Abstract
This chapter describes the control systems and mechanisms of air movement into and out of the lungs to allow oxygen and carbon dioxide to exchange with blood. Emphasis is placed on those systems where the molecular physiology constitutes targets for the actions of drugs on the respiratory system. Coordinated contraction of airway, intercostal, and diaphragm muscles is required to cause inspiration of gas into the lungs. Expiration is normally passive but when ventilation increases (e.g., in response to exercise), expiration becomes active with contraction of the internal intercostal and abdominal muscles. Most bronchial smooth muscle is found in the 12th to 16th generations of the airway, and four mechanisms control muscle tone in these airways: neural, humoral, direct physical and chemical, and local cellular pathways. Neural control is the most important in normal lung, but airway smooth muscle contains numerous adrenoreceptors that respond to circulating catecholamines. In healthy lungs ventilation and perfusion are well matched and approximately equal, but in diseased lungs or during general anesthesia there are discrepancies between the two that can adversely affect gas exchange, impairing both oxygenation and carbon dioxide elimination. Regional hypoxic pulmonary ventilation is a reflex that acts to divert pulmonary blood flow away from hypoxic areas of lung and is an important mechanism for optimizing ventilation-perfusion relationships. The respiratory pattern is generated within the medulla, which coordinates the voluntary and involuntary demands of respiratory activity via multiple neuronal connections. Reflex control of breathing occurs in response to both carbon dioxide and oxygen.
Keywords:
respiration, inhalation, exhalation, airway resistance, bronchioles, asthma, airway remodeling
Chapter Outline
Active Control of Pulmonary Vascular Resistance
This chapter describes the physiology of the respiratory system by detailing the control systems and mechanisms of air movement into and out of the lungs that allow oxygen and carbon dioxide to exchange with blood. Emphasis is placed on those systems where the molecular physiology is understood as these systems constitute targets for the actions of drugs on the respiratory system, both beneficial and harmful.
Pulmonary Ventilation
Ventilation is the process by which air is drawn into and out of the lungs and delivered to the alveoli for gas exchange. It can be divided into an active inspiratory phase and most often a passive expiratory phase. Contraction of the inspiratory muscles increases the volume of the chest cavity, thus reducing intrathoracic pressure and causing air to move down its pressure gradient from the mouth. To achieve this, the respiratory muscles must overcome the inherent elasticity of the respiratory system as well as resistance to gas flow in the airways. This resistance consists of elastic resistance of lung tissue and chest wall, resistance from surface forces at the alveolar gas/liquid interface, frictional resistance to gas flow through the airways, resistance to deformation of thoracic tissues, and finally a negligible component of inertia associated with movement of gas and tissue.
Expiration is usually a passive process during which the respiratory muscles relax, allowing the elastic tissues of the chest wall to return to their resting position. The point at which the tendency for the lung to contract equals the tendency of the chest wall to expand is the resting position of the respiratory system, the functional residual capacity (FRC).
Muscles of Ventilation
During inspiration, subatmospheric pressure occurs throughout the airway. Collapse of large airways is prevented by their cartilaginous structure and of small airways by the elasticity of surrounding lung tissue, but in the pharynx collapse can easily occur. In conscious individuals, upper airway collapse is prevented by a combination of both tonic muscle activity and phasic inspiratory contraction of the pharyngeal dilator muscles. This muscle activity is controlled via reflex stimulation of mechanoreceptors in the larynx and pharynx that respond to subatmospheric pressure by rapidly (<50 msec) activating the pharyngeal dilators. Similar responses in the laryngeal muscles result in abduction of the vocal cords during inspiration and adduction in expiration. Adduction of the cords in early expiration is believed to act to slow expiratory flow rate and thus help prevent alveolar collapse.
The diaphragm is the principal muscle for inspiration and is responsible for around three-quarters of the tidal volume during resting inspiration in the supine posture. Intercostal muscles also contribute to inspiration, particularly when in an upright position and when there is increased ventilation (e.g., with exercise or with dyspnea from lung pathology). The external intercostal and parasternal portions of the internal intercostal muscles are normally active during inspiration, but changes in posture and other activities involving movement of the trunk affect which muscles are active in each phase of respiration. Other muscles such as the scalenes and sternocleidomastoids can also contribute to inspiration.
When supine, the weight of the abdominal contents assists in passive expiration by pushing the diaphragm cephalad. When upright or while hyperventilating, however, the internal intercostals and abdominal wall muscles contract to assist expiration. Many of the respiratory muscles have additional functions, including maintenance of posture, coughing, sneezing, and speech. Each of these functions must be integrated with the muscles’ primary role in breathing.
The Airway
Gas flow within the airways is predominantly turbulent in the pharynx, larynx, and large airways, becoming more laminar in smaller airways as gas velocity decreases. For laminar flow, the flow rate is related to the fourth power of the radius (r 4 ) of a straight tube as described by the Poiseuille equation. Lung volume has a large effect on airway diameter, so the relationship between lung volume and airway resistance is hyperbolic. Airway resistance is low at FRC, and increasing lung volume further has little effect. In contrast, a small reduction in lung volume results in a large increase in resistance. The main sites of resistance within the airway are the nose and major bronchi. Speed of airflow rapidly decreases as air passes from large to small airways, with the aggregate cross-sectional area increasing to very large values after the eighth generation of airway (small bronchi, 1–2 mm in diameter).
In addition to conducting gas to and from alveoli the pharynx, larynx and large airways “condition” inspired gas by warming and humidifying each breath to fully saturated with water vapor at body temperature before the gas reaches the smaller airways. The airway lining is also responsible for defending the lungs from the myriad inhaled particles that would damage the alveoli or gain access to the body if they reached the gas exchanging regions of the lung. All of these functions are achieved by the airway epithelium producing airway lining fluid, which provides the water required for humidification and contains large amounts of a sticky glycoprotein called mucin, which is responsible for capturing inhaled particles and removing them from the lung. Excessive secretion of mucin or abnormal airway lining fluid function is a feature of many respiratory diseases, including smoking, chronic obstructive pulmonary disease, and cystic fibrosis, leading to often irreversible narrowing of larger airways.
Most airway diseases also involve reduction in the diameter of small airways as the result of bronchospasm and mucosal edema. This reduction in diameter in turn causes increased airway resistance and decreased gas flow. A large proportion of current pharmacotherapy for airway disease targets airway smooth muscle and inflammation in an attempt to increase airway diameter and gas flow.
Cellular Physiology
Most bronchial smooth muscle is found in the 12th to 16th generations of airway, which are bronchioles typically with a diameter of less than 1 mm. They have no cartilaginous support and their walls contain a high proportion of smooth muscle relative to luminal diameter.
Four mechanisms are involved in controlling muscle tone in small airways: neural pathways, humoral (via blood) control, direct physical and chemical effects, and local cellular mechanisms. Neural control is the most important in normal lung, with direct stimulation and humoral control contributing under some circumstances. Cellular mechanisms, particularly mast cells, have little influence under normal conditions but are important in airway disease.
Neural Control
The parasympathetic nervous system is the most important determinant of bronchomotor tone and when activated can completely obliterate the lumen of small airways. Both afferent and efferent nerve fibers travel via the vagus nerve (X) with efferent ganglia in the bronchial walls. Afferent nerves arise from receptors under the tight junctions of the bronchial epithelium and respond either to noxious stimuli acting directly on the receptors or to cytokines released by cellular mechanisms such as mast cell degranulation. Efferent nerves release acetylcholine, which acts at M 3 muscarinic receptors to cause contraction of airway smooth muscle, while also stimulating M 2 prejunctional muscarinic receptors to exert negative feedback on acetylcholine release. Stimulation of any part of the reflex arc results in bronchoconstriction. Some degree of resting tone is normally present and therefore permits a small degree of bronchodilation when vagal tone is reduced in a similar fashion to vagal control of heart rate.
In contrast to the parasympathetic system, the sympathetic system is poorly represented in the lung and not yet proven to be of major importance in humans. Indeed, it appears unlikely that there is any direct sympathetic innervation of airway smooth muscle.
The airways are provided with a third autonomic control system, the nerves of which are neither adrenergic nor cholinergic, and are referred to as noncholinergic parasympathetic nerves ( Fig. 29.1 ). This is the only potential bronchodilator nervous pathway in humans, though the exact role of these nerves remains uncertain. The efferent fibers run in the vagus nerve and pass to the smooth muscle of the airways where they cause slow (minutes) and prolonged relaxation of bronchi. The major neurotransmitter is vasoactive intestinal peptide, which produces airway smooth muscle relaxation by promoting production of nitric oxide (NO). How NO relaxes airway smooth muscle is not as fully understood as its effect on vascular smooth muscle, but resting airway tone does seem to involve NO mediated bronchodilation.

