Key words
capnography, infrared spectroscopy, lung imaging, pulse oximetry, respiratory mechanics
Key Points
- ▪
Intraoperative respiratory monitoring is a fundamental component of the American Society of Anesthesiologists’ standards for basic anesthetic monitoring. Monitoring of oxygenation and ventilation is essential for the safe conduct of an anesthetic.
- ▪
A thorough understanding of the physiological and technological principles underlying respiratory monitoring is essential for its appropriate clinical application.
- ▪
The majority of respiratory monitors in clinical use provide information at the systemic and whole-lung level from which inferences are made regarding the regional lung and tissue-level conditions.
- ▪
The degree of invasiveness of utilized monitors should be determined by clinical requirements.
- ▪
Pulse oximetry is a noninvasive, reliable, and simple method for continuously monitoring the fractional arterial oxygen saturation.
- ▪
Ventilation-perfusion mismatch, shunt, and hypoventilation are the most common causes of hypoxemia in the perioperative period. Monitoring of gas exchange, and its response to various interventions, may differentiate etiologies for hypoxemia.
- ▪
Mixed venous oxygen saturation ( <SPAN role=presentation tabIndex=0 id=MathJax-Element-1-Frame class=MathJax style="POSITION: relative" data-mathml='Sv¯O2′>Sv⎯⎯O2Sv¯O2
S v ¯ O 2
) allows for monitoring of the global balance between oxygen delivery and consumption. Its measurement provides information on gas exchange, cardiac output, and global oxygen consumption.
- ▪
Systems utilizing near infrared spectroscopy are used clinically to monitor regional tissue oxygenation, particularly in the brain. The value of regional tissue oxygenation monitoring for clinical management is currently being established.
- ▪
Capnography is the primary quantitative method to assess ventilation in the perioperative period. Besides providing physiologic information on ventilation, pulmonary blood flow, and aerobic metabolism, capnography is important for verifying the endotracheal tube positioning, and determining the integrity of the breathing circuit.
- ▪
End-tidal carbon dioxide (CO 2 ) is not always a reliable approximation of arterial CO 2 tension, especially in the presence of significant heterogeneity in the distribution of ventilation and perfusion.
- ▪
Measurement of the pressures, flows, and volumes associated with ventilation is necessary to optimize mechanical ventilation, as well as to detect pathophysiologic mechanical derangements of the respiratory system (i.e., increased airway resistance or reduced lung compliance).
- ▪
Imaging techniques have emerged as important tools for respiratory monitoring. Lung ultrasonography is increasingly utilized in emergency and perioperative settings, allowing for prompt bedside assessments of pulmonary abnormalities such as pneumothorax, lung edema, consolidation, and pleural effusions. Electrical impedance tomography is another noninvasive imaging technique that provides information on lung aeration and recruitment.
- ▪
Current approaches to respiratory monitoring primarily assess pulmonary mechanical and global gas exchange processes. The monitoring of tissue and subcellular respiration remains a desirable goal for future innovation.
Overview of Respiratory Monitoring
Respiratory monitoring is essential to every anesthetic. Its major relevance for maintenance of homeostasis and patient safety is acknowledged by its mandatory position in national and international standards for anesthetic monitoring. Through the decades, advances in respiratory monitoring have resulted in substantial reduction in anesthetic morbidity and mortality, and opened a new era of safe anesthetic practice.
Respiration is the transport of oxygen (O 2 ) from the environment to the body cells and the transport of carbon dioxide (CO 2 ) from those cells to the environment. The concept includes a component of cellular respiration, the process by which cells obtain energy in the form of adenosine triphosphate from the controlled reaction of hydrogen with O 2 to form water. Thus, in its broadest sense, respiratory monitoring refers to the continuous or periodic assessment of processes involved with the exchange of respiratory gases between the environment and the subcellular pathways where those gases are utilized and produced ( Fig. 41.1 ). Respiratory monitoring includes assessment of (1) convective and diffusive gas transport through the branching airway tree and alveoli, (2) equilibration of gases between alveoli and pulmonary capillary blood, (3) mass balance of the distinct regional ventilation and perfusion contributions to produce expired gases and arterial and mixed venous blood, (4) gas transport between the blood and body tissues through the microcirculation, (5) gas diffusion between tissues and mitochondria, and (6) cellular respiration with O 2 use and CO 2 production.

S v ¯ O 2
, mixed venous oxygen saturation; S p O 2 , peripheral oxygen saturation; US, ultrasound.
Advances in physiologic measurements have enhanced our understanding of these stages of respiratory function during anesthesia. This chapter provides an overview of current and emerging techniques of respiratory monitoring. Despite these technical advancements, current instrumentation is limited in providing accurate and comprehensive information on respiratory function in anesthetized and critically ill patients. The area is therefore rich for research to advance the monitoring of all components of respiration.
American Society of Anesthesiologists Standards
The word monitoring is often associated with electronic instrumentation, and it is noteworthy that the current American Society of Anesthesiologists’ (ASA) Standards for Basic Anesthetic Monitoring states in Standard I that “Qualified anesthesia personnel shall be present in the room throughout the conduct of all general anesthetics, regional anesthetics, and monitored anesthesia care” ( Box 41.1 ). This precedes reliance on any instrumentation (as implied in Standard II), and clearly indicates that the anesthesia provider brings essential expertise and interpretation to monitoring beyond information provided by equipment. Increased safety in our specialty lies primarily in high-quality training and environments that encourage continuing education, and not exclusively on new technology. The ASA Standards for Basic Anesthetic Monitoring reflect monitoring principles during anesthesia established in the 1980s 3 and should be systematically followed. The standards represent a foundation to additional monitoring according to clinical requirements.
Standard I
Qualified anesthesia personnel shall be present in the room throughout the conduct of all general anesthetics, regional anesthetics, and monitored anesthesia care.
Standard II
During all anesthetics, the patient’s oxygenation, ventilation, circulation, and temperature shall be continually † evaluated.
† Note that “continual” is defined as “repeated regularly and frequently in steady rapid succession” whereas “continuous” means “prolonged without any interruption at any time.”
FLOAT NOT FOUNDOxygenation
Objective: To ensure adequate oxygen concentration in the inspired gas and the blood during all anesthetics.
Methods
Inspired gas: During every administration of general anesthesia using an anesthesia machine, the concentration of oxygen in the patient breathing system shall be measured by an oxygen analyzer with a low oxygen concentration limit alarm in use. ∗
∗ Under extenuating circumstances, the responsible anesthesiologist may waive the requirements marked with an asterisk ( ∗ ); it is recommended that when this is done, it should be so stated (including the reasons) in a note in the patient’s medical record.
Blood oxygenation: During all anesthetics, a quantitative method of assessing oxygenation such as pulse oximetry will be employed. ∗ When the pulse oximeter is utilized, the variable pitch pulse tone and the low threshold alarm will be audible to the anesthesiologist or the anesthesia care team personnel. ∗ Adequate illumination and exposure of the patient are necessary to assess color. ∗
Ventilation
Objective: To ensure adequate ventilation of the patient during all anesthetics.
Methods
Every patient receiving general anesthesia will have the adequacy of ventilation continually evaluated. Qualitative clinical signs such as chest excursion, observation of the reservoir breathing bag and auscultation of breath sounds are useful. Continual monitoring for the presence of expired carbon dioxide will be performed unless invalidated by the nature of the patient, procedure, or equipment. Quantitative monitoring of the volume of expired gas is strongly encouraged. ∗
When an endotracheal tube or laryngeal mask is inserted, its correct positioning must be verified by clinical assessment and by identification of carbon dioxide in the expired gas. Continual end-tidal carbon dioxide analysis, in use from the time of endotracheal tube/laryngeal mask placement, until extubation/removal or initiating transfer to a postoperative care location, will be performed using a quantitative method such as capnography, capnometry, or mass spectroscopy. ∗ When capnography or capnometry is utilized, the end tidal CO 2 alarm will be audible to the anesthesiologist or the anesthesia care team personnel. †
When ventilation is controlled by a mechanical ventilator, there will be in continuous use a device that is capable of detecting disconnection of components of the breathing system. The device must give an audible signal when its alarm threshold is exceeded.
During regional anesthesia (with no sedation) or local anesthesia (with no sedation), the adequacy of ventilation will be evaluated by continual observation of qualitative clinical signs. During moderate or deep sedation the adequacy of ventilation will be evaluated by continual observation of qualitative clinical signs and monitoring for the presence of exhaled carbon dioxide unless precluded or invalidated by the nature of the patient, procedure, or equipment.
The Physical Examination
Physical examination remains an essential component of perioperative respiratory monitoring. It provides essential information for diagnosis and treatment, and may be the first indication of changes in patient status requiring intervention. Physical examination has limitations, but it routinely allows for detection of information relevant for the management of the patient.
