Key Points
- ▪
The incidence of perioperative acute kidney injury (AKI) (previously referred to as acute renal failure) varies, depending on the definition used.
- ▪
Although uncommon, AKI requiring dialysis is associated with extremely high morbidity and mortality rates.
- ▪
The mechanism for perioperative AKI is complex and commonly involves multiple factors including ischemia-reperfusion injury, inflammation, and toxins.
- ▪
Repeated direct perioperative assessments of renal hemodynamics or tubular function are impractical; therefore indirect assessments, such as trends of serum creatinine concentrations, are the best practical perioperative tools to assess renal function.
- ▪
Intraoperative urine formation depends on many factors and is not validated as a measure of the risk of postoperative renal dysfunction. Yet postoperatively, patients with low intraoperative urine output may develop renal dysfunction. Therefore urine output should be carefully monitored in the intraoperative setting.
- ▪
Early biochemical markers for kidney injury may soon become new tests that can provide prompt clinical information.
- ▪
As part of preoperative risk assessment, both serum creatinine and proteinuria can provide important and useful information.
- ▪
Intraoperative hypotension and hypovolemia are significant risk factors for AKI.
- ▪
With regard to fluid management, the use of balanced salt solutions may reduce the risk of AKI. Volume overload is a risk factor for adverse outcomes in patients with AKI and may influence concentrations of conventional markers of kidney function such as serum creatinine.
- ▪
Renal replacement therapy may be indicated for severe AKI: at present, data does not support the use of one modality over another.
Acknowledgment
The editors, publisher, and authors, Drs. Kathleen D. Liu, Daniel H. Burkhardt III, and Rupert M. Pearse, would like to thank Drs. Mark Stafford-Smith and Andrew Shaw for their contribution to this chapter in the prior edition of this work. It has served as the foundation for the current chapter.
Introduction and Acute Kidney Injury Definitions
Acute kidney injury (AKI) (previously known as acute renal failure) is characterized by rapid decline in the glomerular filtration rate (GFR) and the accumulation of nitrogenous waste products (blood urea nitrogen [BUN] and creatinine). AKI occurs in approximately 5% to 25% of all hospitalized patients, depending on the precise definition used for AKI, and with more frequent rates in patients who are critically ill in the intensive care unit (ICU) (also see Chapter 17 ). AKI is also a serious perioperative complication for patients undergoing major surgery. As the incidence of AKI varies by the definition used, the mortality of AKI ranges from 10% to 35% for mild AKI, whereas AKI in the ICU setting is associated with a 50% to 80% mortality rate. However, supportive care with dialysis has reduced mortality from AKI. Whereas the mortality rate of oliguric AKI was 91% during World War II, mortality declined to 53% during the Korean War with the provision of dialysis. AKI requiring dialysis develops in 1% to 7% of patients after cardiac or major vascular surgery and is strongly associated with morbidity and mortality in this context.
Perioperative renal failure was long defined as a requirement for postoperative dialysis. However, this concept has evolved during the past several years. First, because the implications of requiring postoperative dialysis are quite different for a patient starting with a normal baseline renal function compared with one starting with advanced chronic kidney disease and because the criteria for the use of dialysis are not standardized, the usefulness of dialysis alone to define AKI has been questioned. Second, studies are difficult to compare because of the use of nonstandard definitions for AKI. For example, in one review of 28 studies, definitions for perioperative AKI varied. Third, consensus definitions that focus on small changes in serum creatinine and on changes in urine output to define AKI have received widespread adoption. This last conclusion is based on the recognition that small changes in renal function directly relate to an increased risk of death.
As a result, recent consensus criteria are being used to define AKI in both the perioperative and other medical settings. The first proposed consensus criteria were the RIFLE ( R isk, I njury, F ailure, L oss, E nd-stage) kidney disease criteria developed by the Acute Dialysis Quality Initiative ( Table 42.1 ). These have subsequently undergone two modifications by the Acute Kidney Injury Network and in the Kidney Disease: Improving Global Outcomes (KDIGO) AKI guidelines. As detailed in Table 42.1 , the central components of these criteria are the focus on relative and absolute changes in creatinine from a baseline value and the definition of several degrees of AKI severity. Consequently, milder AKI (e.g., KDIGO, stage 1 disease) will be more common than stage 3 disease and will also be associated with a lower mortality rate. These criteria have also proposed definitions for AKI based on urine output. Overall, although there are studies demonstrating that AKI defined based on urine output is associated with adverse outcomes in the critical care setting and is more common than AKI defined based on creatinine, the urine output criteria are not as well validated. At present, there is no clear method to correct urine output for morbid obesity; in addition, urine output may be unmeasurable if a urinary bladder catheter is not present. Not surprisingly, AKI by urine output criteria is substantially more common than AKI by creatinine-based criteria; in a study of more than 4000 subjects undergoing major noncardiac surgery, the incidence of AKI increased from 8% to 64% when urine output criteria were incorporated. Although each AKI stage was associated with an increased risk of death, the association with mortality was attenuated in this analysis when urine output criteria for AKI were used. Finally, it should be noted that the importance of oliguria (<0.5 mL/kg/h) as a predictor of creatinine-based AKI is less well established in the perioperative setting than in other clinical settings. In a recent large single-center observational study, urine output of less than 0.3 mL/kg/h during major abdominal surgery was associated with an increased risk of perioperative AKI (defined as a 0.3 mg/dL rise in serum creatinine within 48 hours or a 50% increase over 7 days from baseline). However, urine output within the 0.3 to 0.5 mL/kg/h range was not associated with creatinine-based AKI.