Humoral Control
Despite the minimal significance of sympathetic innervation, airway smooth muscle has plentiful β 2 -adrenergic receptors that are highly sensitive to circulating epinephrine and act via complex second-messenger systems (see Fig. 29.1 and described later). Basal levels of epinephrine probably do not contribute to airway muscle tone, but this mechanism is brought into play during exercise or during the sympathetic “stress response.” There are α-adrenergic receptors that cause bronchoconstriction, but these are not thought to be of physiologic significance.
Physical and Chemical Effects
Direct stimulation of the respiratory epithelium activates the parasympathetic reflex described previously, thereby causing bronchoconstriction. This is a protective reflex that evolved to prevent inhaled particles gaining access to the lower respiratory tract by effectively reducing airway size and air flow to distal lung units. This type of protection is necessary because the thin alveolar capillary membrane (to facilitate gas transfer) is a vulnerable interface between the internal milieu and the outside world. Physical factors known to produce bronchoconstriction include mechanical stimulation of the upper airway (e.g., by laryngoscopy) and the presence of a foreign body in the airway. Inhalation of particulate matter, an aerosol of water, or just cold air can also cause bronchoconstriction, particularly in patients with asthma. Many chemical stimuli also result in bronchoconstriction, including liquids with low pH such as gastric acid, and irritant gases such as sulfur dioxide, ammonia, ozone, and nitrogen dioxide.
Local Cellular Mechanisms
Inflammatory cells found in the lung include mast cells, eosinophils, neutrophils, macrophages, and lymphocytes. These inflammatory cells are stimulated by a variety of pathogens, but some can also be activated by the direct physical factors described earlier. Once activated, cytokine production causes amplification of the response, and a variety of mediators are released that can cause bronchoconstriction ( Table 29.1 ). These mediators are produced in normal individuals, but patients with airway disease are usually “hyperresponsive” and so develop bronchospasm more easily.
Source | Bronchoconstriction | Bronchodilation | ||
---|---|---|---|---|
Mediator | Receptor | Mediator | Receptor | |
Mast cells, other proinflammatory cells | Histamine | H 1 | Prostaglandin E 2 | EP |
Prostaglandin D 2 | TP | Prostacyclin (PGI 2 ) | EP | |
Prostaglandin F 2α | TP | |||
Leukotrienes C 4 , D 4 , E 4 | CysLT 1 | |||
PAF | PAF | |||
Bradykinin | B 2 | |||
C-fibers | Substance P | NK 2 | ||
Neurokinin A | NK 2 | |||
CGRP | CGRP | |||
Endothelial, epithelial cells | Endothelin | ET B |
Molecular Physiology
There are two opposing systems that control bronchial smooth muscle tone: signaling pathways resulting in increased intracellular cyclic adenosine monophosphate (cAMP), reduced intracellular calcium ions (Ca 2+ ), and bronchodilation, and pathways resulting in increased inositol triphosphate (IP 3 ), increased Ca 2+ , and bronchoconstriction.
Bronchodilator Pathway
These pathways are summarized in Fig. 29.1 . The molecular basis for the function of β 2 -adrenoreceptors is now clearly elucidated. This G-protein–coupled receptor contains 413 amino acids and has 7 transmembrane helices. The agonist binding site is deep within the hydrophobic core of the protein, which sits within the lipid bilayer of the cell membrane. This affects the interaction of drugs at the binding site. Lipophilic drugs form a depot in the lipid bilayer from which they can repeatedly interact with the binding site of the receptor, producing a longer duration of action than hydrophilic drugs. Receptors exist in either activated or inactivated form, the former state occurring when the third intracellular loop is bound to the α-subunit of the G s -protein. Agonists of the β 2 receptor probably do not induce a significant conformational change in the protein structure but rather stabilize the activated conformation, allowing this to predominate.
β 2 -Adrenoreceptor stimulation results in activation of G s , a guanosine triphosphate (GTP)-binding regulatory protein, which in turn activates adenylyl cyclase. This enzyme catalyzes the conversion of adenosine triphosphate (ATP) to cAMP. cAMP inhibits the release of Ca 2+ from intracellular stores in addition to activating protein kinase A, which in turn phosphorylates proteins regulating the interaction of actin and myosin. As a result of these actions, smooth muscle cells relax, leading to bronchodilation (see Fig. 29.1 ).
Intracellular cAMP is rapidly hydrolyzed by phosphodiesterase (PDE). Seven subtypes of PDE have been identified with subtypes 3, 4, and 7 predominating in the lungs. Several phosphodiesterase inhibitors are available (e.g., theophylline), which function by prolonging smooth muscle relaxation resulting from β 2 -receptor stimulation. However, older PDE inhibitors are nonspecific and as a result, their use in the treatment of bronchoconstriction is limited by side effects. Newer, more specific, inhibitors (e.g., of PDE subtype 7) may also have an antiinflammatory effect in lung disease.
Two β 2 -receptor genes are present in humans, with 18 polymorphisms described, giving rise to a large number of possible phenotypic variants. Some clinical differences are observed between variants, but the contribution of different β 2 receptor phenotypes to the overall prevalence of asthma appears to be minimal.
Bronchoconstrictor Pathway
Stimulation of M 3 acetylcholine receptors ( Fig. 29.2 ) results in the activation of a different G-protein (G q ) that in turn activates phospholipase C, stimulating the formation of IP 3 . IP 3 binds to receptors on the sarcoplasmic reticulum, promoting the release of Ca 2+ from intracellular stores. Myosin light chain kinase is activated by Ca 2+ and in turn phosphorylates myosin chains and activates myosin adenosine triphosphatase (ATPase). As a result, cross-bridges are formed between actin and myosin and contraction of smooth muscle occurs. IP 3 is then converted to inactive inositol diphosphate (IP 2 ) by IP 3 phosphatase. Other mediators of bronchoconstriction (e.g., tachykinin and histamine) exert their effects via similar mechanisms resulting in an increase in IP 3 production (see Table 29.1 ).

