Chapter 26
Respiratory Anatomy, Physiology, Pathophysiology, and Anesthetic Management
Anatomy of the Respiratory System
Nose
The principal arterial supply of the nasal fossae comes from the ophthalmic arteries through the anterior and posterior ethmoid branches and from the internal maxillary artery through the sphenopalatine arteries. Because of the location of the interior maxillary artery, it is sometimes ligated for the treatment of persistent nosebleed. The veins accompany the arteries; the ethmoid veins open into the superior sagittal sinus, and the nasal veins drain into the ophthalmic veins and then into the cavernous sinuses. Infections in the nose can result in meningitis because of this venous communication between the intracranial and intranasal circulation. The sensory nerves from the upper respiratory tract come from the ophthalmic nerve and the maxillary nerve (both are branches of cranial nerve V). Lymphatic drainage from the cavities of the nose is via the deep cervical lymph nodes adjacent to the internal jugular vein.
The hairs at the entrance to the nostrils are of minor importance to filtration because they remove only large particles. Much more important is the removal of particles by turbulent precipitation. Air passing through the nasal passageways hits many obstructions including the septum, the turbinates, and the pharyngeal wall. When the inspired air is forced to change direction, the inhaled particles cannot change course as rapidly, and they become embedded in the mucus-covered surfaces of these processes. The particles trapped in the mucus are moved by the cilia either to the naris or posteriorly to the pharynx to be expectorated or swallowed. This occurrence is important because it denies entry of infectious, carcinogenic, or irritating substances. Nasal filtration is extremely effective for particles above 10 µm and less than 1 nm, but filtration efficiency is inverse to particle size for particles that are between 10 nm and 1 µm.1
Larynx
The adult larynx extends from vertebrae C3 to C6 and is a protective structure that prevents aspiration during swallowing; vocalization evolved secondarily. The larynx consists of one bone, nine cartilages (Table 26-1), ligaments, muscles, and membranes.
Laryngeal Cartilages
In adults, the narrowest portion of the larynx is the cricoid opening between the vocal cords; in children younger than 10 years, the narrowest part is just below the vocal cords at the cricoid cartilage. This anatomic difference is of clinical significance: a tube with adequate clearance through the vocal cords may create mucosal pressure at the level of the cricoid ring. For this reason, traditionally, uncuffed endotracheal tubes have been used in children less than 8 to 10 years of age. Although an uncuffed tube may offer advantages in terms of positioning,2 it is becoming more commonplace to use an adjusted-size cuffed tube in pediatric patients for the added assurance of tidal volume delivery and to reduce the necessity to change the tube because of incorrect sizing.3
Movements of the Vocal Cords
The true vocal cords are fibromembranous folds attached anteriorly to the thyroid cartilage and posteriorly to the arytenoids. The focal points of movement are the arytenoid cartilages, which rotate and slide up and down on the sloping cricoid cartilage. The muscles controlling laryngeal movement (Box 26-1) are most conveniently thought of as pairs having opposing actions. The laryngeal inlet is closed by the aryepiglottic muscle and opened by the thyroepiglottic muscle. The cricoid opening is dilated by the posterior cricoarytenoid muscles and closed by the interarytenoid muscles assisted by the lateral cricoarytenoid muscles. The cricothyroid muscles lengthen the true vocal cords, and the thyroarytenoid muscles shorten them. Both sets of muscles can alter the tension on the vocal cords and are important for determining the pitch of the voice.4
Nerve Supply to the Larynx
The inferior (or recurrent) laryngeal nerves arise from the two vagus nerves at different levels. The left nerve descends with the vagus and then loops around the arch of the aorta to come back up to the neck. The right nerve travels with the vagus nerve as far as the subclavian artery; it loops around this artery and then comes back up the neck. The recurrent laryngeal nerve supplies sensation to the larynx below the level of the vocal cords and innervates all the muscles of the larynx except the cricothyroid and part of the interarytenoid muscles. Damage to the recurrent laryngeal nerve(s) during surgery on the neck or from airway devices or anesthetic blocks can lead to unilateral or bilateral vocal cord paralysis with hoarseness or dyspnea, respectively.5,6 Blood supply to the larynx is provided by the superior thyroid artery (a branch of the external carotid artery) and the inferior thyroid artery (a branch of the thyrocervical trunk, which arises from the subclavian artery).
