-associated costs compared with their non-SDB counterparts. Similar findings were reported by the same group for data from 91,028 patients undergoing bariatric surgery. In this study, patients with a previous diagnosis of SDB had a shorter hospital length of stay and lower total costs compared with non-SDB patients. The reasons for the differences are unclear. Patients with a known history of sleep apnea may receive more sophisticated perioperative monitoring and more aggressive treatment of their respiratory disease, which might also explain the higher rate of postoperative reintubation in SDB patients reported in the same group. Another possible explanation relates to the consequences of chronic nocturnal desaturation, which can have preventive effects in terms of the consequences of perioperative acute hypoxia. Most importantly, these findings indicate that the association of SDB, and perioperative outcome is likely to be more than a unidirectional relationship. More likely is that SDB could have a dual effect, leading to increased rates of perioperative respiratory complications in patients with SDB, but additionally protecting the same population from the fatal consequences of these complications. The mechanism of the potential link between OSA and postoperative respiratory failure, as well as postoperative delirium, is probably multifactorial. Frequent episodes of airway collapse in OSA lead to hypoxia, disrupted sleep, daytime sleepiness, and increased arousal threshold from sleep, all of which can be considered as potential precipitating or augmenting factors of postoperative complications. It is intriguing to note that patients with OSA appear to be most vulnerable to hypoxia on postoperative night two or three, the time interval during which postoperative delirium most typically manifests.
One population that might deserve additional attention in perioperative care is patients with OHS who, compared with obese patients, are more likely to be admitted to a hospital and require more healthcare resources. In addition, higher rates of ICU admission, lengthier long-term care requirements at discharge, and higher mechanical ventilation rates have been reported in patients with OHS compared with severe obese patients without OHS.
In summary, patients with SDB are more vulnerable to developing severe perioperative complications. Nevertheless, it is currently unclear whether these complications translate to a poorer overall postoperative outcome for these patients.
Perioperative Management in Patients with Sleep-Disordered Breathing
The standard of care for perioperative management of patients with SDB depends on the severity of the disease, comorbidities, and the threat associated with the planned surgical procedure. It is probably neither feasible nor necessary to conduct a sleep study in all obese patients at risk for sleep apnea who are scheduled for surgery; however, high-risk patients need to be identified and treated perioperatively. Fig. 10.13 shows an algorithm that is being developed by an interdisciplinary international group of clinicians. The algorithm is driven by the idea that patients who have been prescribed CPAP preoperatively should continue to use CPAP perioperatively. Patients who carry several risk factors (procedural and comorbidity-based) may need a preoperative sleep medicine consultation and/or a CPAP prescription.

Preoperative Screening
Several strategies need to be considered to identify patients with SDB adequately. Although clinical examination is the easiest and most cost-effective method of assessment using predisposing physical characteristics, it has a poor sensitivity and specificity of only 50% to 60% to diagnose OSA accurately. However, the clinical value of using questionnaires relates to the fact that they help to identify a high-risk perioperative population, independently of the question of whether an individual patient has OSA. For example, the STOP-Bang questionnaire basically screens for comorbidities of OSA that we know increase the risk of perioperative complications (hypertension, obesity, male sex, older age) rather than direct breathing disorder–associated characteristics. The Score for Prediction of Obstructive Sleep Apnea might also be a helpful tool to predict patients with OSA and its perioperative outcome based on comorbidities and easily available clinical data.
PSG is required to identify SDB specifically, but it cannot be used for preoperative screening of surgical patients. PSG is expensive, may delay surgery, and is inconvenient for the patient. A multistep approach of preoperative screening is required. Preoperative assessment should include a screening for SDB and for the current use of NIV. OSA might not represent real risk in patients scheduled for low-risk procedures from a surgery and anesthesia point of view. The authors believe that patients with no previously diagnosed SDB undergoing a low-risk surgical procedure should receive standard perioperative monitoring and treatment.