RIFLE | AKIN | KDIGO | ||||
---|---|---|---|---|---|---|
Class | SCr | Stage | SCr | Stage | SCr | Urine Output a |
Risk | Increase in SCr to >1.5× baseline | 1 | Increase in SCr ≥0.3 mg/dL or to ≥1.5×-2× baseline | 1 | Increase in SCr ≥0.3 mg/dL within 48 h or to ≥1.5×-2× baseline, which is known or presumed to have occurred within the past 7 days | Urine output <0.5 mg/kg/h for >6 h |
Injury | Increase in SCr to >2× baseline | 2 | Increase in SCr to >2×-3× baseline | 2 | Increase in SCr to >2×-3× baseline | Urine output <0.5 mg/kg/h for >12 h |
Failure | Increase in SCr to >3× baseline, or increase of ≥0.5 mg/dL to absolute value of ≥4 mg/dL | 3 | Increase in SCr to >3× baseline, or increase of ≥0.5 mg/dL to absolute value of ≥4 mg/dL, or need for RRT | 3 | Increase in SCr to >3× baseline, or increase to absolute value of ≥4 mg/dL, or need for RRT; in pediatric patients eGFR <35 mL/min/1.73 m 2 | Urine output <0.3 mg/kg/h for >12 h or anuria for >12 h |
Loss | Need for RRT >4 weeks | |||||
End stage | Need for RRT >3 months |
Additional challenges for the identification of AKI in the intraoperative setting include large blood volume loss and fluid shifts, which may artificially dilute serum creatinine. Unlike the postoperative or critical care setting where renal monitoring can involve periodic evaluation of kidney function under relatively stable conditions, intraoperative renal monitoring involves a briefer unstable period, often involving significant blood loss, major fluid shifts, wide hemodynamic fluctuations, and even direct compromise to renal artery blood flow. Therefore the anesthesia provider is likely the first monitor (in a sense) required for preserving renal function by recognizing and treating factors that may contribute to or exacerbate AKI; for example, the toxic effects of aminoglycosides and iodinated contrast materials are exacerbated by intravascular volume depletion.
As medical populations shift toward older and more critically ill patients undergoing increasingly high-risk procedures, patients are at an increased risk of AKI in the perioperative setting, and the role of the anesthesia provider becomes even more critical. Indeed, a recent study of dialysis after elective major surgery suggests that the incidence of dialysis-requiring AKI is rising from 0.2% in 1995 to 0.6% in 2009, with the majority of the increase occurring after vascular and cardiac surgery. Although ischemic causes may be primarily responsible for perioperative AKI, the successful development of renoprotective strategies has not occurred. Furthermore, other pathophysiologic contributors to perioperative AKI may include contrast-induced nephropathy, pigment nephropathy (e.g., hemoglobin, myoglobin), cholesterol emboli (e.g., atheroembolic renal disease), aminoglycoside toxicity, and sepsis. Animal studies of such pure nephropathies treated with logical renoprotective interventions often demonstrate success; unfortunately, this success has not extended to equivalent renoprotection in humans. It may not be surprising that a specific treatment for a pure nephropathy nonselectively applied to a mixture of nephropathies, variably expressed in different patients, would be unsuccessful. Postoperative AKI, rather than being a single entity, is likely a mosaic of several pure nephropathies, each of varying importance for a particular patient and procedure ( Fig. 42.1 ).

Pathophysiologic Processes of Ischemic Acute Kidney Injury
In general, the causes for AKI can be divided into prerenal, intrinsic renal, and postrenal sources. In the perioperative setting, patients may be at increased risk for prerenal AKI, either attributable to volume depletion or to exacerbation of associated chronic prerenal physiologic conditions, such as congestive heart failure, which may be exacerbated by volume overload. Intraoperatively, hypotension due to vasodilation and negative inotropy/chronotropy from anesthetic agents may lead to prerenal physiology. Depending on the nature of the surgical procedure, the patient may also be at increased risk of postrenal AKI attributable to obstruction of the ureters, bladder, or urethra. However, the primary cause of perioperative AKI is acute tubular necrosis (ATN). Defining the cause of AKI is also critical because treatment of the underlying cause is critical for the reversal of AKI and potential renal recovery.
The two primary mechanisms of ATN are ischemia-reperfusion and nephrotoxic effects, with three sources of insult common to many surgical procedures during which postoperative AKI is prevalent: hypoperfusion, inflammation, and atheroembolism. Other sources of renal insult in selected patients may include rhabdomyolysis and specific drug-related effects. Certain classes of medications may also contribute to hypoperfusion by virtue of their hemodynamic effects (notably angiotensin-converting enzyme [ACE] inhibitor 1, angiotensin-receptor blockers [ARBs], and nonsteroidal antiinflammatory drugs [NSAIDs]), and, consequently, the risk of ATN.
Ischemic renal failure related to shock or severe dehydration is always preceded by an early compensatory phase of normal renal adaptation (e.g., pre-prerenal failure), followed by a condition termed prerenal azotemia during which the kidney maximizes activities at the expense of the retention of nitrogenous end-products to preserve the internal environment through retention of solutes and water ( Fig. 42.2 ). In studies of community-acquired AKI, the incidence of prerenal azotemia may be as frequent as 70%. In contrast, in a classic study of hospital-acquired AKI, although hypoperfusion accounted for 42% of cases of AKI, only 41% of these cases of hypoperfusion were attributable to inadequate intravascular volume.

Although prerenal azotemia is ominous and typically accompanied by oliguria (<0.5 mL/kg/h), it is reversible. At a critical tilting point, as conditions go beyond the compensatory mechanisms that maintain renal perfusion, ischemia leads to irreversible renal cell necrosis or ATN. This represents the pure form of ischemic AKI. Other forms of ATN are due to toxins, including medications (e.g., aminoglycosides, cisplatinum), pigments (e.g., hemoglobin, myoglobin), and iodinated contrast dye. These forms of ATN do not involve the typical pattern of preceding prerenal azotemia with associated oliguria, since the insult is sudden. Importantly, most cases of perioperative AKI are the result of numerous renal insults, rather than being attributable to one pure source ( Fig. 42.3 ). In particular, patients with prerenal azotemia are likely at increased risk for toxic ATN.

Interruption of blood flow to the kidneys for more than 30 to 60 minutes results in ATN and irreversible cell damage. The kidneys receive 1000 to 1250 mL/min of blood or 3 to 5 mL/min/g of tissue for the average adult, and this amount far exceeds what is needed to provide the kidney’s intrinsic oxygen requirement. Intracortical blood flow may not be evenly distributed. Because the renal cortex contains most of the glomeruli and depends on oxidative metabolism for energy, ischemic hypoxia injures the renal cortical structures, particularly the pars recta of the proximal tubules. As ischemia persists, the supply of glucose and substrates continues to decrease; glycogen is consumed, and the medulla, which depends to a great extent on glycolysis for its energy sources, becomes more adversely affected. Early cell changes are reversible, such as the swelling of cell organelles, especially the mitochondria. As ischemia progresses, a lack of adenosine triphosphate interferes with the sodium pump mechanism, water and sodium accumulate in the endoplasmic reticulum of tubular cells, and the cells begin to swell. Onset of tubular damage usually occurs within 25 minutes of ischemia as the microvilli of the proximal tubular cell brush borders begin to change. Within an hour, they slough off into the tubular lumen, and membrane bullae protrude into the straight portion of the proximal tubule. After a few hours, intratubular pressure rises, and tubular fluid passively backflows. Within 24 hours, obstructing casts appear in the distal tubular lumen.
