Key Points
- ▪
Removal of carbon dioxide (CO 2 ) is determined by alveolar ventilation, not by total (minute) ventilation.
- ▪
Dead space ventilation can be dramatically increased in patients with chronic obstructive pulmonary disease and pulmonary embolism to more than 80% of minute ventilation.
- ▪
Breathing at small lung volumes increases airway resistance and promotes closure of airways.
- ▪
Hypoxemia can be caused by alveolar hypoventilation, diffusion impairment, ventilation-perfusion mismatch, and right-to-left shunt.
- ▪
Almost all anesthetics reduce skeletal muscle tone, which decreases functional residual capacity (FRC) to levels close to the awake residual volume.
- ▪
Atelectasis during anesthesia is caused by decreased FRC and the use of high inspired oxygen concentrations (FiO 2 ), including breathing oxygen before induction of anesthesia.
- ▪
General anesthesia causes ventilation-perfusion mismatch (airway closure) and shunts (atelectasis).
- ▪
Venous admixture is due to <SPAN role=presentation tabIndex=0 id=MathJax-Element-1-Frame class=MathJax style="POSITION: relative" data-mathml='V˙A/Q˙’>V˙A/Q˙V˙A/Q˙
V ˙ A / Q ˙
mismatch (response to increased FiO 2 ) and shunts (unresponsive to increased FiO 2 ).
- ▪
Hypoxic pulmonary vasoconstriction is blunted by most anesthetics, and this results in increased ventilation-perfusion mismatching.
- ▪
Respiratory work is increased during anesthesia as a consequence of reduced respiratory compliance and increased airway resistance.
Respiratory Physiology Is Central to the Practice of Anesthesia
Respiratory function is inextricably linked to the practice of anesthesia. Adverse respiratory effects can occur during anesthesia, and the most serious cases involve hypoxemia. These events range from intractable hypoxemia caused by loss of airway patency to postoperative respiratory depression from opioids or regional anesthesia. In the absence of adverse outcomes, general anesthesia still has significant effects on respiratory function and lung physiology, documented by observations made in the operating and recovery rooms. Improved appreciation of anesthesia-induced physiologic alterations (e.g., mechanisms of bronchospasm, impact of mechanical ventilation), as well as pioneering developments in monitoring (e.g., pulse oximetry and capnography), together are associated with the specialty of anesthesiology’s emergence as a leader in patient safety. Finally, integrative measures of respiratory function, ranging from exercise capacity, spirometry to tissue oxygenation, to global O 2 consumption, may help predict outcomes following anesthesia and surgery.
Pulmonary Physiology in Health
The mechanisms by which anesthesia-associated respiratory dysfunction is caused can be determined with an examination of normal functions and mechanisms of respiration in health. We briefly review cellular respiration, whereby O 2 is consumed and CO 2 is produced, the transport of O 2 and CO 2 in the blood, and the principles by which the lung oxygenates blood and eliminates CO 2 .
Respiration in the Cell
The partial pressure of oxygen (PaO 2 ) in normal arterial blood is approximately 100 mm Hg, and decreases to 4 to 22 mm Hg in the mitochondrion, where it is consumed. Glucose (C 6 H 12 O 6 ) is converted into pyruvate (CH 3 COCOO − ) and H + by glycolysis in the cytoplasm, and the pyruvate diffuses into the mitochondria and forms the initial substrate for Krebs cycle, which in turn produces nicotinamide adenine dinucleotide (NADH), as well as adenosine triphosphate (ATP), CO 2 , and H 2 O. The NADH is a key electron (and H + ) donor in the process of oxidative phosphorylation, wherein O 2 and adenosine diphosphate are consumed and ATP and H 2 O are produced. Thus the net effect is oxidation of glucose to produce energy (ultimately as ATP), H 2 O, and CO 2 .
Transport of O 2 in the Blood
O 2 reaches the cells following transport by arterial blood, and the overall delivery of O 2 ( <SPAN role=presentation tabIndex=0 id=MathJax-Element-2-Frame class=MathJax style="POSITION: relative" data-mathml='D˙O2′>D˙O2D˙O2
D ˙ O 2
) is the product of the arterial blood O 2 content (CaO 2 ) and blood flow (cardiac output, <SPAN role=presentation tabIndex=0 id=MathJax-Element-3-Frame class=MathJax style="POSITION: relative" data-mathml='Q˙’>Q˙Q˙
Q ˙
) as
D˙O2=CaO2×Q˙
Oxygen is carried in the blood in two forms: O 2 bound to hemoglobin (the vast bulk), and O 2 dissolved in the plasma, and the content is expressed as the sum of these components:
CaO2=[(SaO2×Hb×O2combiningcapacityofHb)+(O2solubility×PaO2)]
where CaO 2 (O 2 content) is the milliliters of O 2 per 100 mL of blood, SaO 2 is the fraction of hemoglobin (Hb) that is saturated with O 2 , O 2 -combining capacity of Hb is 1.34 mL of O 2 per gram of Hb, Hb is grams of Hb per 100 mL of blood, Pa o 2 is the O 2 tension (i.e., dissolved O 2 ), and solubility of O 2 in plasma is 0.003 mL of O 2 per 100 mL plasma for each mm Hg Pa o 2
The binding of O 2 to hemoglobin is a complex, allosteric mechanism. Important insights can be gained by understanding how characteristic abnormalities of blood O 2 carriage (e.g., carbon monoxide [CO] poisoning, methemoglobinemia) affect O 2 tension, content, and delivery.
Methemoglobin (MetHb), formed by the oxidation to Fe 3+ (ferric) instead of the usual Fe 2+ (ferrous) iron, is less able to bind O 2 , resulting in diminished O 2 content and less O 2 delivery. Here, the Pa o 2 (in the absence of lung disease) will be normal: if the O 2 content is calculated from the Pa o 2 , it will appear normal, but if directly measured, it will be low. In contrast, MetHb level will be elevated. In severe cases, lactic acidosis develops because of impaired O 2 delivery. In addition, because MetHb has a blue-brown color, the patient will appear blue, even if the fraction of MetHb is modest; specialized oximetry can separately measure MetHb levels. The apparent cyanosis is not responsive to supplemental O 2 , and therapy involves converting (i.e., reducing) the MetHb to Hb (e.g., by using methylene blue). Important medical causes of MetHb include benzocaine, dapsone, or in susceptible patients, inhaled nitric oxide (NO).
In CO poisoning, the CO binds to Hb, with far greater (over 200-fold) avidity than molecular O 2 , tightly forming CO-Hb and resulting in two main effects. First, formation of CO-Hb results in fewer sites available for O 2 binding, and this reduces the blood O 2 content. Second, the formation of CO-Hb causes conformational changes in the Hb molecule such that the tendency to release bound O 2 is reduced. This effect corresponds to a leftward shift of the Hb-O 2 dissociation curve, and although this aspect of CO binding does not reduce the O 2 content or “global” delivery of O 2 , it does reduce the release of O 2 and its local delivery to the cells. Because the color of CO-Hb closely resembles that of O 2 -Hb, the color of the blood (and the patient) is bright red; however, as with MetHb, the PaO 2 will be normal (assuming no pulmonary disease) as will be the calculated CaO 2 ; however, the measured CaO 2 will be low and if severe, a lactic acidosis will be present. Modern pulse oximeters can distinguish between Hb-O 2 and CO-Hb.
Finally, the Bohr effect refers to a shift of the Hb-O 2 dissociation curve caused by changes in CO 2 or pH. In the systemic capillaries, the P co 2 is higher than in the arterial blood (and the pH correspondingly lower) because of local CO 2 production. These circumstances shift the Hb-O 2 dissociation curve to the right, which increases the offloading of O 2 to the tissues. The opposite occurs in the pulmonary capillaries; here, the P co 2 is lower (and the pH correspondingly higher) because of CO 2 elimination, and the dissociation curve is shifted to the left to facilitate O 2 binding to Hb.
Transport of Co 2 in the Blood
CO 2 is produced by metabolism in the mitochondria, where the CO 2 levels are highest. The transport path (involving progressively decreasing pressure gradients) is from mitochondria through cytoplasm, into venules, and finally, in mixed venous blood from where it is eliminated through the alveoli. In the blood, CO 2 is transported in three main forms: dissolved (reflected as Pa co 2 , partial pressure; accounts for approximately 5% of transported CO 2 ), bicarbonate ion (HCO 3 –; almost 90%), and carbamino CO 2 (CO 2 bound to terminal amino groups in Hb molecules; approximately 5%). The usual quantities of CO 2 in the arterial and (mixed) venous blood are approximately 21.5 and 23.3 mmol of CO 2 per liter of blood, respectively.
Breathing O 2 can sometimes induce hypercapnia, as occurs in patients with severe chronic lung disease who are breathing supplemental O 2 . Although traditionally thought to occur because increased Pa o 2 reduces ventilatory drive, this is now thought not to be the case, resulting instead from the Haldane effect, as well as from impairment of hypoxic pulmonary vasoconstriction (HPV). The Haldane effect is the difference in the amount of CO 2 carried in oxygenated versus deoxygenated blood, and two mechanisms explain this. First, increased Pa o 2 decreases the ability to form carbamino compounds—reducing the amount of CO 2 bound to Hb—thereby raising the amount of dissolved CO 2 (i.e., elevated P co 2 ). Second, the amino acid histidine, which has an imidazole group that is an effective H + buffer at physiologic pH, is an important linking molecule between heme groups and the Hb chains. Increasing the partial pressure of oxygen (PO 2 ) increases the amount of O 2 bound to Hb; this changes the conformation of the Hb molecule, which in turn alters the heme-linked histidine and reduces its H + buffering capacity. Therefore, more H + is free (not buffered) and binds to HCO 3 –, releasing stored CO 2 . Impairment of HPV by elevated O 2 allows increased perfusion to poorly ventilated regions; this has the effect of decreasing perfusion (and delivery of CO 2 ) to better ventilated regions, diminishing the efficiency of CO 2 exhalation. Patients with impaired ability to increase alveolar ventilation ( <SPAN role=presentation tabIndex=0 id=MathJax-Element-6-Frame class=MathJax style="POSITION: relative" data-mathml='V˙A’>V˙AV˙A
V ˙ A
) cannot compensate for the increased CO 2 availability, and therefore, in these patients, adding supplemental O 2 can result in elevated Pa co 2 .
Oxygenation in the Pulmonary Artery
Systemic venous blood (central venous blood) enters the right ventricle via the right atrium. The O 2 saturation (SO 2 ) differs among the major veins: higher venous SO 2 reflects greater blood flow, less tissue oxygen uptake, or both. SO 2 is usually higher in the inferior vena cava (IVC) than in the superior vena cava (SVC), possibly because of the high renal and hepatic flow relative to O 2 consumption. In the right ventricle, the central venous blood ( <SPAN role=presentation tabIndex=0 id=MathJax-Element-7-Frame class=MathJax style="POSITION: relative" data-mathml='ScvO2′>ScvO2ScvO2
S cv O 2
) from the SVC and IVC is joined by additional venous blood from the coronary circulation (via the coronary sinuses). In the right ventricle, an additional small amount of venous drainage from the myocardium enters through the thebesian veins, and as all this venous blood enters the pulmonary artery, it is well mixed and is termed mixed-venous blood ( <SPAN role=presentation tabIndex=0 id=MathJax-Element-8-Frame class=MathJax style="POSITION: relative" data-mathml='SvO2′>SvO2SvO2
S v O 2
); thus <SPAN role=presentation tabIndex=0 id=MathJax-Element-9-Frame class=MathJax style="POSITION: relative" data-mathml='SvO2<ScvO2′>SvO2<ScvO2SvO2<ScvO2
S v O 2 < S cv O 2
, although the trends of each usually run in parallel.
Ventilation
Ventilation refers to the movement of inspired gas into and exhaled gas out of the lungs.
Alveolar Ventilation
Fresh gas enters the lung by cyclic breathing at a rate and depth (tidal volume, V T ) determined by metabolic demand, usually 7 to 8 L/min. While most inspired gas reaches the alveoli, some (100-150 mL) of each V T remains in the airways and cannot participate in gas exchange. Such dead space (V D ) constitutes approximately one third of each V T . Anatomic V D is the fraction of the V T that remains in the “conducting” airways, and physiologic V D is any part of a V T that does not participate in gas exchange ( Fig. 13.1 ).