Trachea
The trachea has a diameter of approximately 2.5 cm and is supported by incomplete rings of cartilage that open posteriorly and prevent tracheal collapse under the negative pressure generated during spontaneous respiration. The trachea extends down to the level of T4-T5, where the carina is located. This level corresponds anteriorly to the angle of Louis on the sternum, which is the articulation of the second rib. The trachea is not a rigid structure; it expands and contracts to accommodate head and neck movement. In an intubated patient, flexion of the neck elevates the carina. As a result, the endotracheal tube moves downward and endobronchial intubation may result. During extension of the head and neck, the trachea moves downward, the endotracheal tube moves upward, and extubation can occur. In pediatric patients, the range of this movement was demonstrated to increase with patient age and height.7 The apparent movement of the endotracheal tube in relation to head flexion may seem paradoxical; the mnemonic “the hose follows the nose” can be used as a memory aid. Neck rotation to the left or right tends to cause tracheal elevation and risk of endobronchial intubation.
Bronchi
At the carina, the trachea divides into the right and left bronchi (Figure 26-1). The cellular structure begins to change at this point from columnar to cuboidal epithelium, and the cartilaginous rings thin into plates once the bronchi penetrate the lungs. From the carina, the bronchi branch off at slightly different angles. The right bronchus takes a less acute angle from the trachea, about 25 degrees, whereas the left bronchus takes off at 45 degrees. Also, the right mainstem bronchus is wider and shorter (2 cm) than the left one (4 cm).8 Because the right bronchus is more nearly vertical than the left, the tendency is much greater for endotracheal tubes, suction catheters, or aspirated foreign materials to enter the right side after passing the carina. Additionally, the beveled tip of an endotracheal tube makes right-sided intubation more likely. The side hole (Murphy’s eye) near the end of the endotracheal tube allows the delivery of gas if the beveled tip of the tube is closely opposed to the similarly angled right main bronchus.
Each successive division of the airways is referred to as a generation, with the mainstem bronchi representing the first generation, the lobar bronchi representing the second, and so on. The lobar bronchi divide into the third generation of airways, called segmental bronchi, which deliver ventilation to the various bronchopulmonary segments of the lung. There are 10 bronchopulmonary segments in each lung, but on the left, the apical and posterior segments and the anterior basal and medial basal segment pairs each arise from a single bronchial branch (Box 26-2). Therefore, only eight third-generation bronchi are found on the left. Segments whose names contain the word basal are located adjacent to the diaphragm. The bronchopulmonary segments create distinct anatomic and functional units. The segments are separated by connective tissue, so gas-exchange properties or pathology tend to be isolated to a segment. A bronchopulmonary segment also can be excised as a unit.
With succeeding generations, the histology of the airways changes, in a progression characterized by thinning of the walls, to transition to the gas-exchanging morphology of the respiratory zone (Box 26-3). The terminal bronchioles divide into the respiratory bronchioles that are perfused by the pulmonary circulation and are the first place in the airway at which exchange of gas with the blood occurs. These airways are characterized by occasional outpouching of alveoli, or air sacs. The respiratory bronchioles divide into several alveolar ducts that lead to circular spaces called atria. Each atrium opens into two to five alveolar sacs, which are spaces lined by alveoli. The terminal airways are very small, and their walls are no longer tented open by cartilage but rather by connection with the adjacent matrix of pulmonary parenchyma in which they are situated. For this reason, they are prone to closure from compression of the pulmonary tissue during respiration or if emphysema, for example, expands the volume of adjacent air spaces and compresses the airways. The lung volume at which small airways tend to close is called the closing volume. In those with obesity and chronic obstructive pulmonary diseae (COPD), the closing volume increases into the range of normal tidal breathing such that some airways close before the intended tidal volume has been expired. Small pores in the alveoli, known as the pores of Kohn, serve to allow collateral gas flow between alveoli and provide a mechanism of relief from gas stagnation from airway closure.9
Respiratory Zone
The respiratory bronchioles and alveolar ducts, sacs, and alveoli comprise the respiratory zone, the area where gas exchange takes place. All parts of the airway prior to this (nose to terminal bronchioles) conduct gas without exchanging gas with the blood and are referred to as the conducting zone. Some refer to the respiratory bronchioles and alveolar ducts where limited gas exchange takes place as the transitional zone because structures here function both to conduct gas and also to participate in some gas exchange. The alveoli are the air sacs that are tightly packed and closely approximated with pulmonary capillaries. The typical maximum number of approximately 300 million alveoli is reached by age 9 years. The alveoli are characterized by very thin walls composed of squamous epithelium. There are three types of cells that form the alveoli: type I pneumocytes, which are the structural cells; type II pneumocytes, which produce surfactant to reduce alveolar collapse from surface tension; and type III pneumocytes, which are macrophages. The average alveolar diameter is approximately 250 µm; therefore the total surface area available for gas exchange is 60 to 80 m2.10
Pulmonary Hila and Coverings
The nerve supply to the bronchi and lungs arises chiefly from the sympathetic nerves and the vagus nerve (which supplies sensory and parasympathetic innervation). All conduits to the lung pass through the hilum, which is the connection of the mediastinum to the pedicle of each lung. The structures included in each hilum include the mainstem bronchus, pulmonary artery and vein, bronchial arteries and veins (which drain into the azygos system), lymphatics, lymph nodes, pulmonary nerve plexuses, and pulmonary ligament. All of this is surrounded by connective tissue. The serous membrane covering the lung is called the pleura. The parietal pleura lines the chest wall, mediastinum, and diaphragm, and at the hilum is then reflected back to cover the lungs as the visceral pleura. Between these two layers is a potential space called the pleural cavity. The touching surfaces of the two layers of pleura are kept slippery by a small amount of serous fluid. Certain conditions can result in occupation of the pleural space by liquids or gas (Table 26-2) and may affect ventilation and lung expansion. Infected intrapleural blood can clot and organize to form a fibrothorax, which must be peeled from the surface of the lung (in a procedure called lung decortication) so the lung can reexpand.