There are many interactions between the IP 3 and cAMP signaling pathways. Activation of G q also results in synthesis of diacylglycerol from phosphatidylinositol bisphosphate by phospholipase C. Diacylglycerol in turn activates protein kinase C, which phosphorylates many downstream proteins, including G-proteins and the intracellular domain of the β 2 adrenoreceptor itself. This causes the β 2 adrenoreceptor to become uncoupled from its G-protein, resulting in downregulation of the transduction pathway. Frequent stimulation of the receptor results in desensitization to its agonist via a similar mechanism.
Bronchoconstriction in Airway Disease
Bronchoconstriction as part of airway disease occurs as a result of complex interactions between proinflammatory cells and mediators. Mast cells are ubiquitous in airway epithelium and can be activated physically (e.g., by coughing) as well as by allergens and infection. Both allergens and infection exert their effects via immunoglobulin E, complement, and cytokines. The last of these cause chemotaxis and activation of other inflammatory cells, including neutrophils, macrophages, eosinophils, and lymphocytes, which in turn leads to augmentation of the inflammatory response.
Another key pathway in the generation of bronchoconstriction is modulated by the noncholinergic parasympathetic system described earlier. C-fiber nerve endings release inflammatory mediators, including tachykinins, which are thought to play an important part in the genesis of bronchial hyperresponsiveness.
The physiologic complexity of bronchoconstriction presents many potential therapeutic targets for the treatment of asthma (see Chapter 30 ). Steroids are the mainstay of treatment. Their antiinflammatory action reduces mucosal edema and swelling as well as smooth muscle tone, vascular permeability, and pulmonary vascular resistance. Glucocorticoids exert their effects by downregulating synthesis of certain proteins, leading to a reduction in inflammatory cytokines such as interleukin (IL)-1β, IL-6, IL-11, prostaglandins, bronchoconstrictor receptors such as neurokinin receptors, and inflammatory enzymes such as cyclooxygenase 2. In addition, glucocorticoids increase antiinflammatory cytokines and the numbers of bronchodilator receptors such as β 2 adrenoreceptors. Cytoplasmic glucocorticoid receptors consist of a single protein with two centrally bound zinc atoms. Agonists bind to the receptor within the cytoplasm, resulting in a conformational change that allows the receptor to enter the nucleus. The receptor then regulates DNA transcription by directly binding to specific regulatory DNA sequences and interacting with other transcription factors to modify gene expression. Steroid responsiveness varies between individuals and this might be due to the variable requirement for other transcription factors to interact with the glucocorticoid receptor.
There is increasing recognition that the inflammatory process underlying asthma development originates from abnormal immune responses to infection or allergens, with important roles for several subtypes of T “helper” (Th) lymphocytes. An early, oversimplistic view of this claimed that asthma developed simply because of an imbalance between the activity of proinflammatory Th2 lymphocytes and their antiinflammatory Th1 counterparts, but more recently the focus has moved to regulatory T cells and natural type-2 helper cells that influence the activity of both Th1 and Th2 cells. Irrespective of the exact cellular mechanisms, the numerous cytokines by which these cells communicate provide great therapeutic potential. Monoclonal antibodies that reduce the activity of the main proinflammatory cytokines in asthma, IL-5 and IL-13, are already available and have shown good outcomes in clinical trials in some patients.
Oxygenation
The partial pressure of oxygen (P o 2 ) of dry air at sea level is 159 mm Hg (21.2 kPa). Oxygen is diluted by water vapor and expired carbon dioxide as it passes through the respiratory tract and then moves down its partial pressure gradient across the alveolar capillary barrier into the blood, then from arterial capillary blood into tissues and cells. P o 2 finally reaches its lowest level in the mitochondria, where it is consumed in oxidative phosphorylation. At this point, the P o 2 is probably within the range of 3.8 to 22.5 mm Hg (0.5–3 kPa), varying within different tissues and cell types. This stepwise reduction in P o 2 from air to mitochondria is known as the oxygen cascade, and any step in the cascade can be affected under pathologic circumstances to result in tissue hypoxia (see Chapter 23 ).
There are about 300 million alveoli in the adult human lung. These thin-walled air sacs form the basic unit of gas exchange and are approximately 0.3 mm in diameter at FRC. Alveoli begin to appear in the lung at the level of the respiratory bronchioles, the level at which gas movement ceases to be tidal and moves instead by diffusion. The walls of alveoli are very thin, approximately 0.5 µm, to allow easy diffusion of respiratory gases. For gas to enter pulmonary capillaries it must first traverse the alveolar-capillary barrier. This consists of the alveolar lining fluid, alveolar epithelial cell, interstitial tissue, basement membranes, and finally the pulmonary capillary endothelium. To minimize the distance between capillary endothelium and red blood cells (RBCs), the diameter of the pulmonary capillaries is small so that the RBCs must flatten to pass, bringing the RBC membrane into close contact with the capillary wall. Diffusion occurs quickly across the blood-gas barrier so that RBCs become fully saturated with oxygen within 0.25 second of entering the pulmonary capillary. This is only a third of the time the cell spends in the capillary in a resting subject and means that a diffusion barrier is rarely a cause of poor oxygen uptake; the only examples in humans are extreme hypoxia at altitude or severe exercise in elite athletes.
Ventilation and Perfusion Relationships
Matching ventilation and perfusion is critical to optimize oxygen transfer. Gas exchange is optimal when ventilation and perfusion are evenly distributed throughout the lung. In a contrasting and extreme example, if 100% of perfusion is to one lung and 100% of ventilation is to the other lung, no gas exchange occurs even though total ventilation and perfusion might be normal.
Distribution of Ventilation
Ventilation to different lung regions is influenced by several factors. The right lung is bigger than the left and therefore receives a greater proportion of total ventilation in the upright and supine positions. The effect of gravity is also important. Lung tissue can be considered as semifluid or gel-like within the chest cavity. The weight of tissue above compresses tissues below, making the density of tissues at the bases greater than at the apices. The dependent bases are therefore less expanded and so more compliant, which in turn means they are better ventilated. With the body in the lateral position, the lower, dependent lung is better ventilated than the upper, nondependent lung, and similarly, in the supine position, the posterior part of the lung is better ventilated than the anterior part. Gravity is not the only influence, and airway distribution also affects ventilation. Scanning techniques with the ability to measure ventilation in areas of lung only a few milliliters in volume show increased ventilation in central compared with peripheral lung regions. This observation probably results from unequal branching patterns of the airways.
Distribution of Perfusion
The flow of blood through the pulmonary circulation equals the flow through the systemic circulation, from about 6 L/min under resting conditions to as much as 25 L/min during exercise. It is remarkable that such a range of flow can normally be achieved with minimal increase in pulmonary vascular pressures, owing to the considerably lower pulmonary vascular resistance (PVR) compared with that in the systemic circulation. PVR determines total blood flow to the lungs but the regional distribution of blood flow is not uniform. Flow progressively increases down the lung in an upright subject with flow per unit of lung volume increasing by 11% per centimeter of descent, but as for ventilation, gravity is not the only factor.
Total PVR is influenced by a variety of both passive and active factors. Passive factors include the following:
- 1.
Transmural pressure. If extravascular pressure is greater than intravascular hydrostatic pressure, the vessel will collapse, obstructing blood flow. This situation occurs in some lung regions during positive-pressure ventilation and can increase regional PVR and reduce perfusion.
- 2.
Lung volume. PVR is at its lowest at FRC. As lung volume increases, some pulmonary capillaries become narrowed and stretched, whereas a reduction in lung volume makes some capillaries more tortuous or kinked, both of which increase PVR.
- 3.
Vascular architecture. The branching pattern of the pulmonary vasculature might be responsible for gravity-independent variation in blood flow, known as the fractal hypothesis . Two aspects of vascular structure contribute to variations in flow. Because of the exponential relationship between flow and radius, bifurcations of pulmonary arteries into two slightly different-sized vessels has a large effect on the flow rates in each. In addition, pulmonary arteries are more numerous than pulmonary airways as a result of small extra branches, often given off at right angles, throughout the pulmonary arterial tree. Mathematical modeling indicates that these “supernumerary” branches contribute significantly to the heterogeneity of regional perfusion.
Ventilation in Relation to Perfusion
Ventilation can be related to perfusion using the ventilation-perfusion ratio (V̇/Q̇). If alveolar ventilation is 4 L/min and pulmonary blood flow is 5 L/min, then the V̇/Q̇ ratio equals 0.8. If ventilation and perfusion of all alveoli were the same, then V̇/Q̇ would be 0.8 throughout the lung. But ventilation and perfusion are not uniform for all the reasons described, and alveoli range from nonventilated to unperfused with all possible combinations in between. Alveoli with no ventilation (V̇/Q̇ ratio of 0) have P o 2 and partial pressure of carbon dioxide (P co 2 ) values that are the same as those of mixed venous blood because the trapped air in the nonventilated alveoli equilibrates with mixed venous blood. Alveoli with no perfusion (V̇/Q̇ ratio of ∞) have P o 2 and P co 2 values that are the same as humidified inspired gas because there is no gas exchange to alter the composition of the alveolar gas. All other alveoli have P o 2 and P co 2 values in between those of mixed venous blood and inspired gas depending on their V̇/Q̇ ratio ( Fig. 29.3 ).

Shunt
Admixture of pulmonary venous blood with poorly oxygenated or mixed venous blood is an important cause of systemic arterial hypoxemia. Venous admixture refers to the degree of admixture of mixed venous blood with pulmonary end-capillary blood that would be required to produce the observed difference between the P o 2 of arterial and pulmonary end-capillary blood. There are several sources of venous admixture. Physiologic sources include the venae cordis minimae (Thebesian veins) that drain blood from the myocardium directly into the chambers of the left heart and the bronchial veins that drain into the pulmonary veins. Pathologic causes include right-to-left shunting in congenital heart disease, particularly conditions involving obstruction of the right ventricular outflow tract (e.g., tetralogy of Fallot). More commonly, pulmonary pathology causes increased venous admixture, usually resulting from pulmonary blood flow past nonventilated or poorly ventilated alveoli in conditions such as pneumonia, atelectasis, and acute lung injury.
Effects of General Anesthesia
General anesthesia has a marked effect on V̇/Q̇ relationships. Following induction of anesthesia, changes in the dimensions of the thoracic cavity owing to muscle relaxation, in particular loss of tonic activity in the diaphragm, lead to a 10% to 20% reduction in FRC. As FRC approaches closing capacity, atelectasis occurs in the dependent parts of the lung ( Fig. 29.4 ), resulting in areas of lung that are perfused but not ventilated (V̇/Q̇ = 0), increasing shunt and therefore impairing oxygenation. Even in dependent areas without atelectasis, the V̇/Q̇ ratio is less than 1 and oxygenation poor. Atelectasis and areas with a low V̇/Q̇ ratio are more likely in patients whose closing capacity is already equal to or greater than FRC prior to anesthesia (e.g., in older patients, infants, and the obese).