V ˙ A
. V D /V T , dead space to tidal volume ratio; <SPAN role=presentation tabIndex=0 id=MathJax-Element-11-Frame class=MathJax style="POSITION: relative" data-mathml='V˙A’>V˙AV˙A
V ˙ A
, alveolar ventilation; <SPAN role=presentation tabIndex=0 id=MathJax-Element-12-Frame class=MathJax style="POSITION: relative" data-mathml='V˙E’>?˙EV˙E
V ˙ E
, minute ventilation. <SPAN role=presentation tabIndex=0 id=MathJax-Element-13-Frame class=MathJax style="POSITION: relative" data-mathml='V˙E=V˙A+f×VD.’>?˙E=?˙A+?×?D.V˙E=V˙A+f×VD.
V ˙ E = V ˙ A + f × V D .
Double arrows indicate normal CO 2 exchange. COPD , Chronic obstructive pulmonary disease.
For a single tidal volume (V T , mL), the following is true:
VT=VA+VD
The product of V T (mL) times the respiratory rate (per minute) is the minute ventilation ( <SPAN role=presentation tabIndex=0 id=MathJax-Element-15-Frame class=MathJax style="POSITION: relative" data-mathml='V˙E’>V˙EV˙E
V ˙ E
). Aggregated over time, minute ventilation ( <SPAN role=presentation tabIndex=0 id=MathJax-Element-16-Frame class=MathJax style="POSITION: relative" data-mathml='V˙E’>V˙EV˙E
V ˙ E
, mL/min) is:
V˙E=V˙A+f×VD
The portion of the <SPAN role=presentation tabIndex=0 id=MathJax-Element-18-Frame class=MathJax style="POSITION: relative" data-mathml='V˙E’>V˙EV˙E
V ˙ E
that reaches the alveoli and respiratory bronchioles each minute and participates in gas exchange is called the alveolar ventilation ( <SPAN role=presentation tabIndex=0 id=MathJax-Element-19-Frame class=MathJax style="POSITION: relative" data-mathml='V˙A’>V˙AV˙A
V ˙ A
), and it is approximately 5 L/min. Because this is similar to the blood flow through the lungs (i.e., the cardiac output, also 5 L/min), the overall alveolar ventilation-perfusion ratio is approximately 1.
Dead Space Ventilation
Maintenance of Pa co 2 is a balance between CO 2 production ( <SPAN role=presentation tabIndex=0 id=MathJax-Element-20-Frame class=MathJax style="POSITION: relative" data-mathml='V˙CO2′>V˙CO2V˙CO2
V ˙ CO 2
, reflecting metabolic activity) and alveolar ventilation ( <SPAN role=presentation tabIndex=0 id=MathJax-Element-21-Frame class=MathJax style="POSITION: relative" data-mathml='V˙A’>V˙AV˙A
V ˙ A
). If <SPAN role=presentation tabIndex=0 id=MathJax-Element-22-Frame class=MathJax style="POSITION: relative" data-mathml='V˙E’>V˙EV˙E
V ˙ E
is constant but V D is increased, <SPAN role=presentation tabIndex=0 id=MathJax-Element-23-Frame class=MathJax style="POSITION: relative" data-mathml='V˙A’>V˙AV˙A
V ˙ A
will naturally be reduced, and the Pa co 2 will therefore rise. Therefore, if V D is increased, <SPAN role=presentation tabIndex=0 id=MathJax-Element-24-Frame class=MathJax style="POSITION: relative" data-mathml='V˙E’>V˙EV˙E
V ˙ E
must also increase to prevent a rise in Pa co 2 . Such elevations in V D occur when a mouthpiece or facemask is used, and in such cases, the additional V D is termed “apparatus deadspace” (which can be up to 300 mL; anatomic V D of the airways is 100-150 mL).
Increases in the volume of the conducting airways (e.g., bronchiectasis) increase the overall V D only slightly. Far more significant increases in V D occur when perfusion to a large number of ventilated alveoli is interrupted, as occurs in a pulmonary embolus (see Fig. 13.1 ). Indeed, with multiple pulmonary emboli, V D /V T can exceed 0.8 (2.7-fold normal). In such a case, to maintain a normal <SPAN role=presentation tabIndex=0 id=MathJax-Element-25-Frame class=MathJax style="POSITION: relative" data-mathml='V˙A’>V˙AV˙A
V ˙ A
(5 L/min), the <SPAN role=presentation tabIndex=0 id=MathJax-Element-26-Frame class=MathJax style="POSITION: relative" data-mathml='V˙E’>V˙EV˙E
V ˙ E
would have to increase (also 2.7-fold) to almost 20 L/min. This effort would cause considerable dyspnea, in addition to the dyspnea induced by the lowered PaO 2 .
Obstructive lung disease can result in diversion of inspired air into (nonobstructed) ventilated, but poorly perfused, regions of the lung. This results in local excesses of ventilation versus perfusion (high <SPAN role=presentation tabIndex=0 id=MathJax-Element-27-Frame class=MathJax style="POSITION: relative" data-mathml='V˙A/Q˙’>V˙A/Q˙V˙A/Q˙
V ˙ A / Q ˙
ratio) in such regions, which is equivalent to an increase in V D /V T (see Fig. 13.1 ). Patients with severe chronic obstructive pulmonary disease (COPD) may have a V D /V T ratio of up to 0.9, and would have to hyperventilate massively (30-50 L/min) to maintain normal Pa co 2 , which is not possible where ventilator reserve is diminished. Such patients demonstrate reduced <SPAN role=presentation tabIndex=0 id=MathJax-Element-28-Frame class=MathJax style="POSITION: relative" data-mathml='V˙A’>V˙AV˙A
V ˙ A
but often have an elevated <SPAN role=presentation tabIndex=0 id=MathJax-Element-29-Frame class=MathJax style="POSITION: relative" data-mathml='V˙E’>V˙EV˙E
V ˙ E
. An important compensatory mechanism is that a lower level of <SPAN role=presentation tabIndex=0 id=MathJax-Element-30-Frame class=MathJax style="POSITION: relative" data-mathml='V˙A’>V˙AV˙A
V ˙ A
will maintain stable CO 2 excretion where the Pa co 2 is increased ( Box 13.1 ).
Alveolar Oxygen Tension (P A o 2 )
PAO2=PIO2−PACO2R+[PACO2×FiO2×1−RR]
A simplified equation can be written without the compensation term:
PAO2=PIO2−PACO2R
Alveolar Ventilation
Alveolar ventilation ( <SPAN role=presentation tabIndex=0 id=MathJax-Element-33-Frame class=MathJax style="POSITION: relative" data-mathml='V˙A’>V˙AV˙A
V ˙ A
) can be expressed as
V˙A=f×(VT−VDS)
Alveolar ventilation can also be derived from:
V˙CO2=c×V˙A×FACO2
V ˙ CO 2
is CO 2 elimination, c is a conversion constant, and F aco 2 is the alveolar CO 2 concentration.
If <SPAN role=presentation tabIndex=0 id=MathJax-Element-37-Frame class=MathJax style="POSITION: relative" data-mathml='V˙A’>V˙AV˙A
V ˙ A
is expressed in L/min, <SPAN role=presentation tabIndex=0 id=MathJax-Element-38-Frame class=MathJax style="POSITION: relative" data-mathml='V˙CO2′>V˙CO2V˙CO2
V ˙ CO 2
in mL/min, and F aco 2 is replaced by P aco 2 in mm Hg, c = 0.863. By rearranging:
V˙A=V˙CO2×0.863PACO2
Static Lung Volumes—Functional Residual Capacity
The amount of air in the lungs after an ordinary expiration is called functional residual capacity (FRC; Fig. 13.2 ); it is usually 3 to 4 L and occurs because of the balance of inward (lung) forces and outward (chest wall) forces. The inward force is the “elastic recoil” of the lung and emanates from the elastic lung tissue fibers, contractile airway smooth muscle, and alveolar surface tension. The outward force is developed by passive recoil from the ribs, joints, and muscles of the chest wall. FRC is greater with increased height and age (loss of elastic lung tissue), and smaller in women and in obesity.