Renal Response to Hypoperfusion: Autoregulation and Distribution of Cardiac Output to the Kidneys
A common intraoperative stress that puts patients at risk for AKI is hypoperfusion due to hypotension and/or hypovolemia. The fraction of cardiac output perfusing the kidneys depends on the ratio of renal vascular resistance to systemic vascular resistance. In general, the response to renal hypoperfusion involves three major regulatory mechanisms that support renal function: (1) afferent arteriolar dilation increases the proportion of cardiac output that perfuses the kidney; (2) efferent arteriolar resistance increases the filtration fraction and preserves GFR; and (3) hormonal and neural responses improve renal perfusion by increasing intravascular volume, thereby indirectly increasing cardiac output.
The kidney produces vasodilator prostaglandins to counteract the effects of systemic vasoconstrictor hormones such as angiotensin II. In a state of low cardiac output when systemic blood pressure is preserved by the action of systemic vasopressors, RBF is not depressed because the effect of the vasopressors is blunted within the kidney. Studies using specific inhibitors of angiotensin II have shown that efferent arteriolar resistance largely results from the action of angiotensin II. At low concentrations, norepinephrine has a vasoconstricting effect on efferent arterioles, indicating that the adrenergic system may also be important for maintaining the renal compensatory response.
Reductions in cardiac output are accompanied by the release of vasopressin and by increased activity of the sympathetic nervous system and the renin-angiotensin-aldosterone system. These regulatory mechanisms to preserve RBF conserve salt and water. One study reported the normal response to hemorrhage in otherwise healthy patients, describing a 30% reduction in RBF with a decrease in mean perfusion pressure from 80 to 60 mm Hg. Changes known to occur at the initiation of cardiopulmonary bypass (CPB) surgery include greater reduction in renal perfusion than systemic perfusion, loss of RBF autoregulation, and stress hormone and inflammatory responses known to be harmful to the kidney. These effects may explain why the duration of CPB surgery independently predicts postcardiac surgery renal impairment.
Detection of Acute Kidney Injury
Laboratory Tests of Serum and Urine as Markers of Renal Function
Standard serum and urine markers of renal function are discussed in Chapter 17 . It should be emphasized that at present, repeated serum creatinine determinations (relative or absolute changes), are most often used to identify AKI. An inherent limitation of almost all currently available tools to detect AKI is the obligate delay between the onset of AKI and the diagnosis of AKI. One striking difference between the management of AKI and acute myocardial infarction is the lack of early biomarkers for AKI in routine clinical practice to guide prompt recognition and intervention when tissue is threatened. Thus significant ongoing efforts are testing the value of early AKI biomarkers and of real-time measurement of GFR.
Novel (Early) Biomarkers of Acute Kidney Injury
Limited progress in the care for AKI has fueled an enormous interest in new early biomarkers. Although a few of the new tools represent attempts to find a filtration marker that is better than creatinine (e.g., cystatin C), most novel AKI biomarkers take advantage of one of the three early consequences of AKI: (1) tubular cell damage, (2) tubular cell dysfunction, and (3) adaptive stress response of the kidney. The hope is that such biomarkers will allow timely AKI identification, diagnosis (e.g., prerenal azotemia vs. ATN), and prognosis. Some of the more promising biomarkers are presented here ( Box 42.1 ).
Filtration-Based Markers of Renal Dysfunction
Cystatin C
β-trace protein
β-2 microglobulin
Biomarkers Reflecting Renal Tubular Cell Damage (Tubular Enzymuria)
α-Glutathione S-transferase
π-Glutathione S-transferase
β- N -Acetyl-β- d -glucosaminidase
γ-Glutamyl transpeptidase
Alkaline phosphatase
Sodium hydrogen exchanger isoform 3
Biomarkers Reflecting Renal Tubular Cell Dysfunction (Tubular Proteinuria)
α 1 -Microglobulin
β 2 -Microglobulin
Albumin
Retinol-binding protein
Immunoglobulin G
Transferrin
Ceruloplasmin
Lambda and kappa light chains
Biomarkers Reflecting Renal Tubular Cell Response to Stress
Neutrophil gelatinase–associated lipocalin
Urinary interleukin-18
Kidney injury molecule-1
Liver fatty acid–binding protein
Insulin-like growth factor binding protein 7
Tissue inhibitor of metallo-proteinase 2
New Filtration-Based Markers of Renal Dysfunction
Of the most novel filtration-based markers of renal dysfunction, the most advanced is cystatin C, a member of the cystatin superfamily of cysteine-proteinase inhibitors that is produced by all nucleated cells at a constant rate. Cystatin C has been clinically available for longer than 15 years and can be rapidly determined. Similar to creatinine, cystatin C accumulates in the circulation with renal impairment and can be used as a marker of glomerular filtration. Serum cystatin C has theoretical advantages over creatinine, particularly as an indicator of mild chronic kidney disease and its sequelae. Several GFR estimating equations have been proposed, based on cystatin C alone or cystatin C and creatinine for use in chronic kidney disease. Although these are commonly used in clinical research studies, in general these are not in widespread clinical use at present.
Although cystatin C outperformed creatinine in detecting AKI after cardiac surgery in some small studies, this sensitivity has not consistently been the case. Indeed, a large multicenter prospective observational study of AKI after cardiac surgery suggested that serum cystatin C was less, not more, sensitive for the detection of AKI. This study, conducted by the Translational Research Investigating Biomarker Endpoints in Acute Kidney Injury (TRIBE-AKI) Consortium, prospectively enrolled more than 1200 adults undergoing cardiac surgery and has rapidly advanced the field of novel biomarkers in this context. Of note, the subset of patients who had AKI by cystatin C and creatinine had more frequent risk of dialysis and death than those who had AKI by creatinine alone. Conditions such as malignancy, human immunodeficiency viral infection, or corticosteroidal or thyroid hormone therapy are associated with increased serum levels of cystatin C without changes in renal function. Other novel markers of filtration include β-trace protein and β-2-microglobulin; these markers may be novel markers of mortality in the general population, compared with creatinine-based eGFR. However, the additional utility of these markers in estimating GFR above and beyond creatinine and cystatin C is unknown.