In nondependent areas of lung the converse problem occurs. These areas are, relative to dependent regions, well ventilated. General anesthesia commonly leads to low cardiac output and pulmonary hypotension, resulting in reduced perfusion to nondependent regions. As a result of these two effects, V̇/Q̇ ratios in nondependent regions are normally greater than 1, and there are some areas with ventilation but no perfusion (V̇/Q̇ = ∞) which constitutes alveolar dead space. Without compensatory increases in ventilation, these regions of lung lead to reduced carbon dioxide elimination and give rise to the large difference between end-expiratory and arterial P co 2 commonly seen during general anesthesia. Although V̇/Q̇ ratios throughout the lung as a whole remain normal during general anesthesia, there is increased scatter of V̇/Q̇ ratios in different lung regions, leading to impairment of both oxygenation and carbon dioxide elimination.
Active Control of Pulmonary Vascular Resistance
In addition to the passive changes in PVR already described, pulmonary blood vessels are also under active control. The pulmonary vasculature is normally kept in a state of active vasodilatation. There are many mechanisms involved in the active control of PVR. Some vasodilators (e.g., prostaglandins and vasoactive intestinal peptide) act directly on smooth muscle cells via the cAMP second-messenger system. In contrast, vasoconstrictors activate G-protein-coupled receptors, leading to increased IP 3 . NO plays an important role in pulmonary blood vessels, and it is likely that NO acts as a final common pathway for relaxation of pulmonary vascular smooth muscle from a variety of different stimuli. Nitric oxide synthase (NOS) produces NO by converting L-arginine to L-citrulline. Pulmonary NOS exists in two forms: constitutive and inducible NOS. The inducible form (iNOS or NOS2) is produced by many cells in response to activation by inflammatory mediators. Constitutive NOS (cNOS or NOS3) is always present in some cells, including pulmonary endothelium, producing short bursts of NO in response to changes in Ca 2+ levels. NO diffuses from the site of production to the smooth muscle cell, where it activates guanylyl cyclase to produce cGMP, which in turn activates protein kinase G. This system is similar to the cAMP pathway and causes relaxation by a combination of effects on cytosolic Ca 2+ levels and the activity of enzymes controlling myosin activity.
Hypoxic Pulmonary Vasoconstriction
Vasoconstriction in response to hypoxia represents the fundamental difference of pulmonary blood vessels compared with systemic vessels. Hypoxic pulmonary vasoconstriction (HPV) is mediated both by mixed venous (pulmonary arterial) and alveolar P o 2 , with the greater influence from the alveolus.
Regional HPV is beneficial as a means of diverting pulmonary blood flow away from areas of lung with a low oxygen partial pressure and is an important mechanism for optimizing V̇/Q̇ relationships. It is also important in the fetus to minimize perfusion of the nonventilated lungs. However, long-term HPV, either continuous or intermittent, can lead to remodeling of the pulmonary vasculature and irreversible pulmonary hypertension.
The pressor response to hypoxia results from constriction of small arterioles of 30 to 200 µm in diameter and begins within a few seconds of P o 2 reduction. In humans, hypoxia in a single lobe of the lung results in a rapid decline in perfusion of the lobe such that within 5 minutes regional blood flow is half that during normoxia. With prolonged hypoxia, HPV in humans is biphasic: the initial rapid response reaches a plateau after about 5 minutes, with the second phase occurring after around 40 minutes and reaching a maximal response after 2 to 4 hours ( Fig. 29.5 ).

Cellular Physiology of Hypoxic Pulmonary Vasoconstriction
Neural connections to the lung are not required as HPV occurs in isolated lung preparations and in humans following lung transplantation. Attempts to elucidate the mechanisms of HPV have been hampered by species differences, the multitude of systems affecting pulmonary vascular tone, and a lack of appreciation of the biphasic nature of the response. Uncertainties remain regarding the exact cellular mechanisms of HPV, but there is now agreement that contraction of pulmonary artery smooth muscle cells (PASMCs) in response to hypoxia is an inherent property of these cells and that pulmonary endothelial cells act only to modulate the PASMC response.
Molecular Physiology
Hypoxia leads to a small increase in intracellular Ca 2+ concentration within the PASMCs, and also a rho-kinase–mediated increase in the Ca 2+ sensitivity of the smooth muscle contractile proteins. The change in Ca 2+ results from opening of L-type voltage-gated Ca 2+ channels (Ca v 1), stimulated by hypoxia-induced inhibition of voltage-gated K + (K v ) channels. The molecular oxygen sensor that affects K v channels remains controversial and might be an inherent property of the K v channels, although mitochondria, nicotinamide adenine dinucleotide phosphate oxidases, and reactive oxygen species are also involved.
Modulation by the endothelial cell of the PASMC response to hypoxia can either enhance or inhibit HPV. Inhibitors of HPV include prostacyclin and NO, both of which maintain some perfusion of hypoxic lung regions, although their role in normal lung is uncertain. For example, prostacyclin is a potent pulmonary vasodilator, but cyclooxygenase, which is required for its production, is inhibited by hypoxia and might therefore diminish its vasodilator effects. Similarly, basal NO secretion by endothelial cells might act to moderate HPV, but hypoxia also inhibits endothelial NO production and so enhances HPV. Molecules that enhance HPV include thromboxane A 2 and endothelin; the latter is released by endothelial cells in response to hypoxia. It is a potent vasoconstrictor peptide, and has a prolonged effect on pulmonary vascular tone such that this mechanism is probably involved in the second slow phase of HPV (see Fig. 29.5 ). Two groups of endothelin receptors are described, ET A and ET B , and their relative expression varies between the central and peripheral pulmonary vasculature. Apart from its vasoconstrictor effects, endothelin also stimulates cellular proliferation of vascular endothelial cells and pulmonary fibroblasts, and so has an important role in the pulmonary vascular remodeling that accompanies long-term hypoxia.
Iron status affects the intensity of an individual’s HPV response. Increased iron availability (achieved by intravenous infusion) attenuates HPV, whereas reducing iron availability by administration of desferrioxamine enhances the response. This finding indicates that HPV in humans is at least partially mediated via hypoxia-inducible factor, a ubiquitous transcription factor that is activated by hypoxia to initiate a range of responses to protect the cell from damage. One of the crucial oxygen-dependent steps in this reaction requires iron as a cofactor, explaining why iron status influences HPV. These observations might be highly significant for patients given that normal subjects have widely varying iron status, depending on such factors as gender, diet, and chronic illness, and also open up the possibility of therapeutic interventions for pulmonary hypertension or hypoxic critically ill patients.
HPV is therefore a complex and poorly elucidated reflex. In normal subjects, HPV within the lung is inhomogeneous, with intense vasoconstriction in some areas and relative overperfusion elsewhere. The degree of this inhomogeneity varies between individuals, and this has important implications when traveling to high altitude. Subjects susceptible to high-altitude pulmonary edema have a more intense and inhomogeneous HPV response. Although this has never been studied, it raises the possibility that the same subgroup of patients would tolerate hypoxia related to lung disease poorly.
Control of Breathing
Early in fetal development the respiratory center forms within the brainstem and is responsible for subconscious rhythmic breathing throughout life. Breathing is controlled by a complex network of physical and chemical reflexes that can be overridden by voluntary control and interrupted by acts such as swallowing, sneezing, vomiting, hiccupping, and coughing. The control system can also be fine-tuned to accommodate for posture, speech, voluntary movement, and exercise. The respiratory pattern is generated within the medulla and coordinates the voluntary and involuntary demands of respiratory activity via multiple neuronal connections.
Respiratory Center
There are two main groups of respiratory neurons: the dorsal and ventral respiratory groups.
The dorsal respiratory group lies close to the nucleus tractus solitarius, which is the area of the brain where visceral afferents from cranial nerves IX and X terminate. This group consists mainly of inspiratory neurons and is responsible for timing of the respiratory cycle.
The ventral respiratory group is a column of respiratory neurons divided into four subgroups. The caudal group has both inspiratory and expiratory functions in addition to controlling the force of contraction of the contralateral inspiratory muscles. The rostral subgroup is composed mainly of the nucleus ambiguus and controls the airway dilator functions of the larynx, pharynx, and tongue. The pre-Bötzinger complex is believed to be the anatomic location of the central pattern generator (CPG), while the Bötzinger complex is within the nucleus retrofacialis and has widespread expiratory functions.
Central Pattern Generation
Unlike the heart, where inherent rhythm is initiated by a single pacemaker cell, respiratory rhythm begins with associated groups of neurons generating regular bursts of activity. This activity can be recorded throughout the medulla but is concentrated in the pre-Bötzinger complex. Respiratory neuron networks that exhibit spontaneous activity achieve this by a combination of intrinsic membrane properties and excitatory and inhibitory feedback mechanisms. In practice, both inhibitory and excitatory neurotransmitters have a dual effect to (1) recruit other cells by direct activation and (2) modulate the spontaneous activity of a single cell by effects on its own membrane ion channels—for example, by slowing the rate at which an action potential travels along a dendrite.
Connections to the Respiratory Center
The pontine respiratory group, previously known as the pneumotaxic center, was believed to be important in controlling the timing of the respiratory cycle. It is no longer considered essential for generation of the respiratory rhythm but exerts fine control over medullary neurons—for example, setting the lung volume at which inspiration is terminated. The pons also coordinates input from other centers (e.g., the hypothalamus, cortex, and nucleus tractus solitaries), integrating cortical and peripheral sensory information, such as odors, temperature, and visceral and cardiovascular inputs with the respiratory rhythm. Voluntary interruption of breathing is possible within limits determined by arterial blood gas tensions. This is necessary for conscious activities such as speech. It is possible that cortical signals bypass the respiratory center, overriding it by acting directly on the lower motor neurons supplying respiratory muscles.
A number of different peripheral receptors also provide input to the respiratory center. Chemical and irritant receptors are found in the nasopharynx, larynx, and trachea. When stimulated by irritants such as smoke and gastric acid, a reflex is initiated that protects the lungs from inhalational injury, resulting in sneezing, coughing, laryngeal closure, apnea, or bronchoconstriction. The Paintal juxtapulmonary capillary receptors (J receptors) are small C-fiber endings in alveolar walls close to pulmonary capillaries. Stimulation can occur in response to pulmonary vascular congestion, pulmonary embolus, or exercise and results in rapid, shallow breathing; apnea; and mucus secretion.
Pulmonary stretch receptors can be divided into two groups. Slowly adapting stretch receptors are situated in bronchial smooth muscle and are stimulated by lung inflation, maintaining their firing rate when lung inflation is sustained and thus acting as a lung volume sensor. Rapidly adapting stretch receptors are located in the superficial mucosal layer of the airways and are stimulated by a change in tidal volume, respiratory frequency, or lung compliance. Stimulation of pulmonary stretch receptors leads to inhibition of inspiration in response to lung distention and a reduction in respiratory rate by increasing expiratory time. This reflex was first described in 1868 by Hering and Breuer, who noted that lightly anesthetized, spontaneously breathing animals ceased or decreased ventilation effort during sustained lung distention. Although this weak reflex has little clinical significance in adult humans, it is more pronounced in neonates and infants.
Baroreceptors located in the carotid sinus and aortic arch are primarily concerned with regulation of the circulation, but can promote hyperventilation in response to a large decrease in arterial pressure.
Molecular Physiology
There are a variety of different neurotransmitters involved in the CPG and respiratory control. Excitatory transmitters include glutamate, which activates both N-methyl- d -aspartate (NMDA) and non-NMDA type receptors. Inhibitory transmitters such as glycine and gamma-aminobutyric acid (GABA) act via glycine and GABA A receptors to hyperpolarize and inhibit respiratory neurons.
Neuromodulators influence CPG output but are not involved in rhythm generation. The exact role of neuromodulators is unclear but they are important for both normal and abnormal breathing. For example, opioids have a profound effect on breathing, suggesting the presence of opioid receptors in the respiratory center. However, the opioid antagonist naloxone has no effect on respiration in resting normal subjects. Other neuromodulators include acetylcholine, serotonin, and substance P. They exert their effects via final common intracellular signaling pathways within CPG neurons involving protein kinase A and protein kinase C, modulating the activity of GABA, glycine, and glutamate-regulated potassium ion (K + ) and chloride ion (Cl − ) channels.
Chemical Control of Ventilation
For many years it was believed that the respiratory center itself was sensitive to carbon dioxide. However, it is now known that both peripheral and central chemoreceptors are responsible for the effect of carbon dioxide on breathing, the latter accounting for about 80% of the total ventilatory response. Because of their reliance on extracellular pH (see later text), the central chemoreceptors are regarded as monitors of steady-state arterial P co 2 and tissue perfusion in the brain, while the peripheral chemoreceptors respond more to short-term and rapid changes in arterial P co 2 .
Central Chemoreceptors
Central chemoreceptors are located 0.2 mm below the ventrolateral surface of the medulla in the retrotrapezoid nucleus inside the blood brain barrier (BBB). As arterial P co 2 rises, so does the CO 2 content of cerebrospinal fluid (CSF). This occurs because the BBB is permeable to CO 2 but not to hydrogen ions. CO 2 diffuses across the BBB into the CSF, where it is hydrated to carbonic acid, which quickly ionizes to increase CSF hydrogen ion concentration and hence reduce pH. The mechanism by which a change in pH causes stimulation of chemoreceptor neurons remains controversial: the retrotrapezoid nucleus might contain pH-sensitive K + channels, and release of ATP has been proposed. Increases in arterial P co 2 lead to an increase in respiratory rate and depth of breathing until steady-state hyperventilation is achieved after a few minutes. The response is linear over the range that is usually studied, with alveolar ventilation increasing by around 2 L/min for each 1 mm Hg increase in arterial P co 2 ( Fig. 29.6 ). As P co 2 continues to rise, the point of maximal ventilatory stimulation is reached, probably in the range of 100 to 200 mm Hg (13.3–26.7 kPa). Beyond this point, respiratory fatigue and CO 2 narcosis occur.