There are two reasons why maintenance of gas in the lung at end-expiration (i.e., FRC) is important. First, inflating an already opened (inflated) lung is easier than when the lung is deflated. This is because complete collapse results in liquid-only surfaces interfacing in alveoli (high surface tension), whereas alveoli in partially inflated lung have air-liquid interfaces (lower surface tension). Second, although perfusion in the lung is phasic, the frequency is rapid and the oscillations in flow are low, resulting in nearly continuous flow. Ventilation is different: the frequency is far slower and the size of the oscillations far larger. If the lung (or large parts of it) completely deflate between breaths, the blood flowing from closed alveoli (that contain zero O 2 ) would have very low SO 2 (the same as mixed venous blood); this would mix into the overall blood flow from the lungs and cause a major O 2 desaturation after every exhalation.
Respiratory Mechanics
The study of respiratory mechanics tells us how inspired air is distributed within the lung and permits quantitation of the severity of lung disease. The components of overall impedance to breathing results from elastance (the reciprocal of compliance), resistance, and inertia.
Compliance of the Respiratory System
The lung is like a rubber balloon that can be distended by positive pressure (inside) or negative pressure (outside). Under normal circumstances, inflation of the lung is maintained because although the pressure inside (alveolar pressure) is zero, the outside pressure (i.e., the pleural pressure) is sufficiently negative. The net distending pressure, which is the difference of the (positive) airway pressure (P AW ) and the (negative) pleural pressure (P PL ) is termed the transpulmonary pressure (P TP ). Thus:
PTP=PAW−PPL
Clearly, increasing the P AW increases the P TP . In addition, lowering the P PL (which is usually negative and making it more negative) also increases the P TP .
Compliance —the reciprocal of elastance—is the term that expresses how much distention (volume in liters) occurs for a given level of P TP (pressure, cm H 2 O); it is usually 0.2 to 0.3 L/cm H 2 O. However, although higher values of P TP maintain greater levels of lung opening, the relationship—as with most elastic structures—between applied pressure and resultant volume is curvilinear ( Fig. 13.3 ). Lung compliance depends on the lung volume; it is lowest at an extremely low or high FRC (see Fig. 13.3 ). In lung diseases characterized by reduced compliance (e.g., ARDS, pulmonary fibrosis, or edema), the pressure-volume (PV) curve is flatter and shifted to the right ( Fig. 13.4 ). In contrast, although emphysema involves the loss of elastic tissue, the overall loss of lung tissue (as seen on computed tomography [CT] scanning) means that the compliance is increased; the PV curve is therefore shifted to the left and is steeper (see Fig. 13.4 ).


Chest wall impedance is not noticed during spontaneous breathing because the respiratory “pump” includes the chest wall. Chest wall mechanics can be measured only if complete relaxation of the respiratory muscles can be achieved ; however, during mechanical ventilation, the respiratory muscles can be completely relaxed. As the lung is inflated by P AW , the properties of the chest wall will determine the resulting change in P PL . Under these circumstances, the increase in lung volume per unit increase in P PL is the chest wall compliance. Values of chest wall compliance are about the same as that of the lung and are reduced with obesity, chest wall edema, pleural effusions, and diseases of the costovertebral joints.
Resistance of the Respiratory System
Airways
Resistance impedes airflow into (and out of) the lung. The major component of resistance is the resistance exerted by the airways (large and small), and a minor component is the sliding of lung and the chest wall tissue elements during inspiration (and expiration). Resistance is overcome by (driving) pressure. In spontaneous breathing, driving pressure will be the P PL ; in positive pressure ventilation, the driving pressure will be the difference between the pressures applied to the endotracheal tube (P AW ; “source”) and the alveolus (P ALV ; “destination”). Resistance (R) is calculated as driving pressure (ΔP) divided by the resultant gas flow (F):
R=ΔPF
The value of airway resistance is approximately 1 cm H 2 O/L/sec, and is higher in obstructive lung disease (e.g., COPD, asthma); in severe asthma, it is elevated approximately tenfold. The presence of an endotracheal tube adds a resistance of 5 (or 8) cm H 2 O/L/min for a tube with internal diameter of size 8 (or 7) cm. For any tube for which the airflow is laminar (smooth, streamlined), the resistance increases in direct proportion to the tube length and increases dramatically (to the fourth power) as the diameter of the tube is reduced.
Two factors explain why most (approximately 80%) of the impedance to gas flow occurs in the large airways. First, as bronchi progressively branch, the resistances are arranged in parallel and the total cross-sectional area at the level of the terminal bronchioles adds up to almost tenfold that at the trachea. Second, in tubes that are large, irregular or branched, the flow is often turbulent, not laminar. When flow is laminar:
F(lam)=ΔPR
In contrast, when flow is turbulent:
F(turb)=ΔPR2
Therefore, for a given radius, far more pressure is required to achieve comparable flow where flow is turbulent; thus the effort required is greater and if prolonged or severe, respiratory failure is more likely.
Several factors can alter airflow resistance. First, resistance lessens as lung volume increases; this is intuitive, as increasing volume (positive pressure or spontaneous breathing) stretches the diameter of the airways. Because this is the key determinant of resistance, the resistance falls to a small extent. The opposite occurs with exhalation ( Fig. 13.5 ). However, as lung volume approaches residual volume (RV)—as can happen during anesthesia—the airways are narrowed in parallel with the compressing lung tissue and the resistance rises exponentially. These effects are apparent with active or passive ventilation. Second, active ventilation has additional effects. Forced expiration can compress small airways (i.e., that do not contain cartilage). In addition, forced expiration can cause turbulent flow in small airways in patients with COPD, precipitously dropping pressure in the lumen and thereby narrowing the bronchioles and resulting in expiratory flow limitation and, after multiple breaths, eventual “dynamic hyperinflation.” Expiring against resistance (or pursed-lips breathing) is sometimes used by those with COPD to make breathing easier. This works by increasing expiratory resistance and slowing expiration. The slowed expiration reduces the pressure gradient driving expiration (i.e., pressure highest in the alveolus, lower toward the mouth). Therefore, the point along the airway tree at which pressure inside the airway has decreased to less than that outside the airway (equal to pleural pressure) is moved from smaller collapsible airways toward the mouth to noncollapsible, cartilaginous airways ( Fig. 13.6 ); this prevents collapse of the smaller airways, which are vital for proper gas exchange.