Respiratory monitoring starts with inspection of the patient, either awake or during anesthesia. In elective cases, the anesthesiologist will have time to investigate causes for abnormal presentations. In emergent situations, careful inspection may be the only source of information for timely and accurate anesthetic management. The observation of respiratory distress should prompt immediate search for specific causes. Assessment of the respiratory rate provides a measure of the breathing pattern. For example, during sepsis, respiratory rate is significantly correlated with disease severity. Anatomic signs relevant to respiration include (but are not limited to) deformities of the chest wall and spine, goiter, tracheostomy scar, and tracheal deviation. Functional elements to be noticed include the components of inspiration and expiration (diaphragmatic versus thoracic), duration and difficulty of inspiration and expiration, paradoxical chest wall motion, use of accessory muscles, central and peripheral cyanosis, pallor, wheezing, stridor, cough and sputum, aphonia, splinting, and clubbed fingers. Neck vein distension should be examined for a potential cardiovascular contribution to respiratory distress, noting that it is a less reliable indicator of central venous pressure during significant dyspnea. Attention should be paid to painful respiration in trauma patients, as well as the possibility of flail chest, pericardial tamponade, hemothorax, pneumothorax, pulmonary contusion, and tension pneumothorax.
Auscultation of the lung during anesthesia is another essential skill in physical diagnosis. Ambient noise, individual hearing limitations, and the acoustic properties of the stethoscope all influence the anesthesiologist’s clinical judgment. A stethoscope of sufficient quality will allow for identification of distinctive normal and abnormal breath sounds: vesicular sounds, ronchi, wheezes, fine and coarse crackles, inspiratory stridor, and pleural friction. A clear understanding of the acoustic mechanisms for each of these sounds is essential for adequate clinical assessment.
Pulse Oximetry
Physiologic Fundamentals
The primary role of the cardiorespiratory system is the transport of O 2 and CO 2 throughout the body. O 2 delivery is quantified as the product of arterial O 2 content and cardiac output (see Chapter 13 , “Respiratory Physiology and Pathophysiology”). Arterial O 2 content (C a O 2 , in mL of O 2 per 100 mL of blood [hemoglobin—Hb], mL/100 mL) is calculated as
CaO2=(1.34×SaO2×Hb)+0.0031×PaO2
where 1.34 mL/g is the O 2 binding capacity of Hb (i.e., Hüfner constant, theoretically equal to 1.39 mL/g but experimental range between 1.31 and 1.37 mL/g because of the presence of small amounts of other Hb species ); S a O 2 is the O 2 saturation of Hb in the arterial blood (percent saturation/100); Hb is the concentration of Hb in the arterial blood (g/dL); 0.0031 is the solubility of O 2 in blood (mL/100 mL/mm Hg); and P a O 2 is the arterial partial pressure of O 2 (mm Hg). As can be inferred from Eq. (41.1) , S a O 2 and Hb are the major determinants of O 2 content in the blood and consequently O 2 delivery to the tissues.
Five species of Hb are found in adult blood: oxygenated Hb (O 2 Hb), deoxygenated Hb (deO 2 Hb); carboxyhemoglobin (COHb); methemoglobin (MetHb); and sulfhemoglobin (SHb). Under normal circumstances, the concentrations of COHb, MetHb, and SHb are small (1%-3% for COHb and less than 1% for MetHb and SHb). Functional O 2 saturation (S a O 2 ) refers to the amount of O 2 Hb as a fraction of the total amount of O 2 Hb and deO 2 Hb and is expressed as
FunctionalSaO2=[O2Hb][O2Hb]+[deO2Hb]×100%
The O 2 Hb fraction or fractional saturation is defined as the amount of O 2 Hb as a fraction of the total amount of Hb :
FractionalSaO2=[O2Hb][O2Hb]+[deO2Hb]+[COHb]+[MetHb]+[SHb]×100%
S a O 2 is a function of the P a O 2 . The relationship between the two is described by the O 2 Hb dissociation curve ( Fig. 41.2 ). As can be appreciated by inspection of the curve, the relationship is not linear. This has important consequences. First, a high S a O 2 cannot discriminate between normoxic and hyperoxic conditions; this can be relevant when attempting to limit O 2 exposure in neonates or patients at risk for O 2 toxicity. Second, a large numeric change in P a O 2 at the flat portion of the curve (P a O 2 approximately above 70 mm Hg) has relatively small consequences in terms of blood O 2 content. Furthermore, changes in temperature, pH, P a CO 2 , and erythrocyte 2,3-diphosphoglycerate concentration can shift the curve such that the same S a O 2 can be present under a range of different oxygen partial pressures (PO 2 ). This may be relevant, given that diffusion from the microcirculation to tissue depends on PO 2 gradients.

Measurement Principles
Oximetry
Oximetry is the measurement of the O 2 saturation of Hb. It is an application of the Beer-Lambert law ( Eq. 41.4 ), which relates the transmission of light through a solution to the concentration of the solute in the solution. For each solute in a solution,
Itrans=Iine−DCε
where I trans is the intensity of transmitted light, I in is the intensity of the incident light, e is the base of the natural logarithm, D is the distance the light is transmitted through the solution, C is the concentration of the solute, and ε is the extinction coefficient of the solute.
The concentration of a single solute in solution can be calculated by measuring the amount of light transmitted through the solution as long as the other variables are known. For a solution containing multiple solutes, the calculation of the concentrations of the different solutes requires that light absorption be measured at a number of different wavelengths at least equal to the number of solutes. In a sample of blood in a cuvette, the absorption of a given wavelength of light passing through the blood will depend on the concentrations of the different species of Hb. Fig. 41.3 A illustrates the absorption spectra of the five species of Hb for wavelengths of light along the visible spectrum. To measure the concentrations of all five species of Hb in a sample of blood, light absorption of at least five different wavelengths must be measured. This measurement is typically conducted using a co-oximeter. A co-oximeter uses the principle of oximetry to measure the S a O 2 as well as the concentrations of other Hb species in a blood sample. Co-oximetry is considered the gold standard for S a O 2 measurements and is relied on in circumstances when pulse oximetry readings are inaccurate or unobtainable.

Pulse Oximetry
Standard pulse oximetry aims to provide a noninvasive, in vivo, and continuous assessment of functional S a O 2 . Estimates of S a O 2 based on pulse oximetry are denoted as S p O 2 . The history of the development of the pulse oximetry has been reviewed in detail elsewhere.
Pulse oximetry takes advantage of the pulsatility of arterial blood flow to provide an estimate of S a O 2 by differentiating light absorption by arterial blood from light absorption by other components. When compared with in vitro oximetry of an arterial blood sample, the challenge of obtaining arterial O 2 saturation in vivo is to ensure that the light is sampling arterial blood and to account for its absorption by other tissues. As illustrated in Fig. 41.4 , light absorption by tissue can be divided into a time-varying (pulsatile) component, historically referred to as “AC” (from “alternating current”), and a steady (nonpulsatile) component, referred to as “DC” (“direct current”). In conventional pulse oximetry, the ratio (R) of AC and DC light absorption at two different wavelengths is calculated. The wavelengths of light are selected to maximize the difference between the ratios of the absorbances of O 2 Hb and deO 2 Hb (see Fig. 41.3 B ). The most commonly used wavelengths of light are 660 nm and 940 nm. At 660 nm, there is greater light absorption by deO 2 Hb than by O 2 Hb. At 940 nm, there is greater light absorption by O 2 Hb than by deO 2 Hb,
R=AC660/DC660AC940/DC940

where AC 660 , AC 940 , DC 660 , and DC 940 denote the corresponding AC and DC components of the 640-nm and 940-nm wavelengths.
The ratio R is then empirically related to O 2 saturation based on a calibration curve internal to each pulse oximeter ( Fig. 41.5 ). Each manufacturer develops its own calibration curve by having volunteers breathe hypoxic gas mixtures to create a range of S a O 2 values between 70% and 100%. The FDA recommends root mean square differences between measured values (S p O 2 ) and reference values (S a O 2 ) under normal conditions ranging from 70% to 100% S p O 2 of ≤3.0% for transmittance, wrap and clip pulse oximeter sensors and of ≤3.5% for ear clip and reflectance sensors. Most manufacturing literature reports an accuracy of ± 2% to 3% S p O 2 over this range. The dependence of S p O 2 on pulsatility may result in inaccurate estimates of S a O 2 when pulsatility is reduced or absent.

A pulse oximeter probe is composed of a light emitter and a photodetector. Transmission pulse oximetry involves the placement of the emitter and detector on opposite sides of the tissue being measured, usually of a finger. Reflectance pulse oximetry probes have the emitters and detector arranged on the same side. They are typically placed on the forehead. In a typical pulse oximeter, two light-emitting diodes (LEDs) are used to emit light at the two wavelengths. During operation, each LED is turned on and off in sequence. The photodetector measures transmission of light from each LED. When both LEDs are turned off, the photodetector measures ambient light and subtracts it from the signals obtained throughout the remainder of the cycle.