Decreasing P co 2 produces a fall in alveolar ventilation, but once arterial P co 2 is less than about 30 mm Hg (4 kPa), there are two possible physiologic responses. Some individuals reduce ventilation further, becoming apneic if P co 2 continues to fall, while others continue to breathe regardless of the reduction in P co 2 . This variable ventilatory response to low P co 2 almost certainly arises from cortical control of respiration, maintaining breathing despite a lack of chemical drive, particularly when awake (see Fig. 29.6 ).
The P co 2 /ventilation response curve is the response of the entire respiratory system to the challenge of raised P co 2 . Apart from reduced sensitivity of the central chemoreceptors, the overall response can be blunted by partial neuromuscular blockade or by obstructive or restrictive lung disease. These factors must be taken into account when drawing conclusions from a reduced response; for example, severe airway obstruction will cause a blunted response to P co 2 even when the central response is normal. Nevertheless, the slope of the P co 2 /ventilation response curve remains one of the most valuable parameters in the assessment of the responsiveness of the respiratory system to CO 2 and its depression by drugs.
Peripheral Chemoreceptors
The peripheral chemoreceptors are the fast-responding monitors of arterial blood located in the carotid bodies close to the bifurcation of the common carotid artery. The carotid bodies contain large sinusoids with a very high rate of perfusion, about 10 times that predicted by their metabolic rate, which is itself very high. This results in a small arterial/venous P o 2 difference, which allows for a rapid (1- to 3-second) response to changes in arterial blood P o 2 . Increased ventilation in response to activation of peripheral chemoreceptors occurs in response to various stimuli:
- 1.
Reduced arterial P o 2 . Glomus (type I) cells within the carotid body are in synaptic contact with neurons of the glossopharyngeal nerve. Discharge rate in the afferent nerves from the carotid body increases exponentially in response to falling arterial P o 2 .
- 2.
Acidemia. Elevated arterial P co 2 or hydrogen ion (H + ) concentration both stimulate ventilation; the magnitude of the stimulus is the same whether the change in pH is due to a respiratory or metabolic cause. Quantitatively, the change produced by elevated P co 2 on the peripheral chemoreceptors is only about one-sixth of that caused by the action on the central chemosensitive areas. This response does, however, occur very rapidly and develops only when a “threshold” value of arterial P co 2 is exceeded.
- 3.
Hypoperfusion. Stimulation of peripheral chemoreceptors by hypoperfusion can occur as a result of severe systemic hypotension, possibly by causing a “stagnant hypoxia” of the chemoreceptor cells.
- 4.
Hyperthermia. An increase in body temperature increases the rate of firing from peripheral chemoreceptor neurons.
Chemical stimulation by a wide range of substances causes increased ventilation via the peripheral chemoreceptors. These substances fall into two groups. The first group consists of agents such as nicotine and acetylcholine that stimulate sympathetic ganglia. The second group of chemical stimulants consists of substances such as cyanide and carbon monoxide that block the cytochrome system and so prevent oxidative metabolism. Some drugs also stimulate respiration via the peripheral chemoreceptors, for example, doxapram.
Molecular Physiology
There is now general agreement that oxygen-sensitive K + channels are responsible for the hypoxic response of the peripheral chemoreceptor type I cells. Hypoxia inhibits the activity of K + channels, altering the membrane potential of the cell and stimulating Ca 2+ channels to open, allowing an influx of extracellular Ca 2+ that stimulates transmitter release. Stimulation of the chemoreceptors by increased arterial P co 2 is dependent on carbonic anhydrase, present in the type I cell, and there is therefore the possibility of both raised P co 2 and decreased arterial pH acting through an increase in intracellular H + concentration, as in the central chemoreceptors.
Various neurotransmitters have been identified within the carotid body with dopamine, acetylcholine, and ATP being the most prominent. Norepinephrine, angiotensin II, substance P, and enkephalins have also been described, although the specific role of each is uncertain. Acetylcholine and ATP are the most likely neurotransmitters involved in signaling between type I cells and afferent nerves. The other transmitters seem to have autocrine rather than neurotransmitter roles, in that their release into the carotid body tissues modulates the response of the cells to various stimuli. For example, dopamine is abundant in type I cells and released in response to hypoxia; its presence causes inhibition of Ca 2+ channels so it effectively “dampens” the acute response. Similarly, the α 2 -adrenoceptor agonist clonidine reduces the ventilatory response to acute hypoxia, indicating that norepinephrine also has an inhibitory effect. Angiotensin II increases the sensitivity of the K + channels to hypoxia, and can be produced locally within the carotid body in response to long-term hypoxia or poor carotid body perfusion, as seen in heart failure.
The gain of the carotid bodies is under nervous system control. There is an efferent pathway in the carotid sinus nerve, which, on excitation, decreases chemoreceptor activity. Excitation of the sympathetic innervation to the carotid body causes an increase in activity.
Ventilatory Response to Sustained Hypoxia
Moderate degrees of sustained hypoxia, with arterial oxyhemoglobin saturation (Sa o 2 ) of about 80%, result in a triphasic physiologic response. The initial acute hypoxic response is an immediate and rapid increase in ventilation. Sudden imposition of hypoxia results in stimulation of ventilation within the lung-to-carotid body circulation time (about 6 seconds). Ventilation continues to increase for 5 to 10 minutes, rapidly reaching high levels. Shortly after the acute hypoxic response reaches a peak, there is a period of hypoxic ventilatory decline (HVD) during which the minute ventilation falls, reaching a plateau level, still above the resting ventilation, after 20 to 30 minutes ( Fig. 29.7 ). The individual degree of HVD correlates with the acute hypoxic response: the greater the initial increase in ventilation, the greater the subsequent decline. Although not completely elucidated, the mechanism of HVD appears to have a significant central component, and HVD represents a change in ventilatory drive rather than a decline in the sensitivity of receptors to hypoxia. Once HVD is complete, continued isocapnic hypoxia results in a second, slower rise in ventilation over several hours (see Fig. 29.7 ). Ventilation continues to increase for at least 8 hours and reaches a plateau by 24 hours. The most likely explanation for this is a direct effect of hypoxia on the carotid bodies, possibly mediated by angiotensin II.