TABLE 26-2
Conditions That Affect the Pleural Space
Material in Pleural Space | Medical Name |
Air | Pneumothorax |
Air under pressure | Tension pneumothorax |
Blood | Hemothorax |
Serous fluid | Pleural effusion |
Pus | Empyema or pyothorax |
Organized blood clot | Fibrothorax |
Lymph | Chylothorax |
Mediastinum
The mediastinum is the region between the two pleural sacs. It lies roughly in the center of the thoracic cavity but is slightly displaced to the left by the presence of the heart. Therefore the left lung represents 45% of the total lung capacity (TLC), whereas the right lung represents 55%. Perforation of the larynx, trachea, pharynx, or esophagus, which sometimes occurs during esophagoscopy, bronchoscopy, or traumatic intubation, can produce mediastinitis, a life-threatening infection of an area containing the trachea, esophagus, and major blood vessels and heart. The mediastinum is divided into four divisions separated by the pericardium (Table 26-3). Common procedures involving the mediastinum include coronary artery bypass, cardiac valve replacement, aortic aneurysm repair, thymectomy for myasthenia gravis, resection of tumors, and mediastinoscopy for diagnosis and staging of cancer.
TABLE 26-3
Subdivision | Location | Contents |
Superior | Above level of the sternal angle, extending superior to the thoracic inlet | Thymus, esophagus, trachea, great vessels |
Anterior | Between sternum and pericardium | Thymus |
Posterior | Between vertebral column and posterior pericardium | Esophagus, thoracic aorta, thoracic duct |
Middle | Between anterior and posterior divisions, bounded laterally by the parietal pleura | Heart, distal trachea, mainstem bronchi, and great vessel trunks |
Mechanics of Breathing
Lung Compliance
Lung compliance is defined as the change in volume divided by the change in pressure (V/P). For a given change in pressure, a more compliant lung has a greater change in volume than a less compliant one. Figure 26-2 shows pressure-volume relationships for a lung. As with many concepts in respiratory physiology, the reader must make the jump from considering the application to a single alveolus (which aids in understanding) to conceptualizing the overall average state in the pulmonary system, which involves many regions existing along a continuum of conditions. In considering lung compliance, the curve in Figure 26-2 represents the collective contribution of alveoli that are almost collapsed at the beginning of inspiration, alveoli that are distended, and alveoli that exist at various intermediate volumes.