The large airways (i.e., pharynx, larynx, and trachea) are outside the chest wall. During inspiration, the intrathoracic airways are exposed to extraluminal pressure (i.e., P PL ) that is less than the lumen pressure; in contrast, the extrathoracic airways are exposed to lumen pressure that is less than the extraluminal (i.e., atmospheric) pressure. This feature, coupled with downward stretch induced by inspiration, narrows the large extrathoracic airways; in the presence of preexisting narrowing (e.g., thyroid enlargement or tumor, paralyzed vocal cord, epiglottitis), this can critically reduce the cross-sectional area.
Tissue
Although not intuitively obvious, resistance of the lung tissue is the applied pressure on tissue divided by the resulting velocity of tissue movement. There are various approaches to determining this in humans, including separately considering the PV characteristics using plethysmography (where the area of the PV curve corresponds to work against total pulmonary resistance) and esophageal pressure (where the area of the PV curve corresponds to work against “tissue” resistance). Alternative approaches mathematically model the lung responses to varying respiratory frequencies. Lung tissue resistance amounts to 20% of the total resistance to breathing; it can be increased threefold or fourfold in chronic lung disease and is reduced by panting respirations. Finally, in adult respiratory distress syndrome (ARDS) the chest wall resistance is increased.
Inertia or Acceleration of Gas and Tissue
A final component of the total impedance to breathing is inertance, or the pressure required to accelerate air and tissue during inspiration and expiration. This component is minor, however, and can hardly be measured under normal breathing, regardless of whether the lungs are healthy. Nonetheless, tissue inertia is large during rapid ventilation, and it could be important during the rapid, shallow breathing characteristic of weaning failure or during high-frequency oscillation.
Distribution of Inspired Gas
Inspired gas is not evenly distributed throughout the lung; naturally, more gas enters those lung units that expand most during inspiration. In the resting lung, the basal (dependent) regions are less aerated than the apical (nondependent) regions; therefore, they have the capacity to undergo greater expansion. During inspiration, most gas goes to the basal units (dorsal, when supine; lower right lung when in the right lateral position). This distribution is because of the compliance properties of the lung and the effects of position on the distribution of the distending pleural pressure (i.e., the P PL gradient). These changes are not related to the properties of the inspired gas.
In the upright position, the P PL is less negative at the base of the lung than at the apex. Because the alveolus pressure (P A ) is uniform throughout the lung, the distending P TP is greater at the apex; therefore, before inspiration commences, the apical lung is more open (and is less compliant) than the basal lung ( Figs. 13.3 and 13.7 ). With inspiration, the contracting diaphragm lowers the P PL by a comparable amount in all areas of the pleural surface (because of the fluid-like behavior of normal lung ) and distends the basal more than the apical regions (see Figs. 13.3 and 13.7 ). Because the pleural pressure gradient is oriented according to gravity, the distribution of ventilation changes with body position.
The P PL gradient exists because lung density, gravity, and conformation of the lung to the shape of the thorax result in crowding of the basal lung tissue, making the local P PL less negative in the basal regions. Because the density of normal lung is approximately 0.3, P PL will become more positive by 0.3 cm H 2 O for each downward vertical centimeter, and more so with injured or edematous lungs. Indeed, experimentally induced weightlessness decreases inhomogeneity in the distribution of ventilation, but does not eliminate it; therefore, nongravitational (e.g., tissue, airway) factors also play a role.
Although the vertical height of the lung is the same in the prone and supine positions, the vertical gradient P PL is less when prone, perhaps because the mediastinum compresses the dependent lung when supine but rests on the sternum when prone. A more even distribution of inspired gas—with improved oxygenation—in the prone position was predicted by Bryan in 1974 44 ; this has been confirmed experimentally.
During low-flow states (e.g., at rest), distribution is determined by differences in compliance and not by airway resistance. Because compliance at the start of inflation is less in the (already more aerated) apex, ventilation is preferentially directed to the base. In contrast, at high airflow, resistance (not compliance) is the key determinant of distribution; because the resistance is lower in upper, more expanded lung regions, increasing flow rate equalizes the distribution of ventilation, as shown by distribution of 133 Xe gas in humans ( Fig. 13.8 ). This is important during exercise or stress because greater amounts of the alveolar-capillary surface area will be used.

Airway Closure
Expiration causes the airways to narrow, and deep expiration can cause them to close. The volume remaining above RV where expiration below FRC closes some airways is termed closing volume (CV), and this volume added to the RV is termed the closing capacity (CC; i.e., the total capacity of the lung at which closing can occur). Closure of airways during expiration is normal and is potentiated by increasing P PL , especially with active expiration. When P PL exceeds the P AW , the airway—if collapsible—will tend to close, and this usually commences at the bases because the basal P PL is greatest (see Fig. 13.7 ).

V ˙ A / Q ˙
ratios with more perfusion than ventilation (B) . This pattern is compatible with intermittent airway closure during breathing.
Three applications of this important principle are of key relevance to anesthesia. First, airway closure depends on age: in youth, the closure does not occur until expiration is at or near RV, whereas with older age, it occurs earlier in expiration (i.e., at higher lung volumes). This occurs because P PL is on average more “positive” (i.e., atmospheric, equal to P AW ) as age increases. Closing can occur at or above FRC in individuals aged 65 to 70 years, such that dependent regions will undergo closure during normal expiration. This may be the major reason why oxygenation decreases with age. Second, in the supine position, FRC is less than when upright, but CC is unchanged; therefore, exhalation of a usual V T (from FRC) encroaches on CC in a supine 45-year-old, and closure may be continuous in a supine 70-year-old ( Fig. 13.9 ). Finally, COPD increases the lung volume at which closure occurs, possibly exacerbated by airway edema and increased bronchial tone.