Emerging Developments
Carbon Dioxide Oscillations and Control of Ventilation
For decades the traditional teaching on the roles of carbon dioxide and oxygen in respiratory control has remained unchanged despite certain anomalies. For example, ventilation increases massively during exercise with no measurable change in arterial blood gas partial pressures. With resting ventilation, gas movement in and out of an alveolus is by diffusion, but as tidal volume increases, gas movement becomes cyclical. As a result, the P co 2 of alveolar gas, and later arterial blood, begins to oscillate in phase with respiration, while the mean values remain unchanged. These oscillations of P co 2 are sensed by the peripheral chemoreceptors and enhance respiratory drive, in effect providing positive feedback to breathing to maintain normal mean arterial P co 2 even when metabolic production of carbon dioxide is very high. This system is believed to contribute to the ventilatory response to exercise and is particularly important if the subject is also hypoxic (e.g., at high altitude).
Asthma Phenotypes and Targeted Therapies
Both host and environmental factors contribute to developing asthma, including a complex heritable component, with multiple genes involved in its development. There are also genetic effects on a patient’s responsiveness to treatment. The pharmacogenetics of the β 2 adrenoceptor have been described above and racial differences have also been observed. For example, individuals of African racial background are less responsive to short-acting bronchodilators compared with Mexican Americans and Puerto Ricans. Other genes may modify the response to corticosteroids and leukotriene receptor antagonists. Understanding asthma genetics may also help develop biomarkers of disease phenotype and treatment; for example, genetic variation in the IL-6 receptor is associated with worse lung function and a more severe asthma phenotype.
However, asthma is not a single disease, but more a heterogeneous group of underlying disease processes evidenced by a wide variety of presentations, physiologic characteristics, and patient outcomes. As a result, the concept of asthma phenotyping has emerged, which involves integrating biological and clinical features with the ultimate goal of improving therapy.
The most common phenotypes identified include the following:
- •
Allergic asthma. Onset in childhood, associated with a family history of atopy, sputum examination typically showing eosinophilic inflammation.
- •
Nonallergic asthma. Adult onset, not associated with allergy, sputum examination may reveal neutrophilia, eosinophilia, or contain few inflammatory cells.
- •
Late-onset asthma. Presents in adult life, more commonly in women, tends to manifest as nonallergic wheezing.
- •
Asthma with fixed airflow limitation. Patients with longstanding asthma develop fixed airflow limitation, thought to be secondary to airway remodeling.
- •
Asthma with obesity. Obese patients with prominent respiratory symptoms and little eosinophilic airway inflammation.
By classifying the characteristics of each phenotype, it is hoped that a more targeted approach to treatment and a better understanding of the clinical course for an individual patient may be possible. In patients with severe disease, some phenotype-guided treatments are already available. Eosinophilic inflammation, allergic processes, and obesity have been identified as phenotypes that may be helpful when considering both nonspecific (corticosteroids) and more immune-targeted therapies including anti–IL-5 and anti–IL-13 antibody treatments. From the phenotypes discussed earlier it can be seen that the age of onset of asthma is linked to differences in allergic tendency and eosinophilic airway inflammation. Assessing the level of eosinophilic or Th2 lymphocyte–induced inflammation has the potential to predict a patient’s response to corticosteroid therapy and perhaps to more targeted treatments. Guidance from the 2016 Global Initiative for Asthma (GINA) now incorporates some phenotype-specific management for the treatment of severe asthma, but aside from this example there is no strong relationship between phenotype and response to therapy.
Remodeling of Airways
Repeated inflammation in small airways results in morphologic changes to airway smooth muscle, vascular, and epithelial cells ( Table 29.2 ). Hyperplasia of smooth muscle cells thickens the airway wall, even when the muscle is relaxed, and increases the degree of airway narrowing that occurs even with small amounts of muscle contraction. These structural changes not only affect airway mechanics, but can also contribute to inflammation themselves through the release of proinflammatory and growth factors. Autopsy and biopsy studies have linked increased amounts of smooth muscle to asthma severity, degree of airway obstruction, and bronchial hyperresponsiveness. Fibrocytes, which may differentiate into myofibroblasts, are also increased in the blood and smooth muscle bundles of patients with asthma who have fixed airway obstruction and/or severe disease. Airway remodeling in asthma patients may result in relatively irreversible narrowing of the airways. The morphologic changes may represent attempted tissue repair in response to chronic inflammation or may occur independently themselves. Remodeling can begin before asthma becomes severe, or is even diagnosed at all, and even though reducing airway inflammation with steroids can delay remodeling, drugs to reverse the structural changes are yet to be discovered.
Tissue | Changes in Asthma |
---|---|
Subepithelial fibrosis | Deposition of collagen fibers and proteoglycans under the basement membrane: seen in most patients with asthma even before symptom onset. Fibrosis can occur in other layers of the airway wall. |
Thickened airway smooth muscle | Consequence of hypertrophy and hyperplasia and contributes to increased thickness of airway wall. Process may relate to disease severity. |
Increased blood vessels in airway walls | Asthma patients have exaggerated influence of growth factors such as vascular endothelial growth factor, possibly mediated by Th2 cells. Increased vascularity may contribute to increased airway wall thickness. |
Mucus hypersecretion | Results from increased number of goblet cells in epithelium and increased size of submucosal glands. |
Key Points
- •
The diaphragm is the principal muscle for inspiration and is responsible for 75% of tidal volume during resting inspiration in the supine posture. In upright postures activity of the intercostal muscles accounts for the majority of inspiration. Expiration is normally a passive process but when ventilation increases (e.g., in response to exercise), expiration becomes active with contraction of the internal intercostal and abdominal muscles.
- •
Most bronchial smooth muscle is found in the 12th to 16th generations of the airway. Four mechanisms are involved in controlling muscle tone in small airways: neural, humoral (via blood), direct physical and chemical, and local cellular. Neural control is the most important in normal lung.
- •
Overall ventilation and perfusion are well matched and approximately equal, but in smaller regions of lung there are discrepancies between the two that can adversely affect gas exchange. For example, during general anesthesia there is a wider than normal spread of V̇/Q̇ ratios throughout the lung that impairs both oxygenation and carbon dioxide elimination.
- •
Regional HPV is beneficial as a means of diverting pulmonary blood flow away from areas of lung with low P o 2 and is an important mechanism for optimizing V̇/Q̇ relationships. Long-term HPV, either continuous or intermittent, leads to remodeling of the pulmonary vasculature and irreversible pulmonary hypertension.
- •
Uncertainties remain regarding the exact cellular mechanism of HPV, but there is now agreement that contraction of pulmonary artery smooth muscle cells in response to hypoxia is involved.
- •
Breathing is controlled by a complex network of physical and chemical reflexes that can be overridden by voluntary control and interrupted by acts such as swallowing, sneezing, vomiting, hiccupping, and coughing. The respiratory pattern is generated within the medulla, which coordinates the voluntary and involuntary demands of respiratory activity via multiple neuronal connections.
- •
Reflex control of breathing occurs in response to both carbon dioxide and oxygen. Minute volume of ventilation increases in a linear fashion in response to increasing P co 2 , a response that is highly variable between individuals. The response to hypoxia is exponential in nature such that below a P o 2 of approximately 60 mm Hg (8 kPa), the ventilatory response curve is very steep.
Anatomy and Imaging: The Thoracic Wall, Intercostal Space, and Thorax
- Jeffrey D. Swenson
- John Skaggs
This special section on Anatomy and Imaging outlines important anatomic structures and imaging techniques for the thoracic wall, intercostal space, lungs, and bronchial tree. Key structures are reviewed in anatomic drawings, plain radiographs, computed tomography (CT) scans, ultrasound (US), and bronchoscopic images. Modern imaging techniques that enable “real-time” visualization of relevant clinical anatomy are emphasized.
The left panel of Fig. AI3.1 illustrates that the external intercostal muscle extends from the superior margin of the rib below to the inferior margin of the rib above but does not overlie the outer cortex of the rib. This important distinction can be used to differentiate the external intercostal muscle from other muscles that overlie the thoracic wall.