Patients undergoing high-risk surgical procedures should receive further workup using a clinical examination combined with standardized and validated questionnaires (e.g., Berlin). Although this diagnostic algorithm might be a sufficient screening for OSA, it might be insufficient to detect OHS. A blood gas analysis should be considered as a method to detect hypercapnia as a major symptom of OHS (criterion: awake daytime hypercapnia PaCO 2 ≥ 45 mm Hg). Alternatively, a venous bicarbonate concentration ≥ 27 mmol/L is highly sensitive (92%) for an increased PaCO 2 , which combined with hypoxemia (SpO 2 ≤ 94%) indicates a high risk of OHS in these patients. Of note, OHS is a diagnosis by exclusion. Severe obstructive airway diseases, severe interstitial lung disease, severe chest wall disorders (e.g., kyphoscoliosis), severe hypothyroidism, neuromuscular disease, and congenital central hypoventilation syndrome need to be excluded, and further evaluation of sleep by a sleep medicine specialist before anesthesia should be considered. In these cases, the sleep specialist should determine the optimal diagnostic instruments in cooperation with the perioperative team, prescribe the perioperative auto-titrating CPAP device, and offer in collaboration with respiratory therapy specialists to improve tolerability of perioperative CPAP therapy.
Perioperative Management of Patients with Possible Sleep-Disordered Breathing
Although the data on optimal intraoperative and perioperative management are still limited, and mainly based on studies with low sample size, the techniques and procedures summarized in Box 10.2 might be considered during the perioperative management of patients with SDB. Patients with a history of SDB who are undergoing surgery and anesthesia with high risk of morbidity should receive an “OSA anesthesia bundle” (see Box 10.2 ) that includes special procedures and preparations during tracheal intubation, extubation, pain therapy, and perioperative CPAP therapy.
Preanesthesia Period
- ▪
Consider regional anesthetic techniques that minimize the chance of postoperative sedation.
Induction Strategy
- ▪
Monitoring: capnogram, tidal volume measurement
- ▪
Sniffing position
- ▪
Reverse Trendelenburg position
- ▪
Consider intubation without nondepolarizing NMBA; consider succinylcholine.
- ▪
Triple airway maneuver with two hands
- ▪
Utilize lung recruitment maneuvers immediately after intubation and apply PEEP for maintaining lung volume during surgery.
- ▪
PCV with PEEP
- ▪
Short-acting anesthetics and narcotics preferred
- ▪
Avoid high-dose steroidal NMBA.
- ▪
Use neuromuscular transmission monitoring.
Intraoperative Management
- ▪
Whenever possible, use of sedatives and narcotics should be reduced.
- ▪
Agents with reduced impairing effect on upper airway patency might be considered (e.g., ketamine, pentobarbital).
- ▪
Neuromuscular blockade should be monitored.
- ▪
Residual neuromuscular blockade should be reversed.
Extubation and Postanesthetic Care Unit
- ▪
Patient should be able to cooperate before extubation. Consider positioning of patients in PACU bed: upper body should be elevated by 45 degrees; lateral position preferred to minimize gravitational effects on the upper airway.
- ▪
In case of impaired respiratory function, a plan needs to be defined and documented for monitoring and treatment, including the consideration of noninvasive ventilation.
- ▪
Patients will be discharged to an unmonitored environment or home when they meet discharge criteria:
- ▪
Vital signs within 20% from baseline
- ▪
Adequate treatment of nausea
- ▪
Pain score ≤40%
- ▪
Aldrete-score ≥8
- ▪
Passed room air challenge test
- ▪
Pain Therapy
- ▪
Consider nonsteroidal antiinflammatory drugs to reduce opioid use whenever possible, if not contraindicated.
- ▪
Use caution when combining opioids with sedatives or hypnotics.