Diffusion of Gas
Gas moves in the large and medium-sized airways by bulk flow (i.e., convection), meaning that the gas molecules travel together at a given mean velocity according to a driving pressure gradient. Flow is through multiple generations of bronchi, and the net resistance falls with each division. After the 14th generation, airways merge with alveoli and participate in gas exchange (respiratory bronchioles). The cross-sectional area expands massively (trachea, 2.5 cm 2 ; 23rd generation bronchi, 0.8 m 2 ; alveolar surface, 140 m 2 ), resulting in a sharp drop in overall resistance. Because the number of gas molecules is constant, the velocity falls rapidly, which by the time the gas enters the alveoli is miniscule (0.001 mm/s); it is zero when it reaches the alveolar membrane. The velocity of the gas entering the alveolus is slower than the diffusion rates of O 2 and CO 2 ; therefore, diffusion—not convection—is necessary for transport in the distal airways and alveoli. Indeed, CO 2 is detectable at the mouth after just seconds of breath-holding, because of rapid diffusion and because of cardiac oscillations (i.e., mixing).
Gas mixing is complete in the alveoli of a normal lung during normal breathing. However, if the alveolus expands (e.g., emphysema), the diffusion distance may be too great to allow complete mixing, potentially leaving a layer of CO 2 -rich gas lining the alveolar membrane and a core of O 2 -rich gas in the alveolus. This represents a “micro” version of inhomogeneous distribution of ventilation.
Perfusion
The pulmonary circulation differs from the systemic circulation: it operates at a five to tenfold lower pressure, and the vessels are shorter and wider. There are two important consequences of the particularly low vascular resistance. First, the downstream blood flow in the pulmonary capillaries is pulsatile, in contrast to the more constant systemic capillary flow. Second, the capillary and alveolar walls are protected from exposure to high hydrostatic pressures; therefore, they can be sufficiently thin to optimize diffusion (i.e., exchange) of gas but not permit leakage of plasma or blood into the airspace. Whereas an abrupt increase in the pulmonary arterial (or venous) pressure can cause breaks in the capillaries, slower increases (i.e., months to years) stimulate vascular remodeling. This remodeling might protect against pulmonary edema (and possibly against lung injury ), but diffusion will be impaired.
Distribution of Lung Blood Flow
Pulmonary blood flow depends on driving pressure and vascular resistance; these factors (and flow) are not homogenous throughout the lung. The traditional thinking about lung perfusion emphasized the importance of gravity; however, factors other than gravity are also important.
Distribution of Blood Flow in the Lung: the Effect of Gravity
Blood has weight and therefore blood pressure is affected by gravity. The height (base to apex) of an adult lung is approximately 25 cm; therefore, when a person is standing, the hydrostatic pressure at the base is 25 cm H 2 O (i.e., approximately 18 mm Hg) higher than at the apex. The mean pulmonary arterial pressure is approximately 12 mm Hg at the level of the heart, and the pulmonary artery pressure at the lung apex can therefore approach zero. Thus less blood flow will occur at the apex (versus the base), and in the setting of positive pressure ventilation, the apical alveoli can compress the surrounding capillaries and prevent any local blood flow.
Based on such gravitational distribution of pulmonary artery pressure, as well as the effect of alveolar expansion, West and colleagues divided the lung into zones I to III ( Fig. 13.10 ). This system is based on the principle that perfusion to an alveolus depends on the pressures in the pulmonary artery (P PA ), pulmonary vein (P PV ), and alveolus (P ALV ). In the apex (zone I), the key issue is that pulmonary arterial pressure is less than alveolar pressure; therefore, no perfusion occurs. Zone I conditions can exist during mechanical ventilation and be exacerbated by low P PA . Whenever zone I conditions exist, the nonperfused alveoli constitute additional dead space (V D ). Below the apex in zone II, P PV is less than alveolar pressure, and the veins are collapsed except during flow, as in a “vascular waterfall.” Although P ALV is always greater than P PV , perfusion occurs when P PA exceeds P ALV (i.e., intermittently, during systole). Below this zone is zone III, in which there are two important differences: P PA and P PV both always exceed P ALV . As a result, there is perfusion throughout systole and diastole (and inspiration and expiration). Gravity results in equal increases in both P PA and P PV toward the lung base; therefore, gravity cannot affect flow throughout zone III by increasing the P PA to P PV pressure gradient alone. Nonetheless, it is possible that the greater weight of the blood nearer the base results in vessel dilatation, thereby lowering vascular resistance and increasing flow. It was subsequently recognized that there is also a decrease in perfusion in the lung base, or zone IV, that is thought to occur because of the effects of gravity compressing the lung at the bases—and the blood vessels therein—and thereby increasing vascular resistance.

Finally, additional evidence for the effect of gravity comes from volunteer experiments in which gravity was increased or abolished by altering the flight pattern of a jet aircraft. In these experiments, zero gravity decreased cardiac oscillations of O 2 and CO 2 during a breath-hold, indicating development of more homogeneous perfusion. In contrast, more recent experiments of exhaled gas analysis (on the Mir space station) reported that the heterogeneity of lung perfusion was reduced, but not eliminated, in the presence of microgravity, indicating that gravity contributes to the heterogeneity of blood flow distribution but does not explain it entirely. While the precise role of gravity is disputed, it is likely to play a smaller role when supine versus when upright.
Distribution of Blood Flow in the Lung: Influence of Factors Not Related to Gravity
Key experiments have reconsidered the effects of gravity. Blood flow measured in the same gravitational plane was less per unit of lung tissue at the apex than at the base. In addition, microsphere assessment demonstrated significant variability within iso-gravitational planes, and lung height appeared to account for less than 10% of the distribution of flow in either the prone or supine positions. In addition, inhomogeneity in the horizontal planes can exceed that in the vertical direction ( Fig. 13.11 ). Other studies have reported a preponderance of perfusion to the central lung (versus peripheral) tissue, which can be reversed by the application of positive end-expiratory pressure (PEEP). Although greater length of radial blood vessels was considered to explain this central-peripheral difference, others have suggested that it is not significant. Finally, differences have been reported among lung regions in local vascular resistance.

Fractal distribution of blood flow may be more important than the influence of gravity. A fractal pattern of perfusion means that in any given region, there will be “spatial correlation” (similarity) of the blood flow between neighboring regions.
Although the methods to study lung perfusion are complex—and there is a spectrum of opinion —the aggregate data suggest that factors other than gravity contribute to the heterogeneity of the distribution of perfusion.
Hypoxic Pulmonary Vasoconstriction
HPV is a compensatory mechanism that diverts blood flow away from hypoxic lung regions toward better oxygenated regions. The major stimulus for HPV is low alveolar oxygen tension (P A O 2 ), whether caused by hypoventilation or by breathing gas with a low PO 2 , and is more potent when affecting a smaller lung region. The stimulus of hypoxic mixed venous blood is weaker. Whereas in humans older volatile anesthetics were thought to inhibit HPV more than intravenously based anesthesia, modern volatile anesthetics, including sevoflurane and desflurane, have little effect. During intravenously based anesthesia, exposure of one lung to an FiO 2 of 1.0 and the contralateral lung to a hypoxic gas mixture (FiO 2 , 0.12 to 0.05) reduced perfusion to the hypoxic lung to 30% of the cardiac output. Pulmonary hypertension, because of vascular remodeling owing to ongoing HPV, can develop in humans at high altitude or in the presence of chronic hypoxemic lung disease.
Clinical Assessment of Lung Function
Spirometry—Total Lung Capacity and Subdivisions
The gas volume in the lung after a maximum inspiration is called the total lung capacity (TLC; usually 6 to 8 L). TLC can be increased in COPD either by overexpansion of alveoli or by destruction of the alveolar wall, resulting in loss of elastic tissue, as in emphysema (see Fig. 13.4 ). In extreme cases, TLC can be increased to 10 to 12 L. In restrictive lung disease, TLC is reduced, reflecting the degree of fibrosis, and can be as low as 3 to 4 L (see Fig. 13.4 ).
Following maximum expiratory effort, some air is left in the lung and constitutes the RV (approximately 2 L). However, usually no region develops collapse because distal airways (<2 mm) close before alveoli collapse, trapping gas and preventing further alveolar emptying. In addition, there is a limit to how much the chest wall, rib cage, and diaphragm can be compressed. The importance of preventing collapse of lung tissue was presented earlier (see Fig. 13.6 ).
The maximum volume that can be inhaled and then exhaled is the vital capacity (VC; 4-6 L), and this is the difference between TLC and RV. VC is reduced in both restrictive and obstructive lung disease. In restriction, VC reduction reflects the loss of lung volume, such as from the constricting (i.e., shrinking) effects of fibrosis. In obstructive lung disease, long-term trapping of air increases the RV and can occur either by encroaching on (and reducing) the VC or in association with a (proportionally smaller) increase in FVC.
Tidal volume (V T , usually 0.5 L) is inspired from the resting lung volume reached at end-expiration (FRC, 2.0 L). With increased ventilation, as in exercise, V T is increased and FRC may be reduced by approximately 0.5 L. However, in airway obstruction, exhalation is impeded such that inspiration commences before the usual resting lung volume is reached; thus end-expiratory volume is increased. Such air trapping reduces the resistance to gas flow in the narrowed airways, but because the lung tissue is hyperinflated and mechanically disadvantaged, the work of breathing overall is increased.
FRC increases with age as elastic lung tissue is lost; this reduces the lung recoil force countering the outward chest wall force, and the lung assumes a higher volume. The rate of this aging process is accelerated in COPD because of the contributions of chronic air trapping and marked loss of elastic tissue. FRC is reduced in fibrotic lung diseases, sometimes to 1.5 L (see Fig. 13.4 ). Lung resection also reduces FRC, but the remaining lung will expand to fill the lung tissue void partially; this is called compensatory emphysema (see Chapter 53 ).
Diffusing Capacity (DL CO )—Diffusion Across Alveolar-Capillary Membranes
The diffusing capacity for carbon monoxide (DL CO ) test integrates many phenomena that are central to respiratory physiology. The test and the factors affecting its interpretation are described here. In the lungs, O 2 and CO 2 diffuse passively: O 2 from alveolar gas into plasma and red cells, where it binds to hemoglobin, and CO 2 in the opposite direction, from plasma to the alveoli. The amount that can diffuse across a membrane in a given period is the diffusing capacity, and it is determined with the following equation:
Diffusingcapacity=(SA×ΔP×Sol)(h×MW)
where SA is the surface area of the membrane exposed to gas, ΔP is the gradient of partial pressure between administered gas versus blood tension, Sol is the solubility of the gas in the membrane, h is the thickness of the membrane, and MW is the molecular weight of the gas.
Assessment of diffusing capacity (sometimes called transfer factor ) uses CO as the test gas; it is inhaled at a small concentration (0.3%) to TLC just after a maximal expiration, filling the lung as much as possible with the dilute CO. The breath is held and then deeply exhaled to RV. The difference between the quantity of CO exhaled versus inhaled will therefore either be taken up by the perfusing blood (i.e., hemoglobin) or remain in the lung (RV). The latter can be determined if the CO is coadministered with an insoluble gas (e.g., helium) that remains in the lung.
Surface Area
The surface area is taken as the area that is capable of exchanging gas on the alveolar and the capillary sides; thus it assumes a ventilated and perfused lung (i.e., not dead space). It will be lower in small lungs, lung fibrosis (restriction), after lung resection, or in cases of lung tissue destruction, such as emphysema.
Membrane Thickness
Thicker membranes reduce the CO transfer because the longer diffusion distance lowers the diffusion capacity, and the solubility of O 2 (and CO 2 ) is lower in fibrotic tissue than in plasma. Differentiating between effects of the volume of capillary blood and the membrane thickness can be difficult, but because oxygen and CO compete for binding to hemoglobin, distinguishing between these issues may be possible by measuring CO transfer with altered FiO 2 (see review by Hughes and colleagues).
Pressure Gradient
The larger the O 2 or CO 2 tension difference (ΔP) between the gas phase (alveolus) and the plasma (capillary), the greater the rate of diffusion. The mixed venous blood entering the pulmonary capillary has a PO 2 of 40 mm Hg (5.3 kPa), and alveolar PO 2 is approximately 100 mm Hg (13.3 kPa); therefore, the driving pressure (ΔP) is 60 mm Hg (8 kPa).
When blood flows through the capillary, it takes up oxygen and delivers CO 2 , but because oxygen pressure builds up in capillary blood, the diffusion rate slows down and becomes zero when pressure is equilibrated across the alveolar-capillary wall. At rest, equilibrium is usually reached within 25% to 30% of the capillary length, and almost no gas transfer occurs in the remaining capillary ( Fig. 13.12 ). However, during exercise or stress (i.e., high cardiac output), blood flow through the capillary is faster, and a longer capillary distance is required before equilibrium is reached. Thickened alveolar-capillary membranes will also prolong the equilibration process and, if severe, can prevent equilibration occurring and increasing the propensity to hypoxemia. If the mixed venous PO 2 (P mv O 2 ) is lower than normal, the driving pressure increases and partially compensates toward achieving equilibrium with alveolar O 2 . The driving pressure is expressed:
ΔP=(PaO2−PmvO2)mmHg