Static effective compliance describes the pressure-volume relationship for a lung when air is not moving; that is, reflecting compliance of the lung and chest wall alone. Static compliance is decreased by conditions that make the lung difficult to inflate, such as fibrosis, obesity, vascular engorgement, edema, acute respiratory distress syndrome (ARDS), and external compression (e.g., that caused by tight dressings or a surgeon leaning on the patient’s chest). Static compliance is increased by emphysema, which destroys the elastic tissue of the lung. This makes the emphysematous lung easier to inflate. The problem with emphysema is not inflation but rather deflation, because the loss of elastic tissue results in small airway collapse as the lung deflates, which causes gas trapping. It is important to note that compliance changes as lung volume changes. In other words, compliance is volume dependent. Figure 26-2 shows that the lung is less compliant both at very low and at very high lung volumes. Alveoli require greater pressure to be inflated when they are almost empty or almost full, respectively. When an alveolus is collapsed, a great increase in pressure is necessary for inflation to begin. Observe in Figure 26-2 that the slope of the inspiratory curve is less at both low volumes and very high volumes. At low volumes, it takes more energy (more negative pressure, i.e., less compliant) to begin to expand the lungs. At high volumes, the alveoli are almost at capacity, and further changes in pressure result in less change in volume (less volume per given pressure = less compliant). As you follow the curve along the expiratory side, notice that an initial increase in pressure (with slower volume change) as the chest wall relaxes is followed by a smooth reduction in volume back to the resting level. Lung compliance results from the interplay of various factors that tend to either expand the lungs or restrict lung expansion. Much of the energy required to expand the lungs, particularly at low volumes, is created by surface tension in the fluid lining of the alveoli, which tends to attract the alveoli toward a smaller volume. Perhaps counterintuitively, a lung filled with fluid (and therefore without an air/fluid interface and the resulting surface tension) has a very high compliance—it requires much less energy to expand. Although not a high-fidelity measurement, static effective compliance can be calculated easily using the following equation:
Dynamic compliance is the compliance of the lung while the air is moving. Dynamic compliance is influenced by the forces involved in static compliance as well as the effects of airway resistance. Airway obstruction (e.g., that caused by bronchospasm or the presence of foreign bodies in the airway) can greatly decrease dynamic compliance.11 Dynamic compliance is calculated as the tidal volume divided by peak inspiratory pressure − PEEP. Many modern anesthesia ventilators can calculate and trend compliance through tracing of pressure-volume curves.
Lung Elastic Recoil
Although the law of Laplace has traditionally been applied to understanding alveolar pressure-tension relationships, there is controversy over whether alveoli should be treated as spherical (and thus subject to the law) or not. Geometricians postulate that closely packed alveoli would not maintain the shape of spheres, but rather of polyhedrons because their sides would be flattened against each other. The classical application of LaPlace described the concept of alveoli as distinct balloon-like structures wherein pressure differentials can cause small alveoli to collapse and expel their gas into larger ones. The fact that alveoli are not individually suspended, but rather part of a connective tissue mesh, argues against this concept. The presence of pores of Kohn also argues against this theory, because the pores allow pressure equalization between adjacent alveoli. Connective tissue and elastic forces probably play the most important role in preventing alveolar closure.12
In any case, it is clear that surfactant is crucial for reducing surface tension and preventing collapse of alveoli and, perhaps more importantly, small airways. The cylindrical shape of airways lends to the application of the law of LaPlace to the role of surfactant in these structures. Surfactant probably also helps prevent fluid bridging (connection of fluid lining from opposite sides of an airway at low volumes), which could impair gas flow.13 In the fetus, surfactant is not produced until approximately 28 to 32 weeks of gestation and does not reach mature levels until approximately 35 weeks’ gestation. The lack of surfactant is the prevalent cause of respiratory distress syndrome (RDS) in premature infants. Formation of surfactant can be hastened by the administration of glucocorticoids (particularly a steroid that crosses the placenta, such as betamethasone) to the parturient mother when premature delivery is threatened or imminent. The direct administration of synthetic surfactant to the airways of premature newborns has also greatly reduced the incidence of RDS. Amniocentesis is sometimes performed to determine whether mature surfactant levels are present in the premature fetus. The ratio of lecithin to sphingomyelin (the L/S ratio) indicates the amount of mature surfactant (dipalmitoyl lecithin) in proportion to the amount of surfactant precursor (sphingomyelin).
Respiratory Pressure
The difference between intraalveolar pressure and intrapleural pressure is called the transpulmonary pressure. Under normal circumstances, the pleural pressure is always slightly negative, owing to the opposing forces of lung tissue contraction and chest wall expansion. The intrapleural pressure becomes slightly more negative during inspiration and slightly less negative during expiration. The transpulmonary pressure fluctuates as the alveolar pressure oscillates between slightly negative during inspiration to slightly positive during expiration, returning to zero whenever airflow is stopped at end-inspiration or end-expiration (Figure 26-3). During normal inspiration, intraalveolar pressure fluctuates only by 1 to 2 mmHg. Therefore very little pressure is applied during eupneic ventilation. During maximal expiration with a closed glottis (such as during coughing), intraalveolar pressure may exceed 100 mmHg, whereas during obstructed inspiration, it may be reduced to as low as −90 mmHg. During the first few breaths of life, a newborn can attain an intraalveolar pressure from −40 to −60 mmHg as the fluid-filled lungs are first aerated. In conditions of low lung compliance, more work is required to expand the lung, and the transpulmonary pressure rises (greater difference between intrapleural and intraalveolar pressure required to increase the lung volume).
