Key Points
- ▪
Inhaled anesthetics affect every part of physiology of the lungs and their pulmonary pharmacology is complex.
- ▪
Volatile anesthetics produce bronchodilation through decreases in cytoplasmic ionized calcium concentration and/or a reduction in calcium sensitivity of airway smooth muscle. Volatile anesthetics also attenuate an increase in pulmonary airway resistance induced by chemical and mechanical stimulation.
- ▪
Inhaled anesthetics reduce the rate of mucous clearance and affect function of type II alveolar cells; these effects can potentially contribute to postoperative pulmonary complications.
- ▪
Volatile anesthetics produce a biphasic response in pulmonary vascular smooth muscle. Although inhibition of hypoxic pulmonary vasoconstriction by volatile anesthetic is an overall small effect, it can contribute to worsening of hypoxemia in patients with underlying pulmonary disease.
- ▪
Volatile anesthetics depress respiratory function through a diminished respiratory drive and an increase in upper airway collapsibility. Following extubation, even at residual concentrations, volatile anesthetics can severely impair peripheral chemoreceptor inputs and hypoxic arousal reflexes.
- ▪
Volatile anesthetics produce dose-dependent reductions in tidal volume and minute ventilation, cause tachypnea, and blunt ventilatory response to hypercapnia and hypoxia.
- ▪
During anesthesia with volatile agents, diaphragmatic function remains relatively well preserved, whereas inspiratory rib cage muscles are significantly depressed resulting in insufficiency of breathing or paradoxical breathing.
- ▪
Volatile anesthetics can compromise upper airway patency. Even at low concentrations, upper airway obstruction can occur in susceptible patients, including elderly, obese, or critically ill patients.
- ▪
Volatile anesthetics vary in their ability to irritate airways and augment defensive airway reflexes. Sevoflurane causes the least amount of subjective airway irritation and is the anesthetic of choice for inhaled induction of anesthesia in infants and children.
- ▪
Preclinical and clinical evidence suggests therapeutic potential of isoflurane and sevoflurane in acute lung injury.
- ▪
Although concerns have been recently raised regarding the use of nitrous oxide, a lack of strong evidence does not justify abandoning it from clinical practice, especially considering its favorable cost-effectiveness.
- ▪
Xenon produces a fast on and off action and is a promising alternative for sedation in the critical care setting.
Acknowledgment
This chapter is a consolidation of two chapters in the eighth edition, Chapter 27 Inhaled Anesthetics: Pulmonary Pharmacology and Chapter 28 Inhaled Anesthetics: Cardiovascular Pharmacology. The editors and publisher would like to thank the following authors: Neil E. Farber, Eckehard A.E. Stuth, Astrid G. Stucke, and Paul S. Pagel for their contributions to the prior edition of this work. It has served as the foundation for the current chapter.
Introduction
Pulmonary pharmacology of inhaled anesthetics is complex. This chapter focuses in depth on pulmonary pharmacology of isoflurane, sevoflurane, desflurane, nitrous oxide, and xenon. The older anesthetics (including halothane, enflurane, and ether) are no longer in use in the developed countries, and are discussed here only for comparison. The lungs are unique in their exposure to a wide variety of physical forces including ventilation, blood flow, and surface tension as well as derangements of function caused by disease and environmental factors. The effects of inhaled anesthetics on ventilatory control, airway tone, mucociliary function, surfactant production, pulmonary vascular resistance (PVR), and acute lung injury (ALI) are examined in detail in this chapter.
The physical properties of commonly used inhalational anesthetics and clinical concerns are presented in Table 21.1 .
Property | Halothane | Isoflurane | Sevoflurane | Desflurane | Nitrous oxide | Xenon |
---|---|---|---|---|---|---|
Boiling point (°C) | 50.2 | 49 | 59 | 24 | −88 | −108 |
Vapor pressure (mm Hg) at 20°C | 241 | 238 | 157 | 669 | 38,770 | – |
Blood:gas partition coefficient | 2.5 | 1.46 | 0.65 | 0.42 | 0.46 | 0.115 |
Oil:gas partition coefficient | 224 | 91 | 47 | 19 | 1.4 | 1.9 |
Minimal alveolar concentration (MAC) | 0.74 | 1.17 | 1.8 | 6.6 | 104 | 63-71 |
Metabolized in the body (%) | 25 | 0.2 | 2-4 | 0.02 | 0 | 0 |
Clinical concerns | Hepatotoxicity | Compound A | Airway irritation | Gas expansion | Apnea |
Inhaled Anesthetics
Asthma and Bronchospasm Overview
Asthma is a chronic airway disease with an estimated worldwide annual death rate of 250,000. Asymptomatic asthma patients have relatively infrequent perioperative respiratory complications; however, bronchospasm develops in about 9% of asthmatics in the perioperative period. Twenty-five percent of asthmatics may present with wheezing after induction of anesthesia, and 1.7% of asthma patients sustain a poor respiratory outcome. In addition, acute bronchospasm can occur at induction, intubation, or during maintenance of anesthesia without prior history of asthma or COPD. According to the ASA Closed Claims Project 40 cases of bronchospasm resulted in settled malpractice claims, with 88% involving brain damage or death. Importantly, only half of the patients had preexisting asthma or COPD. Adverse respiratory events accounted for 28% of claims related to anesthesia-related brain damage and death in the United States; they were associated with the highest mean cost per closed claim. In France, 7% of anesthesia-related deaths were also attributed to bronchospasm. A nonallergic mechanism was involved in nearly 80% of cases. Although bronchospasm caused by airway irritation occurred more frequently in patients who had one or more predisposing factors including asthma, heavy tobacco smoking, or bronchitis, only 50% and 60% of patients with nonallergic and allergic bronchospasm, respectively, had history of asthma.