Pulse oximetry has been an integral component of intraoperative anesthetic management since the first anesthetic monitoring standards were introduced in 1986. It was adopted as a minimum monitoring standard by the ASA the same year and has subsequently been defined as a minimum standard for intraoperative monitoring by the World Federation of Societies of Anaesthesiologists and the World Health Organization (WHO). The use of pulse oximetry is a part of the WHO safe surgery checklist.
Multiwavelength pulse oximeters—that is, pulse oximeters with additional wavelengths of light to allow for the continuous noninvasive measurement of total hemoglobin concentration (S p Hb) —as well as concentrations of MetHb and COHb utilizing up to 12 wavelengths have been developed. S p Hb in the surgical and intensive care unit (ICU) settings has shown reasonable bias and precision when compared with laboratory measurements. However, few of the measurements were obtained in patients with Hb concentrations within the clinically relevant range of 6 to 10 mg/dL. Another limitation of S p Hb may be its absent or unreliable signal under conditions of low peripheral perfusion. To date, noninvasive COHb measurements have not shown enough precision to replace laboratory measurements. Accuracy has improved at hypoxic O 2 levels, but detecting COHb for S a O 2 less than 87% is still unreliable. Pulse oximetry-based MetHb measurements with a newer device are accurate, even under conditions of hypoxia.
Universal pulse oximetry has been recommended by the US Secretary of Health and Human Services to screen newborns for critical congenital heart disease. Use of a clinical algorithm based on detection of S p O 2 lower than 95% in either right upper or lower limbs, or more than a 2% difference led to a sensitivity of 75% in suspected cases and 58% in cases that were not suspected. When this modality is combined with a routine anomaly scan and newborn physical examination screening, 92% of critical congenital heart disease lesions can be identified.
Photoplethysmography . Along with measuring O 2 saturation, the pulse oximeter can also be used as a photoplethysmograph. Because the absorption of light is proportional to the amount of blood between the transmitter and photodetector, changes in the blood volume are reflected in the pulse oximeter trace ( Fig. 41.6 ). During anesthesia, the plethysmographic trace is affected by changes in blood volume pulsations, which depends on the distensibility of the vessel wall, as well as on the intravascular pulse pressure. Variations in the amplitude of the pulse oximetry plethysmographic waveform (ΔPOP) have been shown to predict fluid responsiveness in mechanically ventilated patients. An index derived from the percent difference between the maximum and minimum amplitudes of the plethysmographic waveform during a respiratory cycle (PVI, Pleth Variability Index) has been incorporated into a commercially available pulse oximeter and used to quantify ΔPOP and predict fluid responsiveness. A number of studies have shown it to be a reasonably reliable indicator of fluid responsiveness perioperatively and in critically ill patients. The technique does have greater reliability in mechanically ventilated patients compared with spontaneously breathing patients, but its accuracy may be compromised by the presence of cardiac arrhythmias. Its accuracy has also been shown to be better for 500 mL fluid challenges compared with 250 mL. Goal-directed fluid management based on PVI has been shown to improve outcomes in major abdominal surgery.

Limitations and Sources of Error
S p O 2 is an estimate of S a O 2 of circulating hemoglobin. As a consequence, it does not provide information about tissue oxygenation. Because S p O 2 is a measurement of functional and not fractional S a O 2 , the presence of other Hb variants can significantly affect its accuracy. The nonlinearity of the Hb dissociation curve prevents the detection of hyperoxia with S p O 2 for high S a O 2 , whereas for low saturations such as at altitude, small changes in P a O 2 can produce large changes in S p O 2 . There is significant variability in the actual in vivo Hb dissociation curve. Thus changes in S p O 2 are not necessarily well correlated with changes in S a O 2. These measurements illustrate that knowledge of the individual Hb dissociation curve is important for correct interpretation of S a O 2 and P a O 2 . More importantly, pulse oximetry does not provide information about ventilation or acid-base status.
A number of conditions can lead to inaccuracies in pulse oximeter readings ( Table 41.1 ). These conditions include decreased perfusion, motion artifact, venous pulsation, low S a O 2 , variant Hb species, the presence of intravascular dyes, and the presence of nail polish.
Source of Error | Effect on S p O 2 Relative to S a O 2 |
---|---|
Hypotension | ↓ |
Anemia | ↓ |
Polycythemia | No significant effect |
Motion | ↓ |
Low S a O 2 | variable |
Methemoglobinemia | ↓/↑ (S p O 2 approaches 85%) |
Carboxyhemoglobinemia | ↑ |
Cyanmethemoglobin | No significant effect |
Sulfhemoglobin | No significant effect |
Hemoglobin F | No significant effect |
Hemoglobin H | No significant effect |
Hemoglobin K | ↓ |
Hemoglobin S | No significant effect |
Methylene blue | ↓ |
Indigo carmine | ↓ |
Indocyanine green | ↓ |
Isosulfan blue | No significant effect/↓ |
Fluorescein | No significant effect |
Nail polish | Black, dark blue, purple ↓ |
Acrylic fingernails | No significant effect |
Henna | Red—No significant effect ↓ |
Skin pigmentation | At S a O 2 >80%, no significant effect At S a O 2 <80%, ↑ |
Jaundice | No significant effect |
Ambient light | No significant effect |
Sensor contact | ↓ |
IABP | ↑ |
Despite the recommendations of numerous boards and guidelines, there is no evidence that the use of pulse oximetry improves patient outcomes, not during transfers to ICUs nor in terms of improving patient mortality. There is evidence that pulse oximetry reduces the incidence of hypoxemia, and the duration and cost of ICU stay, suggesting it allows for early intervention.
The calibration of pulse oximeters is based on curves obtained in normal individuals under experimental conditions with S a O 2 as low as 70%. As such, pulse oximeters have limited accuracy for S a O 2 values less than 70%. Moreover, systematic errors in S p O 2 tend to increase as S a O 2 falls below 90%. At S a O 2 levels under 70%, a positive or negative bias of the S p O 2 value can be observed, depending on the manufacturer of the pulse oximeter. Manufacturers have developed pulse oximeters with increased accuracy at saturations as low as 60%. Preliminary data suggest that these probes may be useful in neonates with cyanotic congenital heart disease.
Hypoperfusion leads to a reduction in the amplitude of the pulsatile component of the light absorbance waveform, the essential signal for pulse oximetry, consequently giving rise to absent or inaccurate readings. Significantly erroneous reductions in S p O 2 may be observed for systolic blood pressures lower than 80 mm Hg. Motion artifact can produce considerable error in the pulse oximeter reading. Manufacturers have developed advanced proprietary signal processing algorithms that effectively filter out noise caused by motion.
With continued clinical use, the performance of the LEDs in the probe may be degraded, leading to inaccuracy in the S p O 2 value outside of the range specified by the manufacturer. These inaccuracies are expected to be more pronounced at lower saturations (i.e., <90%).
Venous pulsations may result in the detection of venous O 2 Hb saturation by the pulse oximeter, resulting in artifactual reduction of the presumed arterial S p O 2 being measured. Venous pulsations can be due to excessively tight placement of adhesive finger probes, severe tricuspid regurgitation, probe placement in dependent positions (e.g., forehead during Trendelenburg position), and possibly in distributive shock when vasodilation may result in physiologic arteriovenous shunting.
The presence of additional species of Hb can also generate erroneous pulse oximeter readings. As outlined earlier, the function of the pulse oximeter is predicated on the assumption that the only components present in the blood capable of absorbing light at the two wavelengths used are O 2 Hb and deO 2 Hb. Under normal circumstances, this assumption is valid, and the S p O 2 readings accurately reflect the S a O 2 . However, the presence of significant concentrations of other Hb species or substances absorbing light at the used wavelengths will lead to erroneous S p O 2 readings. As illustrated in Fig. 41.3 , both COHb and MetHb absorb light at one or both of the wavelengths used by the pulse oximeter. Accordingly, the presence of these Hb species will produce errors in S p O 2 . The absorption of light at 660 nm by COHb is similar to that of O 2 Hb. At 940 nm, COHb absorbs virtually no light. Thus, in a patient with carbon monoxide poisoning, the S p O 2 will be falsely elevated. MetHb absorbs a significant amount of light at both 660 nm and 940 nm. As a result, in its presence, the ratio of light absorption R ( Eq. 41.5 ) approaches unity. An R-value of 1 represents the presence of equal concentrations of O 2 Hb and deO 2 Hb and corresponds to an S p O 2 of 85%. Thus, in a patient with methemoglobinemia, the S p O 2 will be 80% to 85%, irrespective of the S a O 2 . SHb absorbs red light (660 nm) more than deoxyhemoglobin (HHb) or MetHb, and likely as much near the infrared spectrum. This results in S p O 2 values close to 85% in severe cases of sulfhemoglobinemia. Although newer generations of co-oximeters can detect SHb, most existing co-oximeters cannot; consequently, additional clinical laboratory testing may be required if sulfhemoglobinemia is suspected.