Resistance to Breathing
where ν = velocity of fluid flow, d = diameter of the vessel, p = density of the fluid, and η = viscosity of the fluid. This version of the formula would apply to flow through a tube (such as the airways). In open systems, length is substituted for diameter. When the inertial forces of density, velocity of flow, and diameter increase, Reynolds number increases. Increasing viscosity of the fluid reduces the product. Products up to 2000 predict laminar flow; above 4000 predict turbulent flow, and a transitional area exists when results are between those numbers.
The clinical application of these concepts resides in strategies to reduce airflow resistance. Bronchodilators will reduce resistance to airflow by increasing the radius of the pathway, as predicted by Poiseuille. Selection of an endotracheal tube size may confer greater or lesser resistance, based on the length and (much more significantly) the internal diameter of the tube. Clinical application of Reynolds number suggests that lower velocity (lower inspiratory flow or lower inspiratory:expiratory ratio) and lower density would promote laminar flow and create lower ventilating pressures. The reduction in density is the conceptual basis for combining helium with oxygen (“heliox”) to improve pulmonary gas distribution in obstructive lung disease.14
Lung Volumes
The following discussion of lung volumes uses the parameters of a normal 70-kg male. Table 26-4 gives an overview of related terms and normal values. The amount of gas that enters and leaves the body with each eupneic breath is approximately 350 to 500 mL and represents the tidal volume (VT). The minute volume (MV) equals VT multiplied by the respiratory rate. However, because some ventilation occupies the conducting zone, only a portion of the minute ventilation (e) participates in gas exchange. The amount of alveolar ventilation in a minute equals VT minus anatomic dead space (the volume of the conducting airways, which is approximately 2 mL per kg of body weight) multiplied by the ventilatory rate. The rate of alveolar ventilation will be indirectly proportional to the arterial CO2 tension. The residual volume (RV) is the volume of gas left in the lung after a maximal exhalation (approximately 1.5 L). The RV cannot be removed from the lungs voluntarily and is important because it is a component of the functional residual capacity, which represents alveolar gas used for oxygenation of the blood between breaths or in periods of apnea. The expiratory reserve volume is the volume of gas expelled from the lungs during a maximal forced exhalation, starting at the end of a normal tidal exhalation. The inspiratory reserve volume is the volume of gas inhaled into the lungs during a maximal forced inhalation, starting at the end of a normal tidal inspiration (2.5 L).
The sum of the four basic lung volumes is the total lung capacity (TLC). Several types of lung capacity measures exist, each of which is the sum of two or more lung volumes. TLC is the volume of air in the lungs after a maximal inspiratory effort (approximately 6 L in a 70-kg adult). The vital capacity is the amount of air that can be forcibly exhaled from the lungs after a maximal inspiratory effort (approximately 4.5 L). The functional residual capacity (FRC) is the volume of gas contained in the lungs after normal quiet expiration. It is the sum of the RV and expiratory reserve volume (approximately 3 L). The inspiratory capacity is the volume of air inhaled into the lungs during a maximal inspiratory effort that begins at FRC (approximately 3 L). Figure 26-4 gives a graphic representation of lung volumes and normal flow measurements.
Closing volume describes the phenomenon during exhalation when small airways collapse, hindering further emptying of lung units distal to them. Closing volume is defined as the volume above residual volume where small airways close, whereas closing capacity describes the absolute volume of gas in the lung when small airways close (the closing capacity is the sum of the closing volume plus the residual volume). The closing volume increases from approximately 30% of the TLC at age 20 years to approximately 55% at age 70 years. Certain conditions increase the closing volume, such as supine positioning, pregnancy, obesity, COPD, congestive heart failure, and aging.15 In abnormal conditions, if the closing volume exceeds the FRC, airway closure occurs during tidal breathing, resulting in poorly ventilated or unventilated alveoli and intrapulmonary shunting. The shearing forces of repetitive airway opening and closing also leads to airway injury.16 Measurement of closing volume analyzing washout characteristics of inert gas is a more sensitive indicator of small airway disease (such as from smoking) than is measurement of spirometry.15
Gas Exchange in the Lungs
Regional Distribution of Alveolar Ventilation
Gravity and other factors influence ventilation and perfusion such that both are unevenly distributed throughout the lungs. In the normal upright lung, the alveoli at the base are more compliant than those at the apex, meaning that those at the base exhibit a greater change in volume with each breath. This is considered to be a result of the effect of gravity on the interconnected parenchyma of the lung, whereby the greatest “pull” is exerted on the more superior portions of lung. Therefore, the alveoli in those areas are held in a more open state even at rest, whereas alveoli at the base are more compressed. During inspiration then, the alveoli at the bases are able to accept more new gas (Figure 26-5). Alterations to the resting volume or impingements on lung compliance may change this relationship. Pregnancy or obesity may hinder expansion of lower units or may cause compression that results in collapsed dependent areas. In that case, other lung areas become the most compliant. The sigmoidal shape of the compliance curve (see Figure 26-2) reveals that alveoli become less compliant at higher volumes (e.g., in nondependent alveoli at high lung volume) and also at very low volumes (e.g., in dependent alveoli at very low resting lung volumes).