Most of the oxygen that dissolves in plasma diffuses into the red cell and binds to hemoglobin; therefore, 1 L of blood (Hb 150 g/L) with a saturation of 98%—normal in arterial blood—carries 200 mL of Hb-bound O 2 , compared with 3 mL that is dissolved (PaO 2 100 mm Hg). The Hb-bound oxygen creates no pressure in plasma, which is important because it allows much more oxygen to diffuse over the membranes before a pressure equilibration is reached. Anemia (or prior CO exposure) reduces—and polycythemia increases—diffusion capacity.
Molecular Weight and Solubility
The rate of diffusion of a gas is inversely related to the square root of its MW; the larger the molecule, the slower the diffusion. O 2 is a light gas (MW 32), and CO 2 is heavier (MW 44). However, diffusion is also directly proportional to solubility in tissue, and CO 2 is almost 30-fold more soluble than O 2 . The aggregate effect is that CO 2 diffuses about 20-fold faster than O 2 82 ; therefore, there is no lung disease compatible with life that measurably impairs CO 2 diffusion.
Intraoperative Respiratory Events
Respiratory Function During Anesthesia
Anesthesia impairs pulmonary function, whether the patient is breathing spontaneously or is receiving mechanical ventilation. Impaired oxygenation of blood occurs in most subjects who are anesthetized, and this is why supplemental O 2 (FiO 2 usually 0.3-0.5) is almost invariably used. Mild to moderate hypoxemia (SaO 2 , 85% to 90%) is common and lasts from seconds to minutes; sometimes it is severe, and approximately 20% of patients may suffer from SaO 2 less than 81% for up to 5 minutes. Indeed, greater than 50% of claims in anesthesia-related deaths relate to hypoxemia during anesthesia. Beyond the operating room, the alterations in lung function acquired during anesthesia persist: clinically significant pulmonary complications can be seen in 1% to 2% of patients after minor surgery, and in up to 20% of patients after more major upper abdominal or thoracic surgery. Such consequences of anesthesia place prime importance on ascertaining the causes of perioperative respiratory dysfunction and the clinical approaches to treatment.
In this section, we describe the effects of anesthesia and mechanical ventilation on lung function. The arrangement of this section parallels the sequence of events involved in oxygenating the blood and removing CO 2 . Thus the first phenomenon that might be seen with anesthesia is loss of muscle tone with a subsequent change in the balance between outward forces (i.e., respiratory muscles) and inward forces (i.e., elastic tissue in the lung) leading to a fall in FRC. This causes or is paralleled by an increase in the elastic behavior of the lung (reduced compliance) and an increase in respiratory resistance. The decrease in FRC affects the patency of lung tissue with the formation of atelectasis (made worse with the use of high concentrations of inspired oxygen) and airway closure. This alters the distribution of ventilation and matching of ventilation and blood flow and impedes oxygenation of blood and removal of CO 2 .
Lung Volume and Respiratory Mechanics During Anesthesia
Lung Volume
Resting lung volume (i.e., FRC) is reduced by almost 1 L by moving from upright to supine position; induction of anesthesia further decreases the FRC by approximately 0.5 L. This reduces the FRC from approximately 3.5 to 2 L, a value close to RV. General anesthesia causes a fall in FRC (approximately 20%), whether breathing is controlled or spontaneous and whether the anesthetic is inhalational or intravenous; this is a major contributor to lowered oxygenation (discussed later). Muscle paralysis in the context of general anesthesia does not cause additional reduction in FRC.
The anatomic basis of the FRC reduction is not well understood. A landmark experiment on three volunteers using two-dimensional tomography suggested that a cephalad shift of the diaphragm, induced by anesthesia and paralysis, was responsible. Recent studies using CT scanning also suggest cephalad diaphragm shift, as well as a decrease in the transverse chest area. However, other data suggest little role for the diaphragm, with possible caudal (not cephalad) shift of its anterior aspect. Simple CT suggests a cranial displacement, except in severe obstructive lung disease. Although the anatomic components of reduced FRC are debatable, the mechanism appears to be related to loss of respiratory muscle tone. FRC is maintained by a balance of the forces inward (lung recoil) versus forces outward (chest wall recoil, chest wall muscles, diaphragm). For example, maintenance of muscle tone using ketamine as the anesthetic does not reduce FRC. Because patients are usually supine, the FRC will already have been reduced, and in elderly patients, this is particularly the case; in this context, the effects of anesthesia are more marked (see Fig. 13.9 ). As can be seen in the figure, FRC decreases with age assuming that weight does not change.
Compliance and Resistance of the Respiratory System
Static compliance of the total respiratory system (lungs and chest wall) is reduced on average from 95 to 60 mL/cm H 2 O during anesthesia. Most studies of lung compliance during anesthesia indicate a decrease compared with the awake state, and pooled data from several studies suggest that anesthesia is associated with a reduction in mean static compliance from almost 190 to approximately 150 mL/cm H 2 O. Data on changes in respiratory resistance are less clear. Although most studies suggest that anesthesia increases respiratory resistance, especially during mechanical ventilation, no studies have corrected for lung volume and flow rates (both affect resistance considerably), and it is possible that changes in resistance occur merely because of volume (i.e., FRC) loss ( Fig. 13.13 ).

Atelectasis and Airway Closure During Anesthesia
The classic article by Bendixen and colleagues proposed “a concept of atelectasis” as a cause of impaired oxygenation and reduced respiratory compliance during anesthesia. That study described a progressive decrease in compliance that paralleled decreases in oxygenation in both anesthetized humans and experimental animals, which was interpreted as progressive of atelectasis. However, others noticed an abrupt decrease in compliance and PaO 2 during induction of anesthesia, and yet atelectasis could not be shown on conventional chest radiography.
Since then, CT scanning has improved our knowledge of the nature of anesthesia-induced atelectasis, and the technique reveals prompt development of densities in the dependent regions of both lungs during anesthesia (data up to 1990 reviewed by Moller and associates). Morphologic studies of these densities in various animals supported the diagnosis of atelectasis. An example of atelectasis as seen on a CT scan is shown in Fig. 13.14 .