Tracheal Intubation
Patients with OSA are frequently obese, and obesity is a risk factor for difficult intubation. Patients should be preoxygenated efficiently before injection of the anesthetic drug. Sniffing and reverse Trendelenburg position are preferred before intubation, which improves maintenance of the passive (i.e., paralyzed) pharyngeal airway in patients with OSA and increases functional residual capacity. To reduce the duration of paralysis, nondepolarizing neuromuscular blocking drugs should be used with caution supported by quantitative neuromuscular transmission monitoring. Immediately after successful intubation, a lung recruitment maneuver and the application of positive end-expiratory pressure for maintaining lung volume during surgery should be considered.
Intraoperative Treatment
Impaired upper airway patency is of special concern whenever anesthetics are administered without a device that bypasses the collapsible upper airway—for example, during sedation for endoscopy. While the overall rate of overt sedation-related complications for such procedures (e.g., endoscopy) appears low, patients who are vulnerable to respiratory complications may suffer from sequelae of increased upper airway collapsibility beyond the immediately observable after intervention.
Opioids and GABAergic sedatives/hypnotics impair upper airway patency without any meaningful difference between compounds given at an equipotent dose of analgesics. Of note, the impairing effects of GABAergic drugs on airway patency can be reversed by the respiratory stimulants ( Fig. 10.14 ). Thus it is recommended not to extubate patients at risk of postoperative airway collapse during hypocarbia but rather at mildly hypercarbic conditions.

Ketamine abolishes the impairment of upper airway patency during loss of consciousness and sleep, and may be a viable adjunct to achieve postoperative pain therapy in patients at high risk of airway collapse.
Nondepolarizing neuromuscular blocking drugs should be titrated optimally by quantitative neuromuscular transmission monitoring to avoid residual neuromuscular blockade, which increases the risk of postoperative respiratory complications. The effects of neuromuscular blockade should be reversed only when present, because inappropriate reversal can impair upper airway function in animals and humans.
Postoperative Care
OSA occurs during sleep and sedation; therefore, tracheal extubation should be delayed until complete recovery of consciousness. Patients should be positioned with the upper body elevated by 45 degrees, which improves airway patency and functional residual capacity. The lateral body position is an alternative for patients who do not tolerate an elevated upper body position. NIV is a viable treatment of postoperative respiratory failure and helps prevent deoxygenation and the development of postoperative negative pressure pulmonary edema; it can also prevent the respiratory depressant effects of opioids.
Before discharge from the post anesthesia care unit, a room air-challenge test should be conducted. Several noninvasive monitoring devices have been developed for patients with and without risk for OSA. However, it is currently unclear if these devices can help prevent postoperative complications, and this requires further investigation.
Pain Therapy
Pain treatment is of special concern during the postoperative period. Opioids dose-dependently reduce respiratory drive, and special caution is recommended when opioids are combined with sedatives or hypnotics. If not contraindicated, regional anesthesia and nonopioid analgesics should be considered to reduce opioid use whenever possible. If opioids are used, CPAP treatment throughout the early postoperative period may be beneficial in patients with high risk for OSA. Pain intensity typically decreases over time following surgery. Decreasing opioid doses are typically required to maintain the balance of decreasing and increasing effects on respiratory drive.
Patients with SDB being treated with CPAP at home should proceed with these treatments throughout the perioperative period. Sufficient functioning of the treatment device needs to be checked preoperatively (see Box 10.2 ).
Knowledge Gaps and Future Research
Although a bold body of evidence on the effect of sleep-disordered breathing throughout the perioperative period has been published during the last decades, there are still large gaps of knowledge. Respiratory, cardiovascular, and neuropsychological complications, as well as unfavorable clinical outcomes, may be more or less common in patients with OSA. However, there are many comorbidities that are potential confounders, such as obesity, diabetes, dyslipidemia, coronary artery disease, and increasing age. OSA is a heterogeneous disease, and detailed physiologic phenotyping could identify pathophysiologic mechanisms that increase or decrease risk of perioperative complications of OSA. Multiple mechanistic pathways that can lead to OSA (e.g., compromised anatomy, dilator muscle dysfunction, low arousal threshold, elevated loop gain, inadequate lung volume tethering, and vascular leak) are potentially relevant in a perioperative setting. In-depth physiological studies are required until phenotyping can be accomplished with less time-intensive studies or through PSG. Noninvasive and readily available physiological studies or clinical prediction models that can characterize patients who have high-risk phenotypes of OSA are an important area of future research.