Biomarkers Reflecting Renal Tubular Cell Damage (Tubular Enzymuria)
Renal tubular cells contain enzymes that are highly specific to their location within the kidney and even tubule region. Under conditions of cellular stress, these enzymes are shed into the urine, making these potentially appealing markers of kidney dysfunction. These markers include α and π isomers of glutathione s-transferase (GST), which are cytosolic enzymes from proximal and distal tubular cells, respectively, and N-acetyl-β-D-glucosaminidase, a proximal tubule lysosomal enzyme. Of note, although enhanced urinary excretion of tubular enzymes can signal damaged tubular cells, it can also reflect an increased turnover of tubular cells or some other metabolic disturbance; thus the use of these markers may need to be applied with caution.
Biomarkers Reflecting Renal Tubular Cell Dysfunction (Tubular Proteinuria)
When small proteins are filtered by the glomerulus, binding and endocytic reuptake in the proximal tubule normally returns these substances to the body through a megalin-mediated transport system. So-called tubular proteinuria results from functional impairment of this process and the escape of small proteins into the urine. Endogenous low molecular weight proteins that are normally taken up in this way include β 2 – and α 1 -microglobulin, retinol-binding protein, lysozyme, ribonuclease, IgG, transferrin, ceruloplasmin, and lambda (λ) and kappa (κ) light chains. Appearance of any of these substances in the urine heralds abnormal proximal nephron function consistent with AKI. However, lysine and its analog (e.g., ε-aminocaproic acid, tranexamic acid) can cause a profound but reversible inhibition of low molecular weight protein reuptake that is transient and apparently benign due to blocking renal-binding sites.
Biomarkers Reflecting Renal Tubular Cell Response to Stress
Examples of markers that assess the response of the kidney to stress include neutrophil gelatinase–associated lipocalin (NGAL), kidney injury molecule–1 (KIM-1), liver fatty acid–binding protein, and interleukin-18 (IL-18). These have each been extensively reviewed in detail in recent literature. Two markers of G1-cell cycle arrest have been shown to be upregulated in AKI, insulin-like growth factor–binding protein 7 (IGFBP-7) and tissue inhibitor of metalloproteinases–2 (TIMP-2). A test that combines levels of IGFBP-7 and TIMP-2 has received U.S. Food and Drug Administration (FDA) clearance as a biomarker for the identification of patients at high risk of AKI.
NGAL is a protein with a critical role in iron scavenging. A transcriptome-wide interrogation for genes induced very early after renal ischemia identified NGAL as a protein generated by ischemic renal tubular cells. Administration of exogenous NGAL in animal models attenuates renal injury. Enthusiasm for NGAL as a biomarker of AKI was fueled by a study in pediatric patients who underwent cardiac surgery, suggesting that plasma and urinary NGAL could predict AKI before elevations in serum creatinine ( Fig. 42.4 ). Although increased NGAL levels are associated with adverse outcomes, studies in the adult perioperative literature have not consistently shown NGAL to predict AKI before elevations in serum creatinine.

KIM-1 is a transmembrane protein expressed at low levels under normal conditions that is significantly upregulated in proximal renal tubular cells in response to ischemic or nephrotoxic AKI. In studies conducted by the Predictive Testing Safety Consortium, an academic-industrial partnership that has worked closely with the FDA to develop novel biomarkers of kidney injury for use in the preclinical setting (in particular, as markers of drug toxicity), KIM-1 outperformed a number of traditional markers of kidney injury in a variety of preclinical models. More recently, assays have been developed to detect plasma KIM-1, which appears to correlate with urinary KIM-1 in the setting of both acute and chronic kidney disease.
Finally, cell cycle arrest has been implicated in models of AKI; more recently, two proteins that induce cell cycle arrest, IGFBP-7 and TIMP-2, have both been shown in a multicenter study to predict the development of KDIGO stage 2 and 3 AKI in critically ill patients. In a validation cohort, these biomarkers had an impressive area under the ROC curve of 0.80 for subsequent AKI. There has been tremendous interest in the use of these biomarkers for perioperative risk stratification, with clinical trials enrolling patients based on elevated levels of IGFBP-7 and TIMP-2 (typically expressed as the product of these two biomarkers).
Preoperative Evaluation of Renal Function and Risk Stratification
The greater the magnitude and duration of the surgical insult and the number of acute and chronic risk factors, the greater the likelihood of perioperative renal compromise and hence the need for preoperative identification of high-risk individuals. Common risk factors for AKI include intravascular volume depletion, aminoglycoside use, radiocontrast dye exposure, use of NSAIDs, septic shock, and pigmenturia. ACE inhibitors and ARBs, respectively, may contribute to intraoperative hypotension and may exacerbate AKI in the setting of impaired hemodynamics (volume depletion, NSAID use). Patients with preexisting renal insufficiency are clearly at increased risk for AKI and should be identified in the perioperative evaluation through measurement of serum creatinine and urinalysis (to evaluate for albuminuria/proteinuria). Chronic kidney disease affects more than 10% of the United States population. Common risk factors for chronic kidney disease include advanced age, diabetes, and hypertension. The potential value of understanding the genetic makeup of patients has yet to be fully explored and is likely to be important. For example, several genetic polymorphisms known to affect inflammation and vasoconstriction demonstrate strong associations with AKI after cardiac surgery, including the IL-6 572C and angiotensinogen 842C polymorphisms. In the future, identification of such polymorphisms may improve perioperative risk stratification.
Large multicenter epidemiologic studies have identified a relationship between markers of abnormal central aortic compliance, such as preoperative isolated systolic hypertension (>160 mm Hg) and wide pulse pressure hypertension (>40 mm Hg), and postoperative AKI and dialysis, in particular in patients undergoing cardiac surgery. Pulse pressure is an index of the effects of large artery stiffness and the rate of pressure on propagation and reflection within the arterial tree. Early return of reflected arterial waves during late systolic rather than early diastolic pressure (from increased propagation velocity in stiff vessels) increases systolic blood pressure (i.e., afterload) and decreases diastolic blood pressure (i.e., perfusion pressure). Perfusion pressure and the risk of perioperative renal dysfunction are linked by the preexisting capacity of the vasculature to compensate for low pressure as it determines flow. Those with a predisposition to low flow attributable to abnormal central aortic compliance (e.g., those with wide pulse pressure) may represent patients who require higher pressure to maintain adequate flow and minimize renal risk compared with normotensive patients.