Alveolar Oxygen and Carbon Dioxide Levels
Approximately 21% of dry atmospheric air is O2; therefore, at the standard barometric pressure of 760 mmHg, Po2atm equals 0.21 × 760 mmHg, or 160 mmHg. Only 0.04% of atmospheric air is CO2, so Pco2atm = 0.3 mm Hg. As the inspired air passes through the upper airways, it is heated to body temperature and humidified to a relative humidity of nearly 100%. The partial pressure of water vapor at body temperature is a fairly constant 47 mmHg. The Po2 of inspired air (Pio2) saturated with water vapor at standard atmospheric pressure = 0.21 × (760 mmHg − 47 mmHg), or 149 mmHg.
Thus during the breathing of atmospheric air, when Paco2 is 40 mmHg and the RQ is 0.8, then Pao2 = (0.21 × [760 mmHg − 47 mmHg]) − 40 mmHg/0.8 = 99 mmHg. Therefore, using the alveolar air equation, one can calculate the Pao2 if the atmospheric pressure, inspired O2 concentration, and Paco2 (which is approximately equal both to the end-tidal Pco2 and the arterial Pco2 [Paco2]) are known, because water vapor pressure and RQ are fairly constant. If the inspired O2 concentration differs from that of room air, then that fraction replaces the 0.21. Pao2 is less than Pio2 because the CO2 is delivered to the alveoli by the pulmonary blood flow at the same time that O2 is taken up from the alveoli. Therefore Paco2 divided by the RQ approximates the amount of O2 that was removed from the alveoli by the pulmonary capillary blood flow.
Pulmonary Blood Flow
The sympathetic nervous system has some influence on pulmonary vascular resistance, as do certain substances circulating in the pulmonary blood. Pulmonary vascular resistance is increased by norepinephrine, serotonin, histamine, hypoxia, endothelin, leukotriene, thromboxane, prostaglandin (e.g., PGF2α), and hypercapnia.17 It is decreased by prostacyclin analogs (e.g., epoprostenol), endothelin receptor antagonists (e.g., bosentan), phosphodiesterase type 5 inhibitors (e.g., sildenafil),18 acetylcholine,19 and isoproterenol (minimal effect).20 Short-term or limited-use medications to reduce pulmonary vascular resistance include inhaled nitric oxide, calcium channel blockers (e.g., amlodipine), and adenosine.18
Influences on Pulmonary Blood Flow
Although pulmonary vessels have less muscular content than systemic arteries, the low pressure of the system makes pulmonary blood flow very sensitive to small changes in arterial tone. Unlike the systemic circulation, where hemodynamic influences are more global, pulmonary blood flow is more prevalently regulated locally by changes in oxygen and carbon dioxide tension. In contrast to the systemic circulation, high oxygen tension and hypocapnea vasodilates pulmonary vessels (which helps those vessels pick up more oxygen), whereas hypercarbia and acidosis cause vasoconstriction. Having the strongest influence on pulmonary local regulation, blood flow to hypoxic or atelectatic alveoli is actively diverted at a precapillary site by a process known as hypoxic pulmonary vasoconstriction. This decreases blood flow away from focal diseased areas of the lung and improves matching of ventilation and perfusion. See Chapter 27 for a discussion of the significance of hypoxic pulmonary vasoconstriction during pulmonary surgery.