With normal S a O 2 , anemia has little effect on S p O 2 . However, in the presence of hypoxia, S p O 2 readings underestimate S a O 2 in anemic patients with true hypoxemia. Pulse oximeters are sufficiently accurate in adult patients with sickle cell disease, as well as in the presence of fetal Hb. However caution is warranted when using pulse oximetry in patients with sickle cell disease, because heme metabolism may result in elevated COHb. Some studies also suggest that S p O 2 may overestimate S a O 2 during vaso-occlusive crises. Another relevant point is that in patients with sickle cell disease, the affinity of O 2 for Hb is normal under normoxic conditions but becomes low during hypoxia.
A relatively uncommon cause for reduced S p O 2 readings is the presence of congenital variants of Hb. Some variants, such as Hb Bassett, Hb Rothschild, and Hb Canabiere, have a reduced affinity for O 2 , and changes in S p O 2 appropriately reflect changes in S a O 2 . Other variants, such as Hb Lansing, Hb Bonn, Hb Koln, Hb Cheverly, and Hb Hammersmith, have altered absorption spectra (closer to HHb) that result in low S p O 2 readings in the setting of normal S a O 2 .
The administration of intravenous dyes can result in inaccurate S p O 2 readings. Methylene blue leads to a transient, marked decrease in S p O 2 down to 65% due to its peak light absorption at 668 nm, which is very close to that of HHb. Indigo carmine and indocyanine green also artificially decrease S p O 2 measurements, although to a lesser extent than methylene blue, because they do not substantially absorb red light. Isosulfan blue can produce a prolonged reduction at higher doses.
Although all colors of nail polish can reduce the calculated value of S p O 2 , black, purple, and dark blue colors have the greatest effect. Nonetheless, the error generally remains within 2%. Depending on the brand of pulse oximeter, artificial acrylic nails may impair S p O 2 readings, although generally not to a clinically significant extent. Under conditions of normal S a O 2 , skin pigmentation has no effect on S p O 2 estimates. However, increased skin pigmentation is associated with S p O 2 values that overestimate S a O 2 by as much as 8% for S a O 2 less than 80%.
In the presence of severe hyperbilirubinemia (30 mg/dL or greater) caused by increased hemolysis or liver disease, the fraction of oxygenated hemoglobin (FO 2 Hb) may be falsely low by artifactual increase in MetHb and COHb, resulting in more accurate S p O 2 than FO 2 Hb measurements. Although earlier case reports and small studies have suggested that ambient light may interfere with the accuracy of S p O 2 readings, a large prospective study found no significant effect on S p O 2 accuracy with exposure to five types of light sources: quartz-halogen, incandescent, fluorescent, infant bilirubin lamp, and infrared. Infrared light pulses from image guidance systems used for navigational neurosurgery can interfere with pulse oximetry readings by causing decreased readings or disruptions in S p O 2 waveform detection. Different pulse oximeters show variable susceptibility to such interference. Shielding the probe with a single layer of aluminum foil can protect from this effect. Misplacement of the probe can allow for direct detection of LED light by the photodetector. This optical shunt gives rise to an S p O 2 reading of 85%.
In patients with intraaortic balloon pump support, S p O 2 accuracy depends on the brand of pulse oximeter used as well as the support ratio. Accuracy is generally reduced in the setting of higher support ratios. In patients with continuous flow ventricular assist devices, pulse oximetry may not be possible because of the absence of pulsatile flow. In these cases, the use of cerebral oximetry as an adjunct is advocated.
Pulse Oximeter Probes
Probes are usually applied to accessible body areas with high vascularity, such as the finger, nose, ear lobe, or forehead. The probes may be reusable or disposable. The advantages of reusable clip probes are that they are more cost-effective compared with disposable adhesive probes, can be rapidly applied, and are amenable to multiple applications in cases of low signal-to-noise ratio at the specified wavelengths. However, disposable probes allow for more secure placement (in case of patient movement) and provide the capability of monitor sites other than acral areas. Although reduced infectious transmission is a purported benefit of disposable probes, evidence is limited, and one must consider that pulse oximetry probes represent a small fraction of anesthetic equipment requiring decontamination. Accordingly, different probe models may be advantageous in specific conditions. For instance, ear and forehead probes may be more reliable during vasoconstriction compared with finger probes, given that the arterial vessels of such regions are less responsive to circulating catecholamines. As an example, in hypotensive patients that require vasopressors, ear and forehead probes may provide more accurate value of S p O 2 , because these areas are less likely to vasoconstrict with endogenous and exogenous catecholamines compared to fingers or toes. During hypothermia with secondary vasoconstriction, the forehead probe is more reliable compared with the finger probe.
Emerging Techniques: Pulse spectroscopy is a new technique that utilizes hundreds of wavelengths to assess normal and dysfunctional hemoglobins. Initial results are promising with accurate S p O 2 determinations, as well as COHb and MetHb assessment during normoxia and hypoxia.
Mixed Venous Oxygen Saturation
Physiologic Fundamentals
Mixed venous oxygen saturation ( <SPAN role=presentation tabIndex=0 id=MathJax-Element-8-Frame class=MathJax style="POSITION: relative" data-mathml='Sv¯O2′>Sv⎯⎯O2Sv¯O2
S v ¯ O 2
) is the O 2 saturation of blood at the proximal pulmonary artery. It has been a frequently monitored variable in critically ill patients, since it reflects the average O 2 saturation of the blood returning from the body to the right heart, weighted by the respective regional blood flows. As such, it is a measure of the balance between global oxygen delivery (DO 2 ) and global oxygen uptake ( <SPAN role=presentation tabIndex=0 id=MathJax-Element-9-Frame class=MathJax style="POSITION: relative" data-mathml='V˙O2′>V˙O2V˙O2
V ˙ O 2
), and a useful resuscitation target. Factors that influence the <SPAN role=presentation tabIndex=0 id=MathJax-Element-10-Frame class=MathJax style="POSITION: relative" data-mathml='Sv¯O2′>Sv⎯⎯O2Sv¯O2
S v ¯ O 2
can be illustrated through a derivation of the mixed venous O 2 content equation.
<SPAN role=presentation tabIndex=0 id=MathJax-Element-11-Frame class=MathJax style="POSITION: relative" data-mathml='V˙O2′>V˙O2V˙O2
V ˙ O 2
(mL/min) is defined as
V˙O2=10×Q˙T×(CaO2−Cv¯O2)
where <SPAN role=presentation tabIndex=0 id=MathJax-Element-13-Frame class=MathJax style="POSITION: relative" data-mathml='Q˙T’>Q˙TQ˙T
Q ˙ T
is the cardiac output (L/min), and <SPAN role=presentation tabIndex=0 id=MathJax-Element-14-Frame class=MathJax style="POSITION: relative" data-mathml='Cv¯O2′>Cv⎯⎯O2Cv¯O2
C v ¯ O 2
is the O 2 content of the mixed venous blood (mL/100 mL).
Rearranging Eq. (41.6) to solve for <SPAN role=presentation tabIndex=0 id=MathJax-Element-15-Frame class=MathJax style="POSITION: relative" data-mathml='Cv¯O2′>Cv⎯⎯O2Cv¯O2
C v ¯ O 2
yields
Cv¯O2=CaO2−V˙O2/Q˙T
The contribution of dissolved O 2 to the blood O 2 content is small. By expanding the definition of O 2 content ( Eq. 41.1 ) and ignoring the term for dissolved O 2 , Eq. (41.7) can be rewritten as
Sv¯O2=SaO2–V˙O2/(1.34⋅Hb⋅QT)
Normal values of <SPAN role=presentation tabIndex=0 id=MathJax-Element-18-Frame class=MathJax style="POSITION: relative" data-mathml='Sv¯O2′>Sv⎯⎯O2Sv¯O2
S v ¯ O 2
range between 65% and 80%. Values close to 40% are associated with tissue hypoxia, anaerobic metabolism, and lactate production. <SPAN role=presentation tabIndex=0 id=MathJax-Element-19-Frame class=MathJax style="POSITION: relative" data-mathml='Pv¯O2′>Pv⎯⎯O2Pv¯O2
P v ¯ O 2
can be derived from <SPAN role=presentation tabIndex=0 id=MathJax-Element-20-Frame class=MathJax style="POSITION: relative" data-mathml='Sv¯O2′>Sv⎯⎯O2Sv¯O2
S v ¯ O 2
values by utilizing the O 2 Hb dissociation curve adjusted to mixed-venous pH, PCO 2 , and temperature (see Fig. 41.2 ). The normal value of <SPAN role=presentation tabIndex=0 id=MathJax-Element-21-Frame class=MathJax style="POSITION: relative" data-mathml='Pv¯O2′>Pv⎯⎯O2Pv¯O2
P v ¯ O 2
is 40 mm Hg.