Atelectasis develops in approximately 90% of patients who are anesthetized, but it is unrelated to the choice of anesthesia. It is seen during spontaneous breathing and after muscle paralysis, and with either intravenous or inhaled anesthetics. The atelectatic area near the diaphragm is usually 5% to 6% of the total lung area, but can easily exceed 20%. The amount of lung tissue that is collapsed is larger, because the atelectatic area consists mostly of lung tissue, whereas normal aerated lung consists of 20% to 40% tissue (the rest being air). Thus 15% to 20% of the lung is atelectatic during uneventful anesthesia, before surgery has commenced; it decreases toward the apex, which usually remains aerated ( Fig. 13.15 ). However, this degree of atelectasis is larger (upward of 50% of lung volume) after thoracic surgery or cardiopulmonary bypass, and can last for several hours. Abdominal surgery adds little to the atelectasis, but after such surgery, it can persist for several days.

Atelectasis is an important cause of hypoxemia: there is a strong and significant correlation between the degree of atelectasis and the size of the pulmonary shunt ( R = 0.81), where atelectasis is expressed as the percentage of lung area just above the diaphragm on CT scan and shunt is expressed as the percentage of cardiac output using the multiple inert gas elimination technique (MIGET). The site of the increased shunt has been colocalized to the areas of atelectasis, using a technique that combines CT scanning and single photon emission computed tomography (SPECT; Fig. 13.16 ). In addition to shunt, atelectasis may form a focus of infection and can certainly contribute to pulmonary complications.

V ˙ A / Q ˙
, causing hypoxemia. In the next lowest region (zone C ), there is complete cessation of ventilation because of atelectasis, but some perfusion exists and causes a shunt. The farther from the top of the lung, the higher the perfusion; however, in the lowermost regions perfusion decreases (see text).
Aside from anesthesia (and the type of surgery), it is difficult to predict the development of atelectasis. The magnitude of atelectasis is often directly related to the body mass index (BMI) and inspired oxygen concentration. Moreover, neither age nor the presence of COPD predicts the development or extent of atelectasis. In COPD, it may be that airway closure precedes (and therefore prevents) alveolar closure. Alternatively, the greater loss of lung (elastic recoil) versus chest wall tissue may serve to protect against atelectasis.
Prevention of Atelectasis During Anesthesia
Several interventions can help prevent atelectasis or even reopen collapsed tissue, as discussed in the following sections.
Positive End-Expiratory Pressure
The application of PEEP (10 cm H 2 O) has been repeatedly demonstrated to reexpand atelectasis in part ( Fig. 13.17 ). Some atelectasis may persist and might require higher PEEP and inspiratory airway pressure. The application of larger levels of PEEP can have complex effects. Reversal of hypoxemia is not proportionally associated with applied PEEP, and a threshold exists in many cases. In addition, SaO 2 may decrease during the application of increased PEEP for two reasons. First, the increased P PL owing to the PEEP can impair venous return, especially in the presence of hypovolemia, lowering the cardiac output and oxygen delivery (DO 2 ) and thereby reducing mixed venous O 2 content ( <SPAN role=presentation tabIndex=0 id=MathJax-Element-48-Frame class=MathJax style="POSITION: relative" data-mathml='CvO2′>CvO2CvO2
C v O 2
). In the presence of an intrapulmonary shunt, such as with atelectasis, the mixed venous blood is shunted directly into pulmonary venous blood causing arterial desaturation. Second, increased PEEP can cause redistribution of blood flow away from the aerated, expanded regions (distended by PEEP) toward atelectatic areas (not distended by PEEP; Fig. 13.18 ). In this context, persisting atelectasis in a dependent lung receives a larger proportion of the total pulmonary blood flow than without PEEP. Finally, anesthesia-induced atelectasis rapidly reemerges after discontinuation of PEEP. Indeed, Hewlett and coworkers in 1974 cautioned against the “indiscriminate use of PEEP in routine anesthesia.”

V ˙ A / Q ˙
distributions in the lung of a healthy, awake subject during anesthesia (zero positive end-expiratory pressure [ZEEP] ) and during anesthesia (10 cm H 2 O positive end-expiratory pressure [PEEP] ). In the awake state, there is no atelectasis and the corresponding minor low <SPAN role=presentation tabIndex=0 id=MathJax-Element-50-Frame class=MathJax style="POSITION: relative" data-mathml='V˙A/Q˙’>V˙A/Q˙V˙A/Q˙
V ˙ A / Q ˙
distribution ( left side of plot) may reflect intermittent airway closure. During anesthesia with ZEEP, atelectasis is apparent in the lung bases (and the diaphragm has been pushed cranially). The low <SPAN role=presentation tabIndex=0 id=MathJax-Element-51-Frame class=MathJax style="POSITION: relative" data-mathml='V˙A/Q˙’>V˙A/Q˙V˙A/Q˙
V ˙ A / Q ˙
has been replaced by atelectasis and large shunt; in addition, a small “high” <SPAN role=presentation tabIndex=0 id=MathJax-Element-52-Frame class=MathJax style="POSITION: relative" data-mathml='V˙A/Q˙’>V˙A/Q˙V˙A/Q˙
V ˙ A / Q ˙
mode ( right side of plot) may reflect alveolar dead space in upper lung regions. With the addition of PEEP during anesthesia, the collapsed lung tissue has been recruited and the shunt has been reduced considerably. Moreover, the “high” <SPAN role=presentation tabIndex=0 id=MathJax-Element-53-Frame class=MathJax style="POSITION: relative" data-mathml='V˙A/Q˙’>V˙A/Q˙V˙A/Q˙
V ˙ A / Q ˙
mode ( right side of plot) has significantly increased; this may reflect additional inflation of nonperfused upper lung.

To avoid negative circulatory effects, the magnitude of PEEP should just be enough to open up a collapsed lung. A PEEP of 7 cm H 2 O in normal-weight patients (BMI < 25 kg/m 2 ) without cardiopulmonary disease will recruit most of the lung, and keep it open with better oxygenation than in a comparable group without PEEP. Thus a forceful dedicated recruit maneuver should not be needed. This effect was seen in nonabdominal surgery, and whether similar beneficial effect will be seen in abdominal surgery remains to be shown.
Recruitment Maneuvers
A sigh maneuver, or a large V T , has been suggested for reversing atelectasis ; however, atelectasis is not uniformly reduced by a V T increase or sigh up to P AW of 20 cm H 2 O. Instead, a P AW of 30 cm H 2 O is required for initial opening, and 40 cm H 2 O for more complete reversal ( Fig. 13.19 ). In the presence of normal lungs, such inflation is equivalent to a VC and can therefore be called a VC maneuver (albeit achieved with positive P AW ). In addition, a significant hemodynamic effect is likely if the VC maneuver is sustained; in fact, inflation with a P AW of 40 cm H 2 O for 7 to 8 seconds appears to successfully open almost all anesthesia-induced atelectasis.

Minimizing Gas Resorption
Although recruitment of anesthesia-induced atelectasis is completely possible with either PEEP or a VC maneuver, continuous application of some level of PEEP is required to prevent rapid recurrence of the atelectasis. However, nitrogen (N 2 )—an insoluble gas that is not absorbed into the blood—can “splint” the alveolus if the alveolus is already opened. As a result, in anesthetized patients, a VC maneuver followed by ventilation with a gas mixture containing 60% N 2 (40% O 2 ) reduced the propensity for reaccumulation of atelectasis, with only 20% reappearing 40 minutes after recruitment.
The same principles apply in the practice of preoxygenation of patients during induction of anesthesia. Here, the aim is to prevent O 2 desaturation (i.e., gain an O 2 safety margin) during induction before the airway has been secured when the anesthesiologist can better manage ventilation and oxygenation. Traditionally, the application of FiO 2 1.0 has been used. Although the SaO 2 is usually well maintained with this approach, atelectasis inevitably forms. The use of 30% versus 100% O 2 during induction was demonstrated in a clinical study to eliminate the formation of atelectasis. Later, a comparison of breathing 100%, 80%, and 60% O 2 during induction demonstrated ubiquitous atelectasis with 100%, less with 80%, and even less with 60% O 2 ( Fig. 13.20 ); however, the trade-off for less atelectasis was a shorter safety margin before occurrence of O 2 desaturation.