In the context of specific surgery types (e.g., cardiac surgery), perioperative risk prediction tools have been developed. In general, these tend to effectively identify low-risk populations, but discrimination for higher risk patients is more modest. This may reflect the fact that intraoperative factors play a major role in the pathogenesis of AKI in a vulnerable patient. In addition, a number of these risk prediction tools focus on dialysis-requiring AKI, which is only the “tip of the iceberg” with regard to disease burden.
Perioperative Acute Kidney Injury: Mechanisms and Treatment
As described in Chapter 17 , anesthesia and surgery influence normal renal function primarily through changes in GFR and urine flow that are attributable to changes in blood pressure and cardiac output. Fluctuations in blood pressure have a major effect on RBF and glomerular filtration. Here, we will briefly discuss considerations for several anesthetic techniques, followed by several specific perioperative considerations.
Regional Anesthesia
Regional anesthetics and the kidneys interact in a complex manner that varies according to the underlying cardiovascular, renal, fluid, and electrolyte status of the patient. In general, epidural and spinal anesthesia reduce systemic and renal vascular sympathetic tone. Spinal cord segments T4 through L1 contribute to the sympathetic innervation of the renal vasculature, which is mediated by sympathetic fibers from the celiac and renal plexus. Autonomic blockade above the fourth thoracic level also blocks cardioaccelerator sympathetic innervation to the heart. If neuraxial blockade reduces arterial blood pressure and cardiac output, then the RBF will be decreased with matching reductions in glomerular filtration and urine output.
Although controversial, intraoperative neuraxial blockade and postoperative epidural analgesia decrease rates of AKI. Rodgers and colleagues conducted a systematic review of 107 randomized clinical trials of intraoperative neuraxial blockade and demonstrated a 30% reduction in the odds of postoperative mortality. This reduction was associated with decreases in the incidence of deep venous thrombosis, pulmonary embolism, transfusion, pneumonia, and respiratory depression, as well as renal failure, although the confidence limits for the renal failure estimates were very wide, in part due to the small number of cases of renal failure observed. Moraca and associates conducted a meta-analysis and reported on the association of thoracic epidural anesthesia with improved surgical outcomes attributable, in part, to a reduction of perioperative morbidity, including reductions in infection, ileus, blood loss, and AKI. Other studies have examined the impact of epidural anesthesia during cardiac surgery and have suggested a benefit with regards to renal failure, although the confidence intervals were wide. Unfortunately, renal failure was not an outcome of several recently published meta-analyses focused on epidural anesthesia during cardiac surgery. Finally, with regard to postoperative analgesia, a recent Cochrane meta-analysis that focused on abdominal aortic surgery suggested better postoperative analgesia and a reduction in other complications including myocardial infarction and respiratory with epidural analgesia but no effect on AKI or postoperative mortality.
Effects of Inhaled Anesthetics
From a historic perspective, older volatile inhaled anesthetics including methoxyflurane and enflurane (no longer clinically used) when used for prolonged periods lead to significant generation of inorganic fluoride and were associated with polyuric renal insufficiency. However, despite significant fluoride generation with sevoflurane and the generation of compound A (a metabolite associated with renal injury in experimental models), there is no reported association with AKI. This may be due to the shorter duration of elevated fluoride levels with sevoflurane and the site of metabolism (intrarenal metabolism of methoxyflurane is fourfold greater than that of sevoflurane).
Effects of Intravenous Anesthetics
Propofol and dexmedetomidine may have antiinflammatory effects that are renoprotective. Propofol increases production of bone morphogenetic protein–7 (BMP-7), which suppresses the tumor necrosis factor α–induced inflammatory cascade during sepsis-induced AKI, as well as decreased injury during ischemia-reperfusion and unilateral ureteral obstruction. Similarly, in addition to altering RBF and sodium and water handling, the α 2 -adrenoreceptor agonists such as dexmedetomidine may stimulate BMP-7 production in the setting of sepsis and ischemia-reperfusion. There has been significant interest in the use of dexmetomidine, in particular in the setting of cardiac surgery, with a recent meta-analysis suggesting a reduction in postoperative AKI with the use of dexmetomidine (OR 0.65; 95% confidence interval [CI], 0.45-0.92, P = .02).
Specific Perioperative Perturbations and Renal Function
Several surgical interventions can affect RBF and, consequently, renal function. Whereas aortic cross-clamping above the renal arteries has obvious influence on glomerular filtration, infrarenal aortic cross-clamping and unclamping also have significant indirect effects on glomerular filtration and urine formation through changes in myocardial function, sympathetic activity, neuronal and hormonal activity (e.g., renin and angiotensin production), intravascular volume, and systemic vascular resistance. During standard CPB surgery, cardiorenal relationships are approximately as expected; RBF decreases to 12% to 13% of total pump flow and is predicted by flow rate and perfusion pressure; however, only mean pressure correlates with urine output.
AKI after aortocoronary bypass surgery continues to be a devastating complication that is associated with multiorgan dysfunction, increased resource utilization, high cost, and increased mortality. Annually, approximately 350,000 patients in the United States undergo coronary artery bypass graft (CABG) surgery. In a multicenter observational study focused on AKI after cardiac surgery, 5% of participants developed AKI as defined by the need for acute dialysis or a doubling of serum creatinine from baseline. The mechanism of perioperative AKI during cardiac surgery is multifactorial. Significant risk factors for AKI include underlying patient characteristics, such as age older than 75 years, history of diabetes, hypertension, pulse pressure, ventricular dysfunction, myocardial infarction, renal disease, perioperative medication exposures (e.g., aprotinin, hetastarch), and surgical characteristics such as intraoperative use of multiple inotropes, insertion of intraaortic balloon pump, and extended duration of the CPB surgery.
The role of CPB surgery in postoperative AKI remains controversial. In their comprehensive guidelines on AKI, KDIGO reviewed the literature on postoperative AKI between patients undergoing off-pump and on-pump coronary revascularization surgeries and ultimately recommended that “off-pump CABG surgery not be selected solely for the purpose of reducing perioperative AKI or need for renal replacement therapy (RRT).” However, patients with chronic kidney disease, who are at the highest risk for AKI after CPB surgery, have often been excluded from randomized clinical trials of off-pump versus on-pump CABG. For example, in the Randomized On/Off Bypass (ROOBY) trial, in which 2303 patients were randomized to off-pump versus on-pump CABG, approximately 7.5% of patients had a preoperative serum creatinine level ≥1.5 mg/dL.