DO 2 is defined as
DO2=QT×CaO2
As is evident from Eq. (41.8) , a low <SPAN role=presentation tabIndex=0 id=MathJax-Element-23-Frame class=MathJax style="POSITION: relative" data-mathml='Sv¯O2′>Sv⎯⎯O2Sv¯O2
S v ¯ O 2
indicates either a reduction in DO 2 secondary to low S a O 2 , low Hb, or low <SPAN role=presentation tabIndex=0 id=MathJax-Element-24-Frame class=MathJax style="POSITION: relative" data-mathml='Q˙T’>Q˙TQ˙T
Q ˙ T
, or an increase in <SPAN role=presentation tabIndex=0 id=MathJax-Element-25-Frame class=MathJax style="POSITION: relative" data-mathml='V˙O2′>V˙O2V˙O2
V ˙ O 2
. The association among <SPAN role=presentation tabIndex=0 id=MathJax-Element-26-Frame class=MathJax style="POSITION: relative" data-mathml='Sv¯O2′>Sv⎯⎯O2Sv¯O2
S v ¯ O 2
, DO 2 , and <SPAN role=presentation tabIndex=0 id=MathJax-Element-27-Frame class=MathJax style="POSITION: relative" data-mathml='V˙O2′>V˙O2V˙O2
V ˙ O 2
can be illustrated by expressing <SPAN role=presentation tabIndex=0 id=MathJax-Element-28-Frame class=MathJax style="POSITION: relative" data-mathml='Sv¯O2′>Sv⎯⎯O2Sv¯O2
S v ¯ O 2
as a function of the O 2 extraction ratio, ERO 2 .
ERO2=V˙O2/DO2
Expanding the terms yields
ERO2=1−Cv¯O2/CaO2
Assuming that the O 2 dissolved in plasma is a negligible component to total O 2 content dissolved in the arterial or mixed venous blood, Eq. (41.11) can be rewritten as
ERO2=1−Sv¯O2/SaO2
For conditions in which the arterial blood is fully saturated, Eq. (41.12) may be further simplified:
ERO2=1–Sv¯O2
Solving for <SPAN role=presentation tabIndex=0 id=MathJax-Element-33-Frame class=MathJax style="POSITION: relative" data-mathml='Sv¯O2′>Sv⎯⎯O2Sv¯O2
S v ¯ O 2
yields
Sv¯O2=1–ERO2
Thus a reduction in <SPAN role=presentation tabIndex=0 id=MathJax-Element-35-Frame class=MathJax style="POSITION: relative" data-mathml='Sv¯O2′>Sv⎯⎯O2Sv¯O2
S v ¯ O 2
results from an increase in ERO 2 , from either an increased <SPAN role=presentation tabIndex=0 id=MathJax-Element-36-Frame class=MathJax style="POSITION: relative" data-mathml='V˙O2′>V˙O2V˙O2
V ˙ O 2
or a decreased DO 2 ( Fig. 41.7 ). A decrease in DO 2 occurs in conditions such as hemorrhagic or hypovolemic shock. An increase in <SPAN role=presentation tabIndex=0 id=MathJax-Element-37-Frame class=MathJax style="POSITION: relative" data-mathml='V˙O2′>V˙O2V˙O2
V ˙ O 2
may occur in conditions such as stress, pain, shivering, sepsis, and thyrotoxicosis. Conversely, an increase in <SPAN role=presentation tabIndex=0 id=MathJax-Element-38-Frame class=MathJax style="POSITION: relative" data-mathml='Sv¯O2′>Sv⎯⎯O2Sv¯O2
S v ¯ O 2
indicates either an increase in O 2 supply (elevated S a O 2 , Hb, or <SPAN role=presentation tabIndex=0 id=MathJax-Element-39-Frame class=MathJax style="POSITION: relative" data-mathml='Q˙T’>Q˙TQ˙T
Q ˙ T
) or a reduction in <SPAN role=presentation tabIndex=0 id=MathJax-Element-40-Frame class=MathJax style="POSITION: relative" data-mathml='V˙O2′>V˙O2V˙O2
V ˙ O 2
, as occurs during hypothermia.

V ˙ O 2
or decrease DO 2 will lead to a reduction in <SPAN role=presentation tabIndex=0 id=MathJax-Element-42-Frame class=MathJax style="POSITION: relative" data-mathml='Sv¯O2′>Sv⎯⎯O2Sv¯O2
S v ¯ O 2
. Conversely, conditions which decrease <SPAN role=presentation tabIndex=0 id=MathJax-Element-43-Frame class=MathJax style="POSITION: relative" data-mathml='V˙O2′>V˙O2V˙O2
V ˙ O 2
or improve DO 2 will lead to an increase in the <SPAN role=presentation tabIndex=0 id=MathJax-Element-44-Frame class=MathJax style="POSITION: relative" data-mathml='Sv¯O2′>Sv⎯⎯O2Sv¯O2
S v ¯ O 2
.
There are some subtle considerations in the interpretation of changes in <SPAN role=presentation tabIndex=0 id=MathJax-Element-45-Frame class=MathJax style="POSITION: relative" data-mathml='Sv¯O2′>Sv⎯⎯O2Sv¯O2
S v ¯ O 2
. At PO 2 values typical of venous blood, small increases in the fraction of inspired oxygen (F I O 2 ) can cause significant increases in <SPAN role=presentation tabIndex=0 id=MathJax-Element-46-Frame class=MathJax style="POSITION: relative" data-mathml='Sv¯O2′>Sv⎯⎯O2Sv¯O2
S v ¯ O 2
because of the shape of the O 2 Hb dissociation curve ( Fig. 41.2 ). Therefore, when <SPAN role=presentation tabIndex=0 id=MathJax-Element-47-Frame class=MathJax style="POSITION: relative" data-mathml='Sv¯O2′>Sv⎯⎯O2Sv¯O2
S v ¯ O 2
is tracked as a measure of cardiac function, changes in F I O 2 must be taken into account during its interpretation. In the setting of septic shock, impairment in O 2 extraction may yield normal <SPAN role=presentation tabIndex=0 id=MathJax-Element-48-Frame class=MathJax style="POSITION: relative" data-mathml='Sv¯O2′>Sv⎯⎯O2Sv¯O2
S v ¯ O 2
values, despite the presence of tissue hypoxia.
Direct measurement of <SPAN role=presentation tabIndex=0 id=MathJax-Element-49-Frame class=MathJax style="POSITION: relative" data-mathml='Sv¯O2′>Sv⎯⎯O2Sv¯O2
S v ¯ O 2
requires the insertion of a pulmonary artery catheter, a procedure associated with some morbidity. However, in most clinical situations, the O 2 saturation of a blood sample drawn from a central venous catheter will suffice. Thus the use of central venous saturation can be a surrogate for <SPAN role=presentation tabIndex=0 id=MathJax-Element-50-Frame class=MathJax style="POSITION: relative" data-mathml='Sv¯O2′>Sv⎯⎯O2Sv¯O2
S v ¯ O 2
. The saturation of blood at the level of a central venous catheter placed in the superior vena cava (S cv O 2 ) reflects the balance between O 2 supply and demand in the brain and upper extremities. Under normal physiologic conditions, S CV O 2 is typically 2% to 5% less than <SPAN role=presentation tabIndex=0 id=MathJax-Element-51-Frame class=MathJax style="POSITION: relative" data-mathml='Sv¯O2′>Sv⎯⎯O2Sv¯O2
S v ¯ O 2
, primarily because of the higher O 2 content of splanchnic and renal venous blood. However, during hemodynamic instability, as circulation is redistributed to the upper body, the relationship between <SPAN role=presentation tabIndex=0 id=MathJax-Element-52-Frame class=MathJax style="POSITION: relative" data-mathml='Sv¯O2′>Sv⎯⎯O2Sv¯O2
S v ¯ O 2
and S CV O 2 may reverse, and the difference between the two may increase significantly. Although trends in S CV O 2 may reflect those in <SPAN role=presentation tabIndex=0 id=MathJax-Element-53-Frame class=MathJax style="POSITION: relative" data-mathml='Sv¯O2′>Sv⎯⎯O2Sv¯O2
S v ¯ O 2
, the two measures cannot be used interchangeably.