The drawing also highlights the location of the major neurovascular bundle supplying the intercostal muscles, ribs, and chest wall. Because of the neurovascular bundle’s location inferior to the rib, chest tube thoracostomy is performed superior to the rib in the targeted intercostal space, thereby avoiding injury to the neurovascular bundle.
In the right panel , note the structural transition of the intercostal muscles between the anterior and posterior thoracic walls. The internal intercostal muscle transitions from a membranous layer in the posterior wall to a muscular layer in the anterior wall. By contrast, the external and innermost intercostal muscles exist as muscular layers in the posterior wall but transition to membranous layers in the anterior thoracic wall. This transition is important since US images of the intercostal space may not reveal 3 distinct muscular layers.
The drawing also highlights important features of the intercostal nerve . At approximately the level of the midaxillary line, the intercostal nerve gives rise to the lateral cutaneous branch . Thus, intercostal injections performed in the posterior thorax produce sensory changes across the entire chest wall. By contrast, injections performed anterior to the division of the lateral cutaneous branch produce limited sensory changes over the medial aspect of the anterior chest wall.
An ultrasound image of the posterior intercostal space between the 7th and 8th ribs is shown ( Fig. AI3.2 ). The position of the transducer is demonstrated in the inset picture. A line with 2 arrowheads marks the confluence of the innermost , internal , and external intercostal muscles extending from the pleura to the outer cortex of the rib. Note that the external intercostal muscle does not extend over the outer cortex of the ribs. The serratus anterior muscle is superficial to the outer cortex of the ribs at this level. This image also shows the clear border of pleura deep to a confluence of the external , internal , and innermost intercostals . Note the position of the intercostal muscles relative to the outer cortex of the ribs and position of the serratus anterior muscle immediately superficial to the outer cortex .

Two normal posterior-anterior (PA) chest radiographs are shown ( Fig. AI3.3 ). The image on the left has been labeled to highlight normal anatomic structures. The standard PA technique for radiographs of the chest is obtained with the patient upright and facing the digital image recorder. When examining a chest radiograph, it is important to know whether an alternative technique has been used (e.g., portable anterior-posterior film), since this may influence the interpretation.

Several important anatomic structures visible on the standard PA chest radiograph are demonstrated. The trachea is normally midline and passes slightly right of the aorta at the level of the carina . Lung markings extend to the chest wall, below the diaphragm, and behind the heart. The area overlying the medial border of the scapula may appear to be without lung markings; however, careful examination reveals lung markings in this region. The appearances of the anterior and posterior ribs are distinctive. The posterior ribs are more readily visible and oriented relatively horizontal to the thorax. By contrast, anterior ribs are less prominent and are angled medially and inferior relative to the thorax. The cardiac silhouette shows the right border as the right atrium and the left border as the left ventricle . In a PA view, the ratio of the cardiac width to thoracic width should be less than 1 : 2. The right and left hemi-diaphragms are domed structures overlying the liver and stomach, respectively. The right hemi-diaphragm is slightly higher than the left. A stomach bubble may be visible below the left hemi-diaphragm . The costophrenic angle (CPA) should be a sharp structure visible in the right and left hemi-thorax between the chest wall and the dome of the diaphragm. The arch of the aorta is visible at the left mediastinal border as it passes posteriorly over the left mainstem bronchus and pulmonary vasculature. The descending aorta is visible behind the cardiac silhouette.
The left panel is a bronchogram with contrast material outlining the trachea and major bronchi ( Fig. AI3.4 ). This image includes the right main bronchus (RMB) , left main bronchus (LMB) , right superior bronchus (RSB) , intermediate bronchus (IB) , right middle bronchus (RMB) , and right inferior bronchus (RIB) . The left main bronchus (LMB) , left superior bronchus (LSB) , and left inferior bronchus (LIB) are also displayed. Note the disparity in length between the RMB and LMB . The RMB is typically 2 to 2.5 cm in length as a result of the proximal origin of the RSB . In rare cases, the RSB may arise directly from the trachea. By contrast, the LMB is approximately 5 cm in length.

The right upper , middle , and lower panels are corresponding flexible bronchoscopic views of the structures seen in the bronchogram. The upper panel shows a view of the RMB and LMB from a position approximately 1 cm above the carina. In the right middle panel, the bronchoscope has been advanced to the carina. At this level, the RMB and RSB are completely visible. The entire length of the LMB can also be seen. The shorter distance between the carina and the orifice of the RSB is evident and has important implications for lung isolation procedures.
The right lower panel is a bronchoscopic view from a position 2 cm into the LMB . At this position, the LSB and LIB are visible.
Computed tomographic pulmonary angiography (CTPA) is used in patients with a high probability of pulmonary embolus ( Fig. AI3.5 ). Ventilation–perfusion scans are now reserved for patients who have a contraindication to CTPA or where CTPA is unavailable. This particular CTPA image shows major vessels of the thorax, including the superior vena cava (SVC) , ascending aorta (AA) , descending aorta (DA) , main pulmonary artery (MPA) , right pulmonary artery (RPA) , and left pulmonary artery (LPA) . The right main bronchus (RMB) and left main bronchus (LMB) are also visible. The red arrow highlights a thrombus in the RPA that is visible as a filling defect in that vessel.

The image illustrates a point-of-care technique used to diagnose pleural effusion ( Fig. AI3.6 ). The dependent (posterior-lateral) surface of the thorax is imaged using a phased array ultrasound transducer. The inset shows the transducer position used to acquire the image. The lung is displaced by pleural fluid (PF) , visualized as echogenic tissue. The displaced lung is outlined by a yellow border. Point-of-care evaluation of pleural effusion using surface ultrasound can provide both a qualitative and quantitative assessment with sensitivity and specificity equal to or exceeding that of conventional chest radiography.

A parasternal image of the thorax (using a linear ultrasound transducer) demonstrates the correct position of the transducer to evaluate potential pneumothorax ( Fig. AI3.7 ). The visceral pleural edge , ribs , and intercostal and pectoralis muscles are labeled. During real-time ultrasound imaging, the visceral pleural edge has a characteristic sliding motion in the normal examination. When a pneumothorax is present, “lung sliding” is not seen. Pneumothorax is another important pathologic condition that can be rapidly diagnosed at the bedside using surface ultrasonography. Its sensitivity and specificity for diagnosing pneumothorax exceeds that of plain chest radiography but is somewhat less than that of computed tomography.