A large observational study of 742,909 nonemergent, isolated CABG cases (including 158,561 off-pump cases) from the Society of Thoracic Surgery Database suggests a benefit to off-pump CABG in those with chronic kidney disease. Propensity methods were used to adjust for patient- and center-level imbalances. The primary endpoint was death or dialysis. In those with lower estimated GFR (eGFR), the risk difference (i.e., number of patients with the outcome per 100 patients in those who underwent CPB minus the number with the outcome in those who underwent off-pump CABG) for the primary endpoint was 0.66 (95% CI, 0.45-0.87) for eGFR 30 to 59 mL/min/1.73 m 2 and 3.66 (95% CI, 2.14-5.18) for eGFR 15 to 29 mL/min/1.73 m 2 . Both component endpoints followed the same trend. Highlighting the importance of chronic kidney disease as a risk factor for AKI after cardiac surgery, whereas slightly less than 1% of the overall cohort received dialysis after cardiac surgery, 2% of those with eGFR 30 to 59 mL/min/1.73 m 2 and 12.5% of those with eGFR 15 to 29 mL/min/1.73 m 2 required dialysis. The risk difference for dialysis alone in the same groups was 0.47 (95% CI, 0.31-0.62) and 2.79 (95% CI, 1.37-4.20) with on-pump versus off-pump CABG, respectively ( Fig. 42.5 ). Along the same lines, a 2932 patient-substudy of the CORONARY clinical trial followed patients after off- or on-pump CABG surgery and demonstrated an interaction between preoperative chronic kidney disease (defined as eGFR <60 mL/min/1.73 m 2 ) and AKI (defined as a 50% increase in serum creatinine from prerandomization baseline within 30 days). In those with chronic kidney disease, 19.2% of those in the off-pump arm had AKI, compared to 30.2% in those who had on-pump surgery. However, there was no difference in sustained renal dysfunction, defined as a 20% or greater loss in eGFR at 1 year between the two study arms (17.1% vs. 15.3% in the off- vs. on-pump arms, P = .23). However, when those with AKI were compared to those who did not have AKI (regardless of treatment arm), AKI was independently associated with an increased risk of sustained renal dysfunction, with an adjusted odds ratio of 3.37 (95% CI, 2.65-4.28, P < .001. This study highlights the fact that in the setting of chronic kidney disease, AKI is only one of many risk factors for chronic kidney disease progression.

In the setting of CABG, there has been tremendous interest in prevention of AKI, and numerous pharmacologic interventions have been tested. As stated previously, one of the major challenges for AKI prevention is that both pre- and intraoperative factors impact the risk of AKI, so it is difficult to identify a priori those who are at highest risk of AKI. Interventions of recent interest where there is not a sufficient evidence base for wide-spread adoption include remote ischemic preconditioning, atrial natriuretic peptide, and fenoldopam, among others.
Intraoperative Management for AKI Prevention: Oxygen Delivery: Blood Gas, Acid-Base Balance, and Hematocrit
Severe arterial hypoxemia to a partial arterial pressure of oxygen (PaO 2 ) value of less than 40 mm Hg is associated with decreased RBF and renal vasoconstriction. Inequalities in oxygen supply and demand are exaggerated and medullary hypoxia is extreme during CPB, effects that last well beyond separation from circulatory support in experimental models.
The effects of anemia on the kidney have been studied mostly in the context of CPB management. When crystalloid and colloid solutions are used to prime an extracorporeal circuit, the initiation of CPB surgery obligates an acute decrease of approximately 30% in oxygen-carrying capacity. Animal studies endorse moderate hemodilution (hematocrit 20% to 30%) as renoprotective during CPB surgery through a reduction of blood viscosity and improved regional blood flow. However, although hematocrit values less than 20% during CPB surgery are commonly accepted clinically (extreme hemodilution), very low hematocrit values are linked with adverse outcomes, including AKI. The Society of Thoracic Surgeons and the Society of Cardiovascular Anesthesiologists guidelines suggest that transfusion triggers should be lower during CPB surgery and that a transfusion trigger of 6 g/dL is reasonable, except in those at risk for end-organ ischemia, in whom a higher trigger may be reasonable. More recently, the European Association for Cardio-Thoracic Surgery and the European Association of Cardiothoracic Anesthesia have developed comprehensive guidelines and suggest that a target hematocrit of 21% to 24% during cardiopulmonary bypass is reasonable.
The solution may not be as simple as transfusion because blood transfusions themselves have been linked to AKI. In a systematic review, Karkouti found 22 studies that examined the association between blood transfusions and AKI postcardiac surgery. An independent association between transfusions and AKI was found in 18 of the 22 studies. The association of perioperative anemia with AKI was further examined in 14 studies, and 9 of the studies found an independent association of perioperative anemia with AKI. Proposed mechanisms for transfusion-associated AKI include exacerbation of inflammation and oxidative stress, which occur during the CPB surgery.
There has been tremendous interest in the impact of the age of stored blood on the risk of AKI. Old blood may result in higher circulating levels of free hemoglobin and free iron, and there has been interest in the impact of the “storage lesion” on AKI. However, at present, data does not support the selective use of fresh blood in the perioperative setting to reduce the risk of AKI.
Perioperative Blood Pressure and Fluid Management
There has been significant interest in perioperative blood pressure targets and the impact on AKI. Several large observational cohort studies have suggested that even transient hypotension was associated with postoperative AKI. One prospective randomized trial compared an individualized blood pressure management strategy targeted at maintaining systolic blood pressure within 10% of baseline versus a standard management strategy (treatment of systolic blood pressure <80 mm Hg or lower than 40% from baseline) and demonstrated a significant reduction in the primary composite endpoint (systemic inflammatory response and at least one organ dysfunction within 7 days). This modest sized clinical trial demonstrated a nonsignificant trend toward reduced postoperative AKI in the individualized blood pressure management arm. Thus blood pressure targets, in particular in patients with chronic kidney disease, need to take into consideration preoperative blood pressure.
Intravascular volume depletion, which commonly occurs in fasting patients undergoing surgery, is a risk factor for AKI. For example, the combination of diabetes mellitus and intravascular volume depletion increases the chance of developing AKI by 100-fold. The most practical preoperative methods to assess volume status are with preoperative patient history and physical examination and by assessing changes in arterial blood pressure in response to changing conditions and dynamic maneuvers. For example, an awake patient normally does not have significant orthostatic changes in arterial blood pressure unless an autonomic or intravascular volume deficit exists. During anesthesia, a similarly dehydrated patient may demonstrate paradoxical arterial pulse changes with positive-pressure inspiration. Nonetheless, there has been tremendous interest in the use of intravascular monitoring techniques in the perioperative setting to reduce the risk of AKI.