In this context, adequate screening tools for OSA during the perioperative period need to be identified. As stated previously, PSG is often challenging to schedule before surgery, and ambulatory technologies may be more useful and should be studied. It might even be more important to identify patients at increased risk of postoperative complications due to specific type of surgery, required mode and depth of anesthesia and opioids, as well as other comorbidities, rather than the presence of SDB per se. It is currently unclear to which extent the risk for perioperative complications associated with SDB are due to or modulated by these coexisting factors. In addition, more severe OSA may be developed by some patients with no or mild OSA prior to anesthesia. Challenging factors are opioids, body position, or intravenous fluids. Some studies found early postoperative monitoring to detect patients with high risk for such aggravation of OSA severity. Additional research is needed to determine if and how postoperative monitoring is able to help stratify the risk for postoperative OSA. During the last few years, different approaches were made to improve postoperative management of patients with OSA (as discussed previously). However, open questions remain: What is the role of supplemental oxygen postoperatively? What are the barriers to CPAP adherence in the perioperative setting? Can patient adherence and positive airway pressure effectiveness be increased with educational resources? Should other respiratory support interventions be examined either in isolation or bundled with positive airway pressure? Is there a role for targeted positive airway pressure therapy, even with CPAP naive patients in very high risk groups? What are the medicolegal liability and patient safety factors to consider when starting CPAP in the hospital?
Future research should aim to answer these questions to further improve the perioperative management of patients with sleep disordered breathing. Testing a bundled approach to care (e.g., an algorithm of care including monitoring, positive airway pressure, education, and other respiratory supports) rather than each component individually might be more useful in initial clinical trials, as this may be more likely to be effective in improving outcomes.
The incidence of perioperative complications is low, and collaborative research networks are needed to identify a sufficient number of patients with OSA and answer the important research questions on perioperative outcomes. Patients with OHS might be at high risk. A minimal set of data elements required in prospective cohort studies should be identified to facilitate multisite collaboration and metaanalysis of independent studies. Case-control studies might also be nested within larger cohort studies. This would facilitate the choice of an appropriate control group. These studies may improve risk stratification and lead to novel targeted therapies. In addition, the identification of interventions that can optimize safety in this population is also important. Initial intervention trials should focus on patients at highest risk for sleep disordered breathing.