Relationship of Pulmonary Blood Flow and Ventilation
Although there may be a general increase in perfusion from top to bottom of the lung, it should be noted that gravity alone does not determine physiologic perfusion. If it did, then it would be observed that the greatest blood flow in the body would be in the lower extremities, with the least in the head, a cogent point of explanation offered by Levitzki.21 Gravity interacts with elastic forces to influence ventilation and with vessel recruitment to alter distribution of perfusion. There is evidence that regional perfusion zones may be situated with the greatest blood flow in the lower, core areas of the lungs, with zones 2 and 1 more resembling concentric spheres radiating toward the periphery.22 Nonetheless, the classic explanation of ventilation-perfusion zones described by West in 1964 serves as a useful model for considering the relationships between ventilation and perfusion,23 and more specifically, the conditions under which intraalveolar pressure may impede vascular flow.24 In this model, regions of the lung are considered in zones, according to the relative intravascular and intraalveolar pressure (Figure 26-6).
The dependent portion of the lung, where both pulmonary arterial and venous pressures exceed alveolar pressure, is known as zone 3. This zone represents continuous blood flow, and it is in this zone that the tip of a pulmonary artery catheter should lie, for example, to ensure continuous communication with the left heart. Alveoli in this zone rest at a lower volume than in zone 1, and so they have greater compliance and represent the greatest proportion of ventilation in the lung; however, the perfusion is also greatest here, and so there is no obstruction to blood flow. West later described a fourth zone in the most dependent portions of lung, wherein extravascular pressure from mechanical compression or interstitial fluid compresses the vessels and occludes their flow.25
Pulmonary Edema
The normal distance for diffusion from the alveolar air space into the pulmonary capillary blood cells is less than 1 micrometer. The gas must traverse the surfactant layer, the flat alveolar type I cells, the interstitial space, the endothelial cells that make up the wall of the pulmonary capillary, a minute amount of plasma, and then finally the membrane of the red blood cell. The pulmonary system is designed to allow free passage of gases across this series of structures, collectively called the respiratory membrane. However, that inherent “leakiness” does predispose this area to unintended movement of fluid.
There is a fine balance between the plasma colloid oncotic pressure, which tends to hold fluid in the pulmonary capillaries, and the capillary hydrostatic pressure, interstitial fluid colloid oncotic pressure, and negative interstitial fluid pressure, which all tend to favor fluid movement into the interstitial space. In normal circumstances, the net of these forces favors movement into the interstitium, helping to divert fluid from the adjacent “leaky” capillaries away from the alveoli and thereby prevent accumulation there.26 Although the interstitium has a large compliance for removing accumulating transudated fluid, derangements in the factors above can lead to fluid accumulation in the interstitium or alveoli and disrupt gas exchange.
A condition that occasionally occurs during emergence from anesthesia is postobstructive pulmonary edema, also referred to as negative-pressure pulmonary edema (NPPE). After extubation, if the patient experiences laryngospasm and then attempts forceful inhalation against the closed glottis, the drastic decrease in intrathoracic pressure pulls fluid from the pulmonary capillaries. The onset of pulmonary edema is rapid and relatively easy to treat. The symptoms resolve rapidly; in most cases, patients are discharged within 24 hours. Treatment includes removing the precipitating condition (relieving airway obstruction) and general supportive measures: oxygen, maintenance of a patent airway, noninvasive continuous positive airway pressure (CPAP), and intubation with PEEP if required to maintain oxygenation. Unlike pulmonary edema related to fluid overload, NPPE does not call for diuretic therapy. However, many reported cases do include the use of controversial treatments of diuretics and corticosteroids.27 The three mainstays of treatment remain treatment of the precipitating condition, normalization of ventilation and oxygenation, and reduction of lung congestion and fluid. Treatment summary is in Box 26-4.