The decision to use any monitor should depend on the patient’s functional cardiac reserve status and the extent of the proposed surgical insult. Although maintaining adequate cardiac output is necessary for maintaining adequate RBF, adequate flow may still not occur. The use of intravascular volume monitoring techniques must include cautious identification of physiologic conditions that influence their validity as a reflection of preload in a particular patient. For example, monitoring central venous pressure to assess preload involves assumptions about normal left and right ventricular function; pulmonary vascular resistance; and mitral, pulmonary, and tricuspid valve function. Similarly, monitoring pulmonary artery pressure or pulmonary capillary wedge pressure assumes normal left ventricular compliance, mitral valve function, and normal airway pressure.
Direct measurements of left atrial pressure may offer insight into the kidney pressure-flow relationship because left atrial hypotension is a powerful stimulus for renal vasoconstriction. Despite equivalent reductions in cardiac output and arterial blood pressure, RBF decreases significantly more when left atrial pressure is decreased (e.g., hemorrhagic shock), compared with left atrial pressure when it is increased (e.g., cardiogenic shock). Left atrial pressure receptors modulate renal vasoconstriction through the release of ANP, a hormone secreted by the cardiac atria in response to intravascular volume expansion. ANP acts on the arterial and venous systems, the adrenal glands, and the kidneys to reduce intravascular volume and decrease blood pressure. Within the kidney, the hormone increases hydraulic pressure in the glomerular capillaries through afferent arteriolar dilation and efferent arteriolar vasoconstriction. ANP reduces arterial blood pressure by relaxing smooth muscle and reducing sympathetic vascular stimulation and also inhibits renin and aldosterone secretion, causing renal vasodilation, natriuresis, and diuresis.
Despite the direct relationship of left atrial pressure and renal vasoconstriction, static monitors of intravascular volume status are gradually being replaced by echocardiographic and dynamic monitors of intravascular volume status. Intraoperatively, one of the most direct ways to monitor intravascular volume may be by direct assessment of the left ventricular end-diastolic area with transesophageal echocardiography. However, monitoring with invasive devices, such as pulmonary artery catheters, arterial cannulas, and transesophageal echocardiography, has not been demonstrated to reduce the incidence of AKI.
Guided fluid optimization has recently garnered significant interest as a step beyond traditional, somewhat unreliable guides to fluid administration (e.g., central venous pressure). The principle behind fluid optimization is to maximize tissue-oxygen delivery by achieving a maximum stroke volume. Intravascular fluid management is typically guided by the physiologic response to dynamic measures; proposed measures include systolic pressure variation, pulse pressure variation, continuous cardiac output monitoring, and esophageal Doppler ultrasonography fluid boluses. Some maneuvers to assess fluid responsiveness may be feasible in the critical care setting but not in the perioperative setting (e.g., passive leg raise).
Given the improved outcomes in patients who are critically ill with the acute respiratory distress syndrome and are managed with a restrictive fluid management strategy, fluid restriction in the perioperative setting has gained attention. A meta-analysis of seven randomized clinical trials in the setting of intraabdominal surgery suggested a restrictive fluid strategy offered no benefit; however, there was also no evidence of harm, including AKI. In some studies, excessive fluid restriction was associated with harm, including an increased risk of anastomotic breakdown and sepsis, and should clearly be avoided. However, more recently, the RELIEF clinical trial randomized 3000 patients undergoing major abdominal surgery to a restrictive or liberal fluid strategy during surgery and up to 24 hours afterward; the restrictive fluid strategy was designed to provide a net even fluid balance. There was no difference in the primary endpoint of disability-free survival at 1 year. However, the restrictive fluid strategy was associated with an increased rate of AKI (8.6% vs. 5.0%, P < .001) and surgical site infections (16.5% vs. 13.6%, unadjusted P = .02), though the comparison for surgical site infections was not considered significant after adjustment for multiple comparisons. Thus at present, fluid management strategies should avoid markedly positive fluid balance, but also need to be wary of underresuscitation.
There has been considerable interest in the use of balanced salt solutions instead of chloride-rich solutions to prevent AKI; the rationale is that chloride-rich solutions reduce renal perfusion in animal models. Subsequently, a pre-post study of chloride-rich versus balanced salt solutions demonstrated a reduced incidence of stage 2 and 3 AKI during the balanced salt solution period. Similarly, a propensity-matched study of patients undergoing major abdominal surgery suggested that patients who received balanced salt solutions had fewer postoperative complications, including postoperative dialysis. However, a subsequent clinical trial, the SPLIT trial, suggested that there was no decrease in AKI with the use of Plasma-Lyte 148 in ICU patients. Of note, 72% of the trial population was enrolled after surgery, with 49% enrolled after cardiac surgery. More recently, the SALT-ED and SMART trials were pragmatic clinical trials that randomized patients to receive balanced salt solutions or normal saline in alternating months in the Emergency Department and Intensive Care Units, respectively, at a single clinical center. Both studies demonstrated a statistically significant reduction in the rate of major adverse kidney events at 30 days, a composite endpoint of death, dialysis, and persistent doubling of creatinine. In SMART, approximately 20% of subjects were admitted in the postoperative setting; there was no evidence of an interaction between treatment effect and type of ICU, but the study was underpowered to observe a difference within this subgroup.
Finally, are these solutions safe in patients who are prone to hyperkalemia? Because balanced salt solutions contain a physiologic amount of potassium, there has been concern that the administration of balanced salt solutions might lead to hyperkalemia in patients with reduced renal function. However, in two randomized clinical trials of intraoperative fluid selection in end-stage kidney disease patients undergoing kidney transplantation, there was no increase in the incidence of hyperkalemia. The median volume of intravenous solution administered in a the trial that compared normal saline to an acetate based buffer was 2625 mL (IQR 2000-3001 mL); in a trial comparing normal saline to lactated Ringers, mean fluid volumes were 6.1 ± 1.2 L and 5.6 ± 1.4 L, respectively. Thus at reasonable doses, balanced salt solutions appear safe across a wide variety of patient populations and are associated with a decreased risk of AKI.