Mixed venous CO 2 has been used to compute the arteriovenous CO 2 difference (ΔPCO 2 = P <SPAN role=presentation tabIndex=0 id=MathJax-Element-54-Frame class=MathJax style="POSITION: relative" data-mathml='v¯CO2′>v⎯⎯CO2v¯CO2
v ¯ C O 2
−P a CO 2 ). In conditions of steady-state CO 2 production, ΔPCO 2 changes inversely and nonlinearly with cardiac output as a result of the Fick equation. Accordingly, ΔPCO 2 is an indicator of the adequacy of cardiac output to provide adequate clearance of tissue CO 2 . However, because of several limitations, the parameter has not yet found widespread clinical use.
Measurement Principles
The measurement of venous O2 saturation can be performed intermittently by co-oximetry of blood sampled from the distal tip of a pulmonary artery catheter ( <SPAN role=presentation tabIndex=0 id=MathJax-Element-55-Frame class=MathJax style="POSITION: relative" data-mathml='Sv¯O2′>Sv⎯⎯O2Sv¯O2
S v ¯ O 2
) or a central venous catheter (S CV O 2 ). Falsely increased values can occur in the presence of a wedged pulmonary artery tip, mitral regurgitation, or left-to-right shunts. Venous saturations can also be measured continuously by spectrophotometry using specialized fiberoptic catheters, which transmit infrared light and detect the amount of light reflected from red blood cells. Specialized venous oximetry catheters are available for both pulmonary artery and central venous monitoring and have the advantage of providing continuous measurements of O 2 saturation. Their disadvantage is the cost. Although continuous venous oximetry catheters trend changes, their reported absolute values are not equivalent to concurrently obtained co-oximetry measurements.
Applications and Interpretation
In patients undergoing major abdominal or cardiac surgery, intraoperative reductions in <SPAN role=presentation tabIndex=0 id=MathJax-Element-56-Frame class=MathJax style="POSITION: relative" data-mathml='Sv¯O2′>Sv⎯⎯O2Sv¯O2
S v ¯ O 2
and S CV O 2 have been associated with postoperative complications. Protocol-based interventions that target specific values of <SPAN role=presentation tabIndex=0 id=MathJax-Element-57-Frame class=MathJax style="POSITION: relative" data-mathml='Sv¯O2′>Sv⎯⎯O2Sv¯O2
S v ¯ O 2
or S CV O 2 have been shown to reduce the length of stay, organ dysfunction, and mortality in patients undergoing major surgery and patients presenting with sepsis. Goal-directed therapy based on S cv O 2 has been advocated for the management of sepsis, and the implementation of such protocols is associated with improvements in mortality. The use of S CV O 2 as a therapeutic endpoint remains controversial for several reasons: S CV O 2 may be increased in sepsis because of impaired tissue O 2 extraction ; the increased cost associated with the use of S CV O 2 -measuring catheters ; and other measures, such as lactate clearance, are less costly while leading to similar outcomes. Furthermore, the management of sepsis without an S CV O 2 endpoint can yield equally favorable results. The difference between <SPAN role=presentation tabIndex=0 id=MathJax-Element-58-Frame class=MathJax style="POSITION: relative" data-mathml='Sv¯O2′>Sv⎯⎯O2Sv¯O2
S v ¯ O 2
and S CV O 2 has been proposed as a marker of complications after cardiac surgery.
Tissue Oxygenation
Arterial and venous O 2 saturations are measures of DO 2 and uptake by the whole body. Although useful, these global measures do not provide information regarding organ or tissue oxygenation, which reflects the important local balance between O 2 supply and demand. Regional O 2 balance can differ both among organs as well as within regions of the same organ. Current noninvasive methods for the assessment of microcirculatory oxygenation make use of reflectance spectroscopy using light either in the visible spectrum (VLS) or in the near-infrared spectrum (NIRS). A recent technique based on the protoporphyrin IX triplet state lifetime aims at the assessment of mitochondrial O 2 tension in vivo, and opens the prospect of future clinical monitoring (see Fig. 41.1 ).
Reflectance spectroscopy probes have light emitters and receivers positioned in line ( Fig. 41.8 ). When they are placed on a tissue surface, light transmission through the tissue is affected by reflection, absorption, and scatter. Reflection depends on the angle of incidence of the light beam and the wavelength of light, whereas scatter depends on the number and type of tissue interfaces. As previously outlined, the Beer-Lambert law relates the absorption of light by the tissue to the concentration of tissue chromophores, the extinction coefficient of each, and the pathlength of the light through the tissue. The predominant tissue chromophore is hemoglobin. The pathlength of the light is affected by both reflection and scatter, so it cannot be measured directly, but rather must be estimated. Most of the detected photons travel in an arc between the two detectors (see Fig. 41.8 ). The depth of penetration of the arc into the tissue is proportional to the wavelength of light and the distance between the transmitter and detector.

VLS makes use of white light with wavelengths of 500 to 800 nm, whereas NIRS employs light in the 700 to 1100 nm range. In general, the depth of penetration of VLS is less than that of NIRS, thus allowing superficial measurements of up to 16 mm and making it suitable for measurements of small subsurface volumes. NIRS can penetrate tissue to a depth of several centimeters and allows sampling of a larger volume of tissue. The O 2 saturation displayed is that of a volume of tissue. This volume includes arteries, capillaries, and veins, and has a predominantly venous weighting.
Clinical Applications
A number of applications have been described for VLS. Buccal microvascular Hb saturation has been associated with survival in patients with sepsis. VLS has also been used for monitoring flap viability following reconstructive surgery. During gastrointestinal and esophageal surgery, reductions in gastrointestinal tissue saturation, measured by VLS, have been associated with postoperative anastomotic complications. Endoscopic VLS differentiates between normal and ischemic areas of colon, and it may be useful for the diagnosis of mesenteric ischemia. In addition, mucosal O 2 saturation of the gastric conduit following esophagectomy is useful and explores the benefits of ischemic preconditioning.
The most widespread application of NIRS has been in cerebral oximetry, with probes placed on the forehead to measure frontal cortical oxygenation (rSO 2 ). Several NIRS systems are commercially available, with each manufacturer offering a different specific technology. As there is no gold standard for cerebral oximetry, it is difficult to compare the accuracy of the devices. Furthermore, each device has its own set of “normal” values. For this reason, acquisition of baseline values for each patient at the start of the procedure has been recommended. Typical values of rSO 2 range from 51% to 82% with a mean baseline of 66%. A reduction of rSO 2 below 20% to 25% of the baseline, or lower than an absolute value of 50%, is a suggested threshold for intervention.
The utility of cerebral oximetry has been explored in cardiovascular, abdominal, thoracic, and orthopedic surgery. In the cardiac surgical setting, intraoperative reductions in rSO 2 have been associated with early postoperative cognitive dysfunction and prolonged ICU and hospital lengths of stay. Baseline rSO 2 measurements have been associated with 30-day mortality after left ventricular assist device surgery. As a guide to therapy, interventions for rSO 2 reductions less than 75% of baseline in patients undergoing coronary artery bypass graft surgery have been shown to significantly reduce the incidence of major organ morbidity and mortality, as well as ICU length of stay.
During carotid endarterectomy, intraoperative reductions in rSO 2 correlate well with changes in transcranial Doppler measurements, electroencephalographic waveforms, and stump pressure , which are consistent with ischemia. Although some studies indicate that reductions in rSO 2 that are less than 20% of baseline are well tolerated, data are lacking for a clear rSO 2 threshold for carotid shunt placement. NIRS has also been preliminarily used during and following ICU open thoracoabdominal aortic aneurysm repair for continuous monitoring of spinal cord oxygenation .
In elderly patients undergoing major abdominal surgery, protocol-based intraoperative treatment of reductions in rSO 2 results in decreases in postoperative cognitive decline and hospital length of stay. In patients undergoing thoracic surgical procedures with single-lung ventilation, early postoperative cognitive dysfunction is directly related to intraoperative exposure time to rSO 2 less than 65%. Patients undergoing shoulder surgery in the beach chair position have a lower baseline rSO 2 and a larger number of episodes of cerebral desaturation, although the clinical implications of these findings are unclear.
Shock is a condition in which patients may have inadequate regional perfusion in the setting of normal global perfusion parameters. In such circumstances, the use of NIRS to monitor tissue perfusion holds some promise. Values obtained by the application of an NIRS probe to the thenar eminence (S t O 2 ) discriminate between healthy volunteers and patients with shock. Furthermore, in patients with major trauma who present with shock, S t O 2 values can identify patients who proceed to develop multiorgan dysfunction or actually die.
Overall, available information is still limited to warrant clinical decision making exclusively based on NIRS measurements. Currently there is insufficient evidence to support the use of perioperative NIRS monitoring in adults to reduce short-term or mild postoperative cognitive dysfunction, postoperative stroke, delirium, or death. Of note, in a 2015 retrospective study, patients who self-identified as African American had lower rSO 2 compared with those who self-identified as Caucasian, a finding attributed to light attenuation by skin pigmentation. Further investigations are necessary to improve our understanding of NIRS and its relevance for clinical management.