Ventilation-Perfusion Relationships in the Lung
Although distribution of ventilation normally decreases moving from dependent to nondependent regions of the lung, the accompanying decrease in perfusion is even greater; therefore the ratio, , increases as measured progressively from dependent to nondependent lung areas (Figure 26-7). Also,
varies: in alveoli that are ventilated but not perfused,
equals 0, so
equals infinity (i.e., dead space); in alveoli that are perfused but not ventilated,
equals 0, so
equals 0 (i.e., a shunt). Similarly, alveoli that are ventilated but poorly perfused are described as “deadspace-like,” whereas alveoli that are perfused but poorly ventilated are termed shuntlike
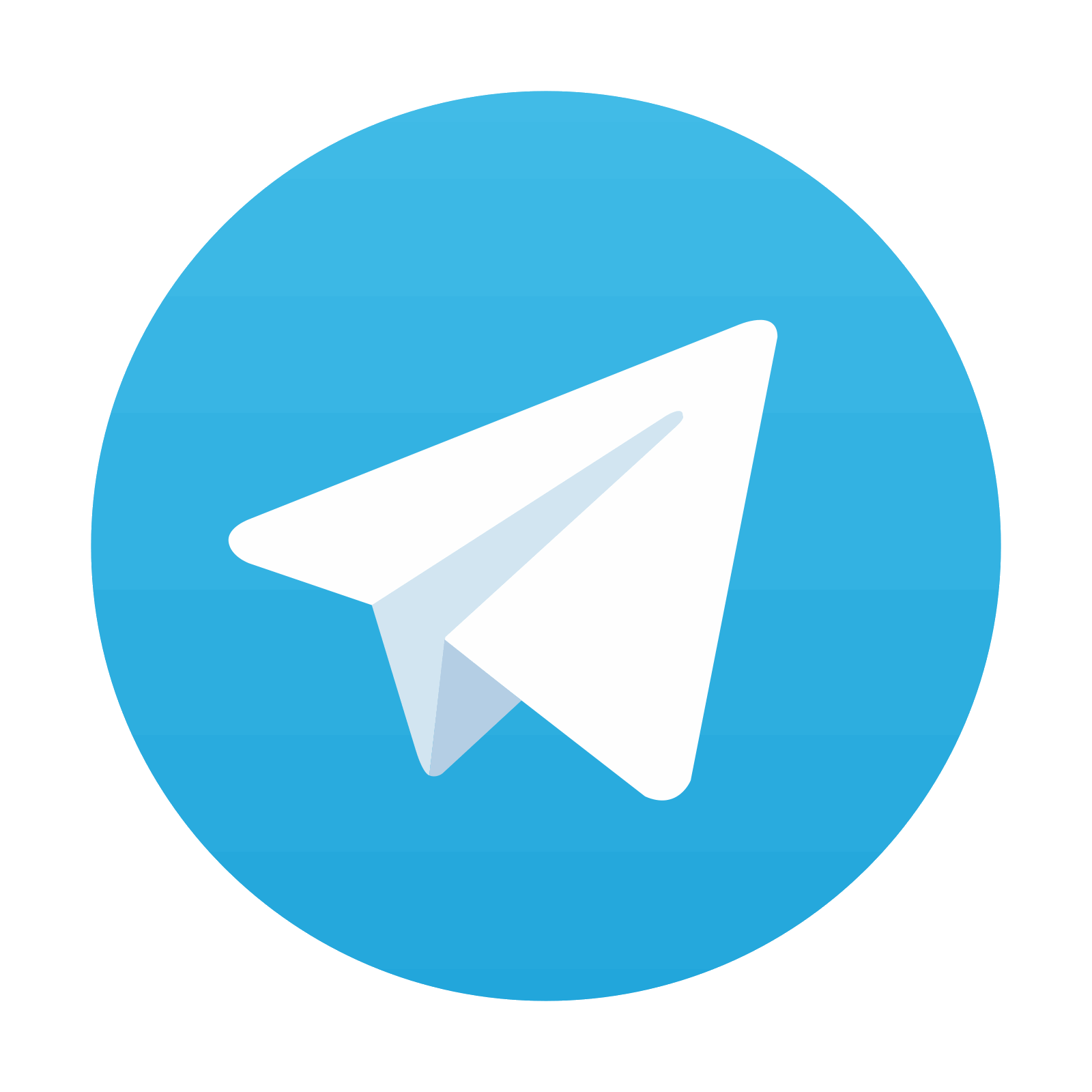
Stay updated, free articles. Join our Telegram channel
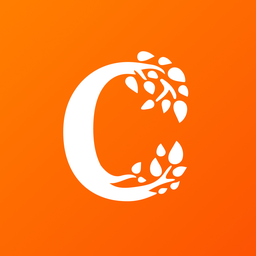
Full access? Get Clinical Tree
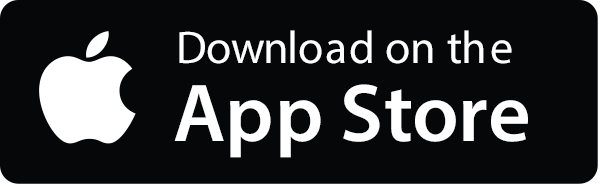
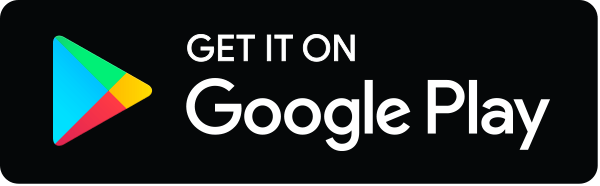