Excessive Intravascular Volume and the Abdominal Compartment Syndrome
The abdominal compartment syndrome was first defined in 1985; since then, it has been progressively recognized as a common contributor to renal dysfunction in the setting of excessive intravascular volume. Not surprisingly, excessive intravascular volume and mechanical ventilation with high airway pressures are significant contributors to the abdominal compartment syndrome. Abdominal compartment syndrome is defined as a sustained increase in intraabdominal pressures to greater than 20 mm Hg that causes organ dysfunction; in contrast, intraabdominal hypertension is typically defined as intraabdominal pressures ≥12 mm Hg without organ dysfunction. This increase in intraabdominal pressure decreases abdominal perfusion pressure (which is mean arterial pressure − intraabdominal pressure), and results in a functional prerenal state attributable to reduced renal perfusion.
The intraabdominal pressure can easily be measured using an indwelling Foley catheter and the same pressure tubing setup used for arterial line blood pressure monitoring. The Foley catheter is clamped distal to the instillation port, and up to 25 mL of saline is instilled into the bladder. The transducer should be zeroed at the midaxillary line, and the pressure should be measured at end-expiration approximately 30 to 60 seconds after fluid is instilled into the bladder to allow for detrusor muscle relaxation. Of note, intraabdominal pressure may be chronically elevated to as high as 12 mm Hg in the obese adult but is not associated with end-organ dysfunction in this context. Prompt recognition of the abdominal compartment syndrome and treatment via decompression in the appropriate clinical context is critical.
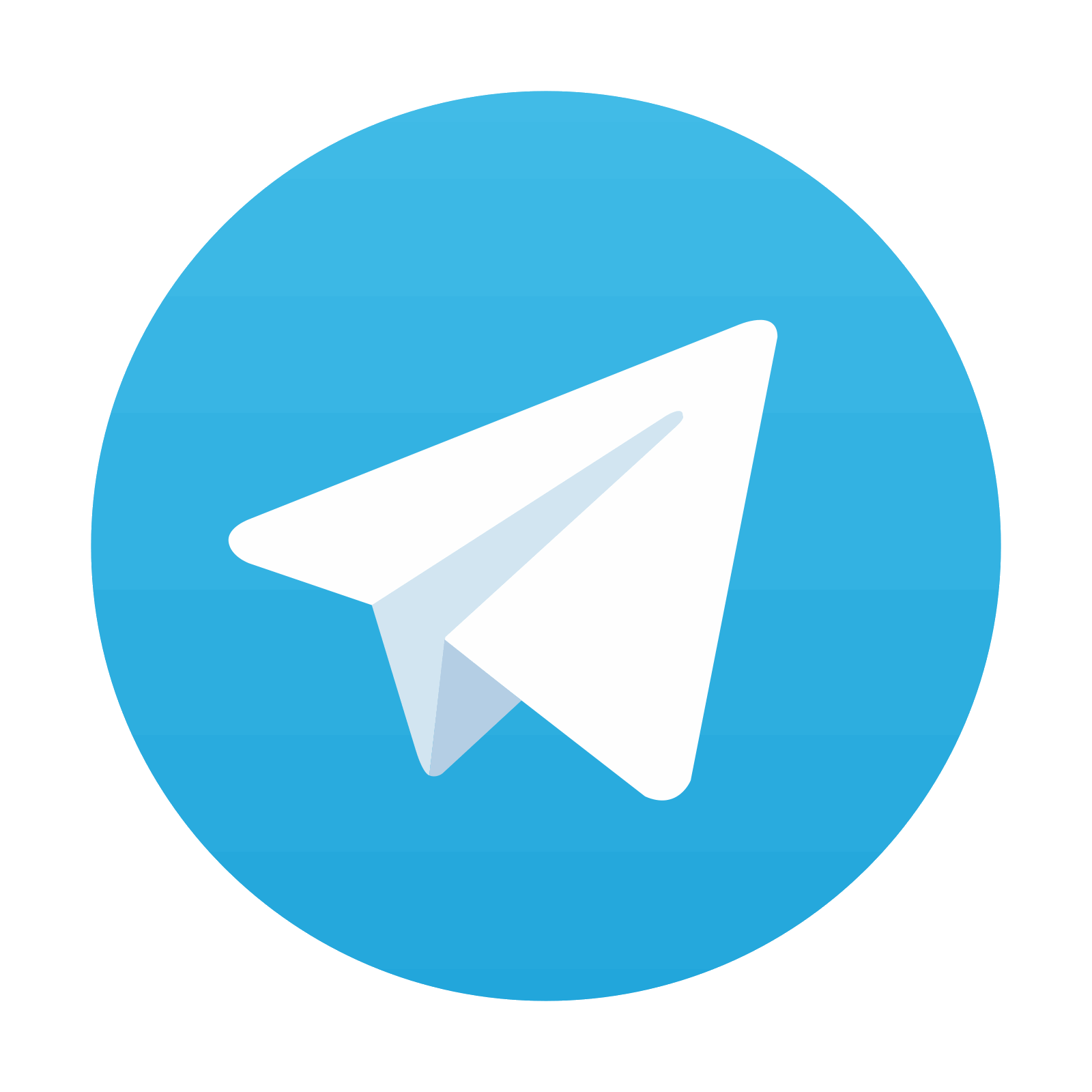
Stay updated, free articles. Join our Telegram channel
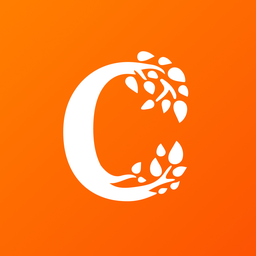
Full access? Get Clinical Tree
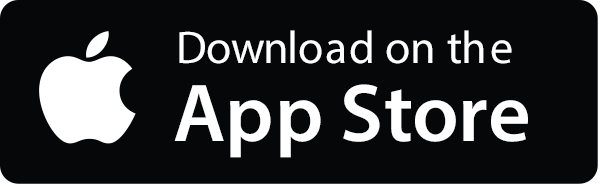
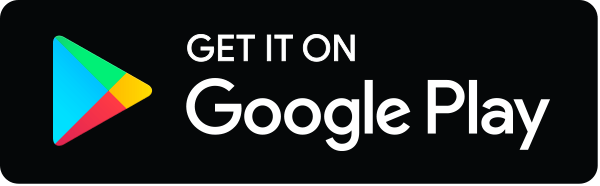