An alternative approach may be continuous positive airway pressure (CPAP). Application of CPAP 10 cm H 2 O permitted the use of 100% inspired O 2 without formation of significant degrees of atelectasis. This might provide an ideal combination of minimal risk of either O 2 desaturation or atelectasis, but it has not been repeatedly verified.
Maintenance of Muscle Tone
Because loss of muscle tone in the diaphragm or chest wall appears to increase the risk of atelectasis, techniques that preserve muscle tone may have advantages. Intravenous ketamine does not impair muscle tone and is the only individual anesthetic that does not cause atelectasis. If neuromuscular blockade is added, atelectasis occurs as with other anesthetics. Ketamine is an extremely useful anesthetic in special circumstances but has challenges with widespread use.
An experimental approach is restoration of respiratory muscle tone by diaphragm pacing. This approach is achieved with phrenic nerve stimulation, and it can modestly reduce the degree of atelectasis; however, the effect is minor and the approach is complicated.
Atelectasis Following Surgery
Hypoxemia is common after anesthesia and surgery. It is enhanced by breathing oxygen before induction of anesthesia and suctioning of the airway (negative pressure) before extubation of the trachea. In addition, splinting and inhibition of coughing associated with pain can cause atelectasis postoperatively. Several approaches have been tried to address such atelectasis-associated hypoxemia following surgery. Administration of 100% O 2 coupled with a VC maneuver is not effective; this is probably because while the VC maneuver recruits the lung, the alveolar opening is not maintained (in fact closure is encouraged by the N 2 -free O 2 ). However, a VC maneuver followed by a lower O 2 concentration (40% O 2 in N 2 ) can maintain an open lung until the end of anesthesia. Oxygenation is sustained for a longer period following ventilation with 50% O 2 in air (i.e., N 2 ) compared with 100%, following cardiopulmonary bypass. In addition, use of 100% inspired oxygen before extubation increases the propensity to atelectasis and treatment of postoperative hypoxemia, considered to be due to atelectasis, is associated with better outcomes when CPAP is used instead of 100% O 2 .
Airway Closure
Intermittent airway closure reduces ventilation of the affected alveoli. Such lung regions can become regions of low <SPAN role=presentation tabIndex=0 id=MathJax-Element-54-Frame class=MathJax style="POSITION: relative" data-mathml='V˙A/Q˙’>V˙A/Q˙V˙A/Q˙
V ˙ A / Q ˙
if perfusion is maintained or is not reduced to the same degree as ventilation. The propensity to airway closure increases with age (see Fig. 13.9 ), as does perfusion to low <SPAN role=presentation tabIndex=0 id=MathJax-Element-55-Frame class=MathJax style="POSITION: relative" data-mathml='V˙A/Q˙’>V˙A/Q˙V˙A/Q˙
V ˙ A / Q ˙
regions. Anesthesia reduces FRC by about 0.5 L, which increases airway closure during tidal ventilation. In fact, the reduction in ventilation in the nonatelectatic lung ( Fig. 13.21 ) is caused by airway closure. In addition, ventilation in these regions is less than perfusion (i.e., regions of low <SPAN role=presentation tabIndex=0 id=MathJax-Element-56-Frame class=MathJax style="POSITION: relative" data-mathml='V˙A/Q˙’>V˙A/Q˙V˙A/Q˙
V ˙ A / Q ˙
) and contributes to impaired oxygenation during anesthesia. Taken together, the combination of atelectasis and airway closure explain about 75% of the overall impairment in oxygenation. In addition, where (CV–ERV) indicates the amount of airway closure occurring above FRC (and ERV is expiratory reserve volume), this value increased with induction of anesthesia, and there is good correlation between low <SPAN role=presentation tabIndex=0 id=MathJax-Element-57-Frame class=MathJax style="POSITION: relative" data-mathml='V˙A/Q˙’>V˙A/Q˙V˙A/Q˙
V ˙ A / Q ˙
and the extent of airway closure. In summary, a simple three-compartment lung model (normal <SPAN role=presentation tabIndex=0 id=MathJax-Element-58-Frame class=MathJax style="POSITION: relative" data-mathml='V˙A/Q˙’>V˙A/Q˙V˙A/Q˙
V ˙ A / Q ˙
matching regions, region of airway closure, and atelectatic lung) describes well the components contributing to impairment of oxygenation during anesthesia (see Fig. 13.21 ).

Distribution of Ventilation and Blood Flow During Anesthesia
Distribution of Ventilation
Redistribution of inspired gas away from dependent to nondependent lung regions has been demonstrated, using isotope techniques in anesthetized supine humans. Radiolabeled aerosol and SPECT demonstrate that ventilation is distributed mainly to the upper lung regions, with a successive decrease toward the lower lung regions, and an absence of ventilation in the lower-most regions, a finding consistent with the atelectasis demonstrable using CT (see Fig. 13.16 ).
Recruitment maneuvers increase dependent lung ventilation in anesthetized subjects in the lateral and supine positions, restoring the distribution of ventilation to that in the awake state. Thus restoration of overall FRC toward the awake level returns gas distribution toward the awake pattern. The explanations are recruitment of atelectatic lung, reopening of closed airways, and further expansion of already expanded (upper) lung regions, decreasing regional compliance and lessening incremental ventilation.
Distribution of Lung Blood Flow
The distribution of lung blood flow has been studied by injection of radioactively labeled macroaggregated albumin and SPECT. During anesthesia, a successive increase in perfusion occurs from upper toward lower regions, with a slight drop in perfusion in the lowermost portion of the lung, which is atelectatic on simultaneous CT (see Fig. 13.16 ). PEEP will impede venous return to the right heart and reduce cardiac output. It can also affect pulmonary vascular resistance, although this would have little effect on cardiac output. In addition, PEEP redistributes blood flow toward dependent lung regions, reducing flow (and increasing dead space) in the upper lung; the increased dependent flow may increase shunt through atelectatic lung.
Hypoxic Pulmonary Vasoconstriction
Several inhaled—but not intravenous—anesthetics inhibit HPV in isolated lung preparations. Human studies of HPV are complex with multiple parameters changing simultaneously, thereby confounding the HPV response with changes in cardiac output, myocardial contractility, vascular tone, blood volume distribution, pH, Pco 2 , and lung mechanics. However, studies with no obvious changes in cardiac output indicated that isoflurane and halothane depress the HPV response by 50% at a minimum alveolar concentration (MAC) of 2 ( Fig. 13.22 ).

Ventilation-Perfusion Matching During Anesthesia
Dead Space, Shunt, and Ventilation-Perfusion Relationships
CO 2 Elimination
Anesthesia impairs CO 2 elimination and oxygenation of blood. The explanation for reduced CO 2 elimination is reduced minute ventilation ( <SPAN role=presentation tabIndex=0 id=MathJax-Element-59-Frame class=MathJax style="POSITION: relative" data-mathml='V˙E’>V˙EV˙E
V ˙ E
) because of respiratory depression, or where this is preserved, because of an increase in the <SPAN role=presentation tabIndex=0 id=MathJax-Element-60-Frame class=MathJax style="POSITION: relative" data-mathml='VD/VT’>VD/VTVD/VT
V D /V T
. Single-breath wash out recordings demonstrate that “anatomic” dead space is unchanged, indicating that increased <SPAN role=presentation tabIndex=0 id=MathJax-Element-61-Frame class=MathJax style="POSITION: relative" data-mathml='VD/VT’>VD/VTVD/VT
V D /V T
is alveolar and confirmed by MIGET scan ( Fig. 13.23 ). Such high <SPAN role=presentation tabIndex=0 id=MathJax-Element-62-Frame class=MathJax style="POSITION: relative" data-mathml='V˙A/Q˙’>V˙A/Q˙V˙A/Q˙
V ˙ A / Q ˙
can be explained by the perfusion of small corner vessels in interalveolar septa in the upper lung regions, where alveolar pressure can exceed pulmonary vascular pressure (zone I). The impaired CO 2 elimination is most easily corrected by increasing the ventilation and is seldom a problem in routine anesthesia with mechanical ventilation.
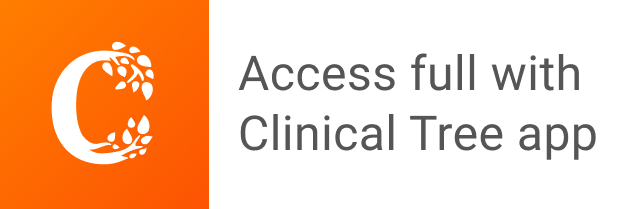