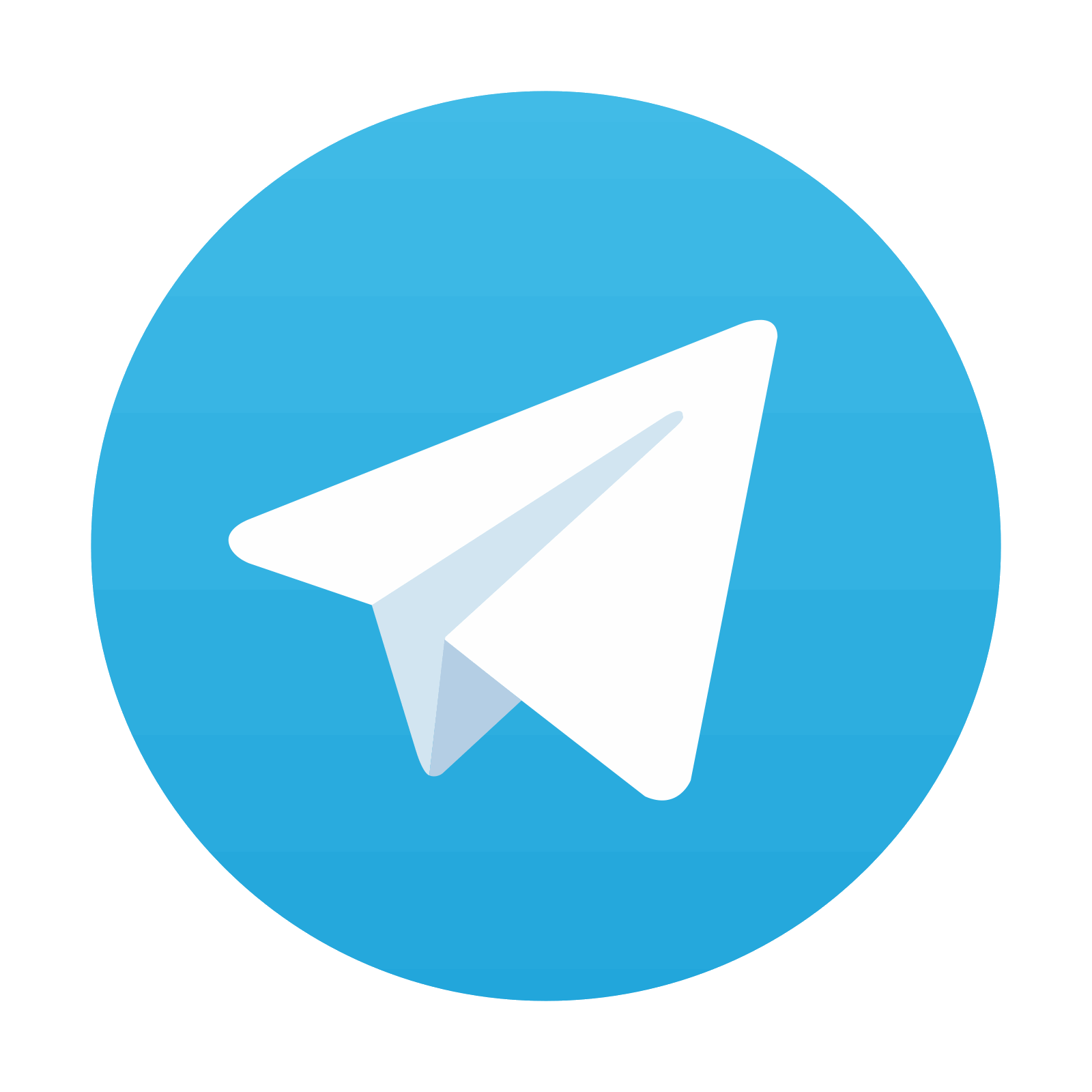
Stay updated, free articles. Join our Telegram channel
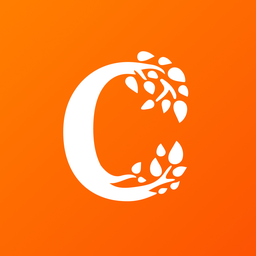
Full access? Get Clinical Tree
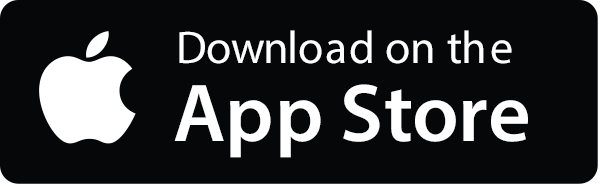
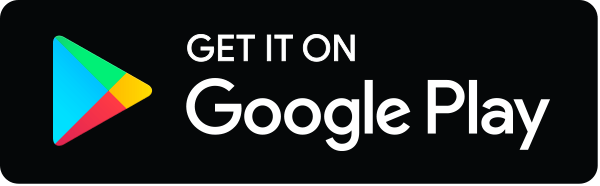