Capnometry and Capnography
General Concepts
The presence CO 2 in exhaled breath reflects the fundamental physiologic processes of ventilation, pulmonary blood flow, and aerobic metabolism. Its continued monitoring ensures the anesthesiologist of correct placement of an endotracheal tube (ETT) or laryngeal mask airway (LMA), as well as the integrity of a breathing circuit. Exhaled CO 2 provides information primarily on ventilation. It is also used to estimate the adequacy of cardiac output. In combination with P a CO 2 , exhaled CO 2 can be used to estimate the ratio of physiologic dead space (V D ) to tidal volume (V T ) by using the Bohr equation :
VDVT=(PaCO2−PE¯CO2PaCO2)
where <SPAN role=presentation tabIndex=0 id=MathJax-Element-60-Frame class=MathJax style="POSITION: relative" data-mathml='PE¯CO2′>PE⎯⎯⎯CO2PE¯CO2
P E ¯ CO 2
is the mixed expired CO 2 partial pressure, such as measured in exhaled air collected in a mixing bag chamber, or computed from a volumetric capnogram. The ability to detect and quantify CO 2 is a crucial component of respiratory monitoring in anesthesia and critical care medicine.
Considerable confusion arises from inconsistent and interchangeable terminology as applied to medical CO 2 gas analysis. In a very general sense, capnometry refers to the measurement and quantification of inhaled or exhaled CO 2 concentrations at the airway opening. Capnography , however, refers not only to the method of CO 2 measurement, but also to its graphic display as a function of time or volume. A capnometer is simply a device that measures CO 2 concentrations. A capnometer may display a numeric value for inspired or exhaled CO 2 . A capnograph , however, is a device that records and displays CO 2 concentrations, usually as a function of time. A capnogram refers to the graphic display that the capnograph generates. Fig. 41.9 illustrates a typical CO 2 concentration profile for three breaths as a function of time.

Measurement Principles
Various methods exist for detecting and quantifying CO 2 concentrations in respiratory gases, such as mass spectrometry, Raman spectrometry, or gas chromatography. The most commonly used method in clinical environments relies on nondispersive infrared absorption. With this technique, a beam of infrared light is passed through a gas sample, and the resulting intensity of the transmitted light is measured by a photodetector. Gaseous CO 2 absorbs light over a very narrow bandwidth centered around 4.26 μm. Its presence in the sample cell decreases the amount of infrared light at this wavelength that reaches the detector in proportion to its concentration. Because the absorption spectrum for CO 2 partially overlaps with the spectra of other gaseous species commonly encountered in anesthesia (i.e., water and nitrous oxide ), infrared filters and compensation algorithms are used to minimize this interference and improve accuracy.
Most capnometers rely on infrared-light source that is focused on a chopper disk that rotates at approximately 60 revolutions per second. The chopper allows the beam to be alternately directed through (1) the sample cell with the gas to be analyzed and (2) a reference cell with no detectable CO 2 . In addition, the light source is completely blocked at various points during the revolution of the chopper disk. The photodetector and associated circuitry process these three signals to estimate the changes in CO 2 concentration continuously in the sample cell. Alternatively, CO 2 concentration may be estimated with solid-state technology, using a beam splitter instead of a chopper wheel. The splitter allows for the measurement of infrared energy at wavelengths within and outside the absorption spectrum of CO 2 .
Capnometers fall into two general categories: sidestream (diverting) and mainstream (nondiverting). Sidestream analyzers are more frequently used in clinical environments. Their CO 2 sensors are physically located away from the airway gases to be measured. Sidestream analyzers incorporate a pump or compressor that aspirates gases into a sample cell located at the unit’s console ( Fig. 41.10 A ). Typical tubing length for this aspiration may be 6 feet, and gas withdrawal rates may vary from 30 to 500 mL/min. This lost gas volume may need to be considered during closed-circuit anesthesia or during ventilation of neonates and infants. The volume can be returned to the circuit, or it can be routed to a scavenger to prevent contamination of the environment with anesthetic or waste gases. Gases must also pass through various filters and water traps before they are presented to the sample cell. Sidestream capnometers have a transport delay time corresponding to the rate at which gas is sampled and the washout of the analyzing chamber (see Fig. 41.10 B ). The capnograms generated by sidestream analyzers also have an associated rise time, defined as the time required for the analyzer to respond to a sudden change in CO 2 concentration. By convention, this is usually the time interval required for the for the analyzer output to change from 10% to 70% of its final value. ∗
∗ Rise time may also be defined as the time required for the analyzer output to change from 10% to 90% of its final value.
, Typical rise times for commercially available capnometers range from 10 to 400 ms, and they can depend on the rotation of the chopper wheel, the rate of gas aspiration, the volume of aspiration tubing and water traps, and the dynamic response of the infrared filters and other electronics.
With mainstream analyzers, the sample cell is placed directly into the patient’s breathing circuit. Thus the inspiratory or expiratory gases pass directly through the infrared light path (see Fig. 41.10 C ). An advantage of mainstream analyzers is that they have no delay time (see Fig. 41.10 D ). Moreover, their rise time is generally faster than that of sidestream analyzers. A disadvantage is the potential increase in dead space, although recent developments in solid-state electronics have made this much less of an issue. In addition, the sample cell is usually heated to 40°C to minimize the condensation of water vapor, which can bias the measurement. This increase in temperature, combined with the proximity of the sensor to the patient’s airway, can potentially increase the risk of facial burns.
Time Capnogram
The simplest and most widely used form of display for exhaled CO 2 is the time capnogram . The time capnogram displays both inspiratory and expiratory phases. Fig. 41.9 shows a typical time capnogram for three breaths. The expiratory phase is divided into three distinct components. Phase I corresponds to the exhalation of dead space gas from the central conducting airways or any equipment distal to the sampling site, which ideally should have no detectable CO 2 (i.e., partial pressure of CO 2 , PCO 2 ∼ 0). During phase II, a sharp rise in PCO 2 to a plateau indicates the sampling of transitional gas between the airways and alveoli. The plateau region of the capnogram, phase III, corresponds to the PCO 2 in the alveolar compartment. For a lung with relatively homogeneous ventilation, phase III is approximately flat throughout expiration. In fact, various mechanisms contribute to the slight upsloping of CO 2 concentration versus time during phase III. Most of these mechanisms reflect a heterogeneous distribution of ventilation-perfusion ( <SPAN role=presentation tabIndex=0 id=MathJax-Element-61-Frame class=MathJax style="POSITION: relative" data-mathml='V˙/Q˙’>V˙/Q˙V˙/Q˙
V ˙ / Q ˙
) or alveolar CO 2 partial pressure (P A CO 2 ) throughout the lung. Well-ventilated and well-matched <SPAN role=presentation tabIndex=0 id=MathJax-Element-62-Frame class=MathJax style="POSITION: relative" data-mathml='V˙/Q˙’>V˙/Q˙V˙/Q˙
V ˙ / Q ˙
regions tend to have lower PCO 2 and shorter time constants, and they empty earlier during the expiratory phase. Less well-ventilated and poorly matched <SPAN role=presentation tabIndex=0 id=MathJax-Element-63-Frame class=MathJax style="POSITION: relative" data-mathml='V˙/Q˙’>V˙/Q˙V˙/Q˙
V ˙ / Q ˙
regions have higher CO 2 levels, and they empty later in the expiratory cycle. Respiratory pathologies associated with an increase in ventilation heterogeneity, such as asthma, chronic obstructive pulmonary disease (COPD), or acute lung injury, yield a steeper upslope of phase III. Interventions that improve ventilation homogeneity, such as positive end-expiratory pressure (PEEP) or bronchodilators, flatten phase III. Mechanical disturbances may also be present during phase III, reflecting processes such as spontaneous breathing efforts, cardiogenic oscillations, or surgical manipulation ( Fig. 41.11 ). Following phase III, a sharp downstroke of PCO 2 occurs as fresh inspired gas moves past the sampling site and washes out the remaining CO 2 . This is referred to as the beginning of phase 0 by some authors, or phase IV by others. Occasionally, a sharp upstroke in PCO 2 is observed at the very end of phase III, which is termed phase IV or IV′, depending on the author. This upstroke probably results from the closure of lung units with relatively low PCO 2 and allows for regions of higher CO 2 to contribute a greater proportion of the exhaled gas to be sampled. Additional insights into various abnormalities in ventilation or perfusion are also obtained by trending time capnograms over many breaths for long periods ( Fig. 41.12 ).