Physics: Blood Gas Measurement
- Kai Kuck
Outline
Blood Gas Measurement Instrumentation
Measurement of Oxygen Tension: Clarke (Polarographic) Oxygen Electrode
Measurement of pH: Glass Electrode
Background
Assessment of arterial blood pCO 2 , pH, and pO 2 is routinely performed during anesthesia and critical care. In combination with respiratory gas measurements and pulse oximetry, it allows evaluation of cardiorespiratory function and metabolism. The most commonly used approaches require the sampling of arterial blood and in vitro analysis in a laboratory or point-of-care instrument. Understanding principles and limitations of the blood gas instrumentation is important to guide the correct interpretation of the measurements.
Blood Gas Measurement Instrumentation
Measurement of Oxygen Tension: Clarke (Polarographic) Oxygen Electrode
Most of the oxygen in blood is bound to hemoglobin. The small amount of unbound, dissolved oxygen is determined by the oxygen tension , abbreviated pO 2 , which is the partial pressure of the dissolved oxygen. Reported in millimeters of a mercury column (mm Hg) or kilopascals (kPa), arterial pO 2 normally ranges between 70 and 100 mm Hg in subjects breathing air at sea level. Oxygen tension and oxygen saturation (the percent of hemoglobin bound to oxygen) are related to each other by the oxyhemoglobin dissociation curve (see Chapters 24 and 29 and Fig. P9.5 ).
Oxygen tension is conventionally measured by the polarographic oxygen electrode developed in 1956 by Leland Clark. It consists of a platinum cathode and a silver/silver chloride anode in a buffered electrolyte solution of potassium chloride. A gas permeable membrane separates the sensor from blood. Oxygen tension in blood equilibrates with the electrolyte solution ( Fig. P7.1 ). At the anode, silver is oxidized and combines with the electrolytes to form silver chloride. The resulting electrons at the platinum cathode reduce oxygen, which combines with water to form hydroxide ions. The current from anode to cathode is measured and is proportional to the oxygen tension in the electrolyte solution and, thus, in the sample (blood). The electrochemical reaction requires a small voltage of 0.6 V to be applied externally. Both the electrodes and the electrolyte are changed through the electrochemical process such that the sensor must be replaced every few months. The electrochemical reactions in the Clarke electrode are temperature dependent and normally the electrode is kept at a constant temperature of 37°C (98.6°F), because temperature has an influence on pO 2 . An experimentally determined correction for the temperature effect in whole blood has been described:

pO 2 is the pO 2 at 37°C (98.6°F), in kPa. If mm Hg is used for pO 2 , the dividend 19.2 kPa should be changed to 144 mm Hg.
ΔT is the difference in °K between 37°C and the actual patient temperature

Erroneous readings can result from perforations of the membrane, interference with other gases (e.g., the electrode can read falsely high in the presence of N 2 O), depletion of the electrodes or the electrolyte, and contamination by proteins or blood products. Alternatives to the Clarke electrode include optical measurements (e.g., based on phosphorescent quenching). Some of these approaches lend themselves to placement on catheter tips for continuous in vivo measurements.
Measurement of pH: Glass Electrode
The pH is a logarithmic representation of the hydrogen ion concentration of a solution, (i.e., its acidity or alkalinity). It is dimensionless and expresses the negative of the logarithm of H + concentration (measured in mol/L or M). For example, the concentration of H + ions in water is 10 −7 M, for a pH of −log(10 −7 ) = 7. Values of pH larger than 7 indicate increased alkalinity and values of pH smaller than 7 indicate increased acidity. Normal values for pH in healthy humans range from 7.35 to 7.45 (note the lack of units).
The pH glass electrode operates on the principle that when two solutions of different hydrogen ion concentrations are separated by a glass membrane, the electrical potential difference between the solutions is proportional to their difference in hydrogen ion concentration. The pH glass electrode consists of two electrochemical cells. A silver/silver chloride measurement electrode is a bulb made of pH-sensitive glass containing a buffer solution titrated to a constant pH value. The outside of the pH-sensitive glass is exposed to the sample (blood) such that an electrical potential difference is created that is proportional between the blood pH and the pH of the buffering solution inside of the bulb. The potential difference is measured using a mercury/mercury chloride (calomel) reference electrode that is placed in buffered potassium chloride solution and is in contact with the blood through a semiporous membrane ( Fig. P7.2 ). In contrast to the Clarke electrode, the pH glass electrode does not require an external voltage source and does not involve an exchange of ions or electrons. As a consequence, neither the electrodes nor the buffering solutions are consumed in the process of measurement.

Both electrodes must be kept at 37°C and calibrated with buffer solutions of known pH. Just as with the Clarke electrode, results are reported with respect to a body temperature of 37°C (98.6°F). If the patient temperature deviates from 37°C, a temperature correction must be applied that has been experimentally determined for whole blood to be
Δ pH Δ T = − 0.015 K − 1 .
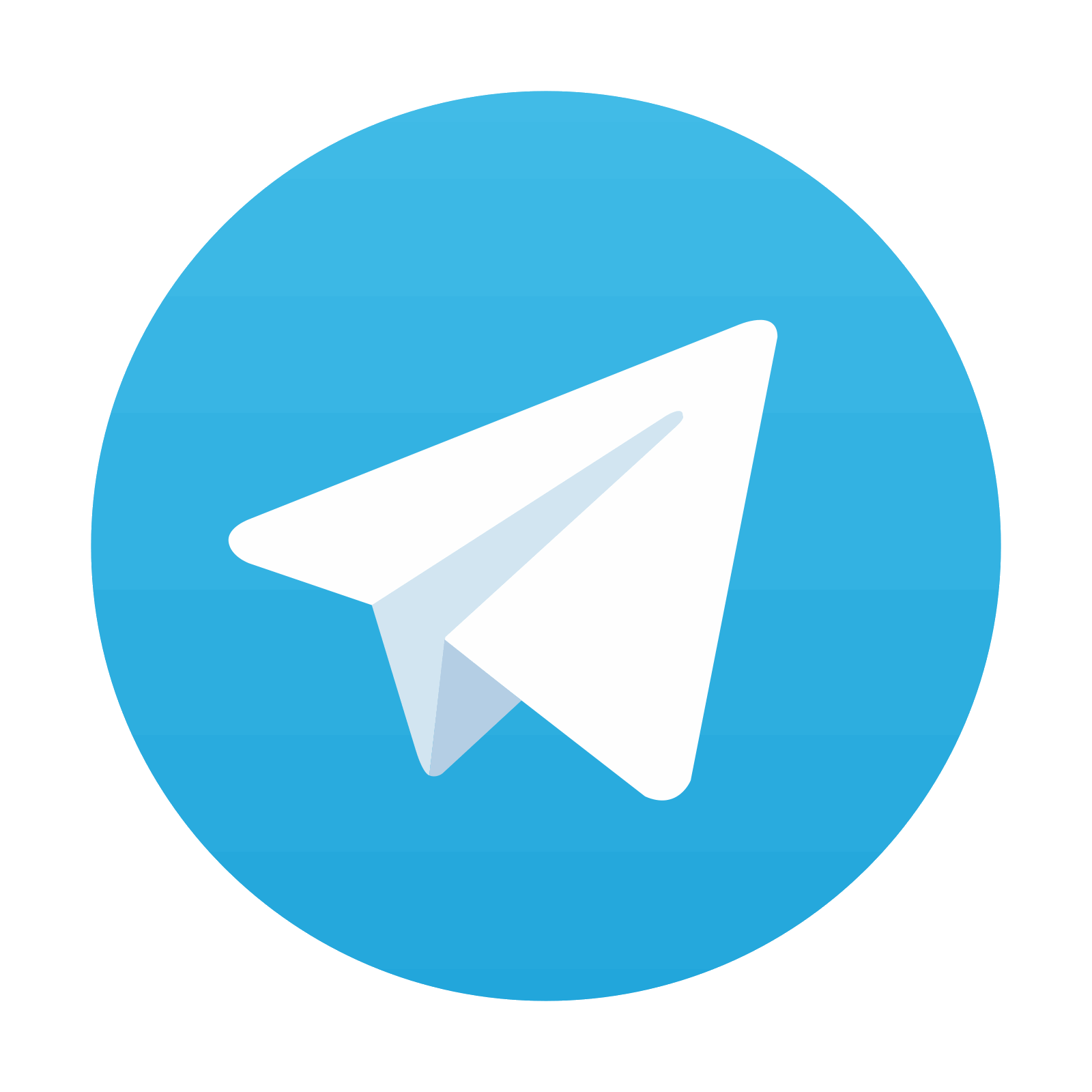
Stay updated, free articles. Join our Telegram channel
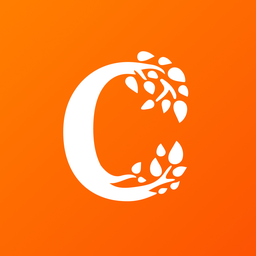
Full access? Get Clinical Tree
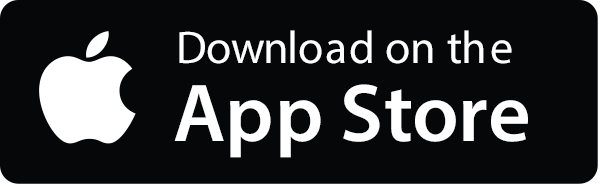
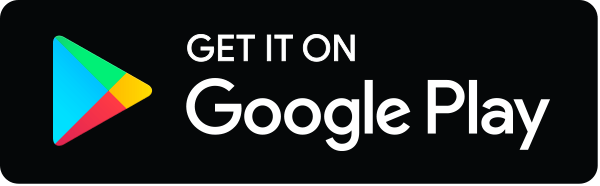