The term “end-tidal” CO 2 (P ET CO 2 ) generally refers to the final value of the exhaled PCO 2 curve, at the very end of the expiratory phase. The method used to determine this number is not universal, and varies according to the manufacturer of the particular capnograph in use. For example, P ET CO 2 may simply be (1) the PCO 2 value just before inspiration, (2) the largest PCO 2 value during a single exhalation cycle, or (3) the PCO 2 value at a specified time in the capnogram averaged across several breaths. If P ET CO 2 is measured during a reasonably flat and undistorted phase III, it may be well correlated with P a CO 2. 170 This may not be the case if the duration of phase III is truncated, or if CO 2 is measured from gas that is contaminated with room or O 2 -enriched air (i.e., during spontaneous breathing with a nasal cannula or facemask). Potential causes of increased or decreased P ET CO 2 are listed in Table 41.2 . In healthy individuals with homogeneous ventilation, the difference between P a CO 2 and P ET CO 2 is usually less than 5 mm Hg, thereby expressing the equilibration between alveolar and pulmonary capillary blood. Several disease states compromise this equilibration and produce increased P a CO 2 − P ET CO 2 difference ( Box 41.2 ). There are situations in which P ET CO 2 can be greater than P a CO 2 , especially in the presence of severe ventilation heterogeneity and lung units with very low <SPAN role=presentation tabIndex=0 id=MathJax-Element-64-Frame class=MathJax style="POSITION: relative" data-mathml='V˙/Q˙’>V˙/Q˙V˙/Q˙
V ˙ / Q ˙
. For steady-state conditions, the P ET CO 2 usually reflects the relative balance between CO 2 production and alveolar ventilation.
↑P ET CO 2 | ↓P ET CO 2 |
---|---|
↑CO 2 Production and Delivery to the Lungs
| ↓CO 2 Production and Delivery to the Lungs
|
↓Alveolar Ventilation
| ↑Alveolar Ventilation
|
Equipment Malfunction
| Equipment Malfunction
|
Increased ventilation-perfusion heterogeneity, particularly with high V/Q regions
Pulmonary hypoperfusion
Pulmonary embolism
Cardiac arrest
Positive pressure ventilation (especially with PEEP)
High-rate low-tidal-volume ventilation
Volume Capnogram
Although time capnography is relatively straightforward to monitor in clinical environments, a major limitation of the technique is its lack of information regarding respiratory flows or volumes. The volume capnogram is a graphic display of CO 2 concentration or partial pressure versus exhaled volume. The inspiratory phase is not defined in a volume capnogram. Similar to its temporal counterpart, it is also partitioned into three distinct phases (I, II, and III) corresponding to anatomic dead space, transitional, and alveolar gas samples ( Fig. 41.13 ). However, it possesses several advantages over the time capnogram. First, it allows for estimation of the relative contributions of anatomic and alveolar components of physiologic dead space. Second, it is more sensitive than the time capnogram in detecting subtle changes in dead space that are caused by alterations in PEEP, pulmonary blood flow, or ventilation heterogeneity ( Fig. 41.14 ). Finally, the numeric integral of PCO 2 as a function of volume allows for determination of the total mass of CO 2 exhaled during a breath and provides for the estimation of <SPAN role=presentation tabIndex=0 id=MathJax-Element-65-Frame class=MathJax style="POSITION: relative" data-mathml='V˙CO2′>V˙CO2V˙CO2
V ˙ CO 2
.

V CO 2
), and the mixed expired CO 2 fraction or partial pressure to be used in the Bohr equation ( Eq. [41.15] ) based on the division of the exhaled CO 2 volume by the exhaled tidal volume. Area Y represents wasted ventilation due to alveolar dead space, while area Z corresponds to wasted ventilation due to anatomic deadspace (V D aw). Thus areas Y + Z represent the total physiologic dead space. The volume capnogram can also be plotted as a PCO 2 versus exhaled volume curve. FETCO 2 , Fraction of end-tidal carbon dioxide.

Blood Gas Analysis
Physiologic Fundamentals
Arterial blood gas analysis is used to assess oxygenation, ventilation, and acid-base status. This section focuses on the use of arterial blood gases to assess oxygenation and ventilation. For a discussion of acid-base status, see Chapter 48 , “Perioperative Acid-Base Balance.”
Oxygenation is reflected in the P a O 2 , which is a function of the alveolar partial pressure of O 2 (P A O 2 ) and the efficiency of O 2 transfer from alveoli to the pulmonary capillary blood. In healthy adults breathing room air at sea level, P a O 2 ranges between 80 and 100 mm Hg. The normal value of P a O 2 decreases with increasing age and increasing altitude. Hypoxemia is defined as a P a O 2 less than 80 mm Hg. There are five physiologic causes of hypoxemia: (1) hypoventilation, (2) <SPAN role=presentation tabIndex=0 id=MathJax-Element-67-Frame class=MathJax style="POSITION: relative" data-mathml='V˙/Q˙’>V˙/Q˙V˙/Q˙
V ˙ / Q ˙
mismatching, (3) right-to-left shunt, (4) diffusion limitation, and (5) diffusion-perfusion mismatch. The first three causes explain the majority of hypoxemia in the perioperative setting. Reduced inspired PO 2 (e.g., in faulty closed or partially closed anesthesia breathing circuits or at high altitude) is an additional cause of hypoxemia.
These factors produce hypoxemia by affecting different steps of O 2 transport from the environment to the arterial blood. A low inspired PO 2 , as well as hypoventilation, reduces the P A O 2 . <SPAN role=presentation tabIndex=0 id=MathJax-Element-68-Frame class=MathJax style="POSITION: relative" data-mathml='V˙/Q˙’>V˙/Q˙V˙/Q˙
V ˙ / Q ˙
mismatch, right-to-left shunt, and alveolar diffusion limitation affect the efficiency of O 2 exchange. Diffusion limitation plays a role in conditions that thicken the alveolar-capillary barrier, such as interstitial lung diseases, and in hypoxemia induced by exercise or altitude. In the clinical setting, impairment of diffusion of O 2 or CO 2 rarely occurs to a significant extent. The remainder of this section focuses on <SPAN role=presentation tabIndex=0 id=MathJax-Element-69-Frame class=MathJax style="POSITION: relative" data-mathml='V˙/Q˙’>V˙/Q˙V˙/Q˙
V ˙ / Q ˙
mismatch and right-to-left shunt.
<SPAN role=presentation tabIndex=0 id=MathJax-Element-70-Frame class=MathJax style="POSITION: relative" data-mathml='V˙/Q˙’>V˙/Q˙V˙/Q˙
V ˙ / Q ˙
mismatch is the most common cause of hypoxemia in the clinical setting. Ventilation and perfusion are nonuniformly distributed throughout the normal lung, with worsening mismatch in the setting of lung disease, general anesthesia, and mechanical ventilation. Areas with low or zero <SPAN role=presentation tabIndex=0 id=MathJax-Element-71-Frame class=MathJax style="POSITION: relative" data-mathml='V˙/Q˙’>V˙/Q˙V˙/Q˙
V ˙ / Q ˙
yield low end-capillary PO 2 , whereas areas with normal or high <SPAN role=presentation tabIndex=0 id=MathJax-Element-72-Frame class=MathJax style="POSITION: relative" data-mathml='V˙/Q˙’>V˙/Q˙V˙/Q˙
V ˙ / Q ˙
produce higher end-capillary PO 2 . However, because of the plateau of the O 2 Hb dissociation curve (see Fig. 41.2 ), the normal and high <SPAN role=presentation tabIndex=0 id=MathJax-Element-73-Frame class=MathJax style="POSITION: relative" data-mathml='V˙/Q˙’>V˙/Q˙V˙/Q˙
V ˙ / Q ˙
regions are limited in the extent to which they increase the O 2 content and compensate for the low <SPAN role=presentation tabIndex=0 id=MathJax-Element-74-Frame class=MathJax style="POSITION: relative" data-mathml='V˙/Q˙’>V˙/Q˙V˙/Q˙
V ˙ / Q ˙
regions ( Fig. 41.15 ). Consequently, <SPAN role=presentation tabIndex=0 id=MathJax-Element-75-Frame class=MathJax style="POSITION: relative" data-mathml='V˙/Q˙’>V˙/Q˙V˙/Q˙
V ˙ / Q ˙
mismatch results in hypoxemia.
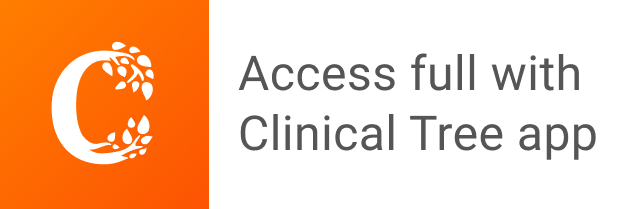