Physiology of Bronchial Smooth Muscle
Airway resistance is increased, at least in part, by an increase in bronchiolar smooth muscle (BSM) tone. BSM extends down to the terminal bronchioles and is controlled primarily by autonomic nervous system activity. Nonadrenergic, noncholinergic mechanisms, activated by the stimulation of the afferent bronchopulmonary sensory C fibers, play a role in BSM constriction to tachykinins, vasoactive intestinal peptide (VIP), adenosine, and calcitonin gene-related peptides.
Asthmatic bronchiolar constriction involves complex interactions of airway nerves, smooth muscle, epithelium, and inflammatory cells. In contrast, reflex bronchoconstriction caused by airway irritation is mediated by sensory afferents in the nucleus of the solitary tract (NTS), projecting to vagal preganglionic neurons (VPN). Glutamate modulates stimulation of NTS and VPN, whereas γ aminobutyric acid (GABA) is inhibitory from NTS projecting to VPN. VPN projections to the airways release acetylcholine (ACh) predominantly onto M3 receptors, inducing bronchoconstriction. Baseline airway tone is also mediated by the vagus nerve. The muscarinic acetylcholine receptors (mAChRs, M1-M5) on BSM are G protein-coupled receptors, three of which (M1, M2, and M3) are expressed in the lungs of humans and of most mammals. Belmonte and colleagues reviewed the essentials of muscarinic control of mAChRs in the lungs. Neuronal inhibitory M2 muscarinic ACh receptors on parasympathetic nerves are responsible for limiting ACh release from these nerves ( Fig. 21.1 ).

Role of Calcium
Alterations in cytoplasmic ionized calcium concentration (ICa +2 ) and calcium (Ca +2 ) influx result from the interplay of cyclic nucleotides in BSM that alters myosin light chain (MLC) and MLC kinase activity. Ca +2 /calmodulin-dependent MLC kinase is critical for tonic smooth muscle contraction. Agonist activation of BSM also involves a second messenger, cADP ribose, resulting in inositol triphosphate (IP 3 )-mediated release of Ca +2 from sarcoplasmic reticulum via activated ryanodine channels. This release is followed by an increase in sodium (Na + ) influx across the cell membrane. This localized increase in Na + may switch the Na + /Ca 2+ exchanger into reverse mode, leading to even more Ca 2+ influx and greater bronchial constriction. Several different cyclic adenosine monophosphate (cAMP) signaling compartments exist in airway smooth muscle that are responsive to different hormones and neurotransmitters. The mechanical stretch of human BSM cells also causes contraction via an influx of Ca 2+ through unique stretch-activated nonselective cation channels. Adenosine indirectly enhances contraction of airway smooth muscle via the activation of mast cells and via nerves by directly stimulating adenosine type I receptors on airway smooth muscle, thereby rapidly mobilizing ICa 2+ stores through G proteins and IP 3 signaling. Agonist-induced stimulation of particulate guanylyl cyclase relaxes bronchial smooth muscle by attenuating inward Ca 2+ current, whereas stimulation of soluble guanylyl cyclase by substances such as nitric oxide (NO) reduces ICa 2+ concentration and Ca 2+ sensitivity.
Histamine
Release of histamine in the airway produces reflex bronchoconstriction from actions on H1 receptors on BSM, eliciting activation of phospholipase C and protein kinase C (PKC) downstream mediators, leading to release of Ca 2+ from intracellular stores. This leads to calcium ion entry through calcium channels, cation channels of the transient receptor potential (TRP1) channel type, and stimulation of a Na + / Ca 2+ exchanger. Nicotinic acid adenine dinucleotide phosphate has also been suggested as a potential second messenger in histamine-induced Ca 2+ release from lysosome-like acidic compartments, functionally coupled to the endoplasmic reticulum via H1 receptor in endothelial cells. This increase in bronchomotor tone is attenuated by the cholinergic antagonist atropine. The histamine-degrading enzyme, histamine N-methyltransferase, has been localized to human airway epithelium and may play a protective role against histamine-mediated bronchoconstriction. Histamine-induced bronchoconstriction, measured as altered pulmonary resistance (RL) and dynamic pulmonary compliance (C dyn ) in response to intravenous (IV) histamine was studied in dogs to determine the bronchodilatory effects of halothane, sevoflurane, isoflurane, and enflurane. All the volatile anesthetics demonstrated an inhibitory effect on increases in RL and decreases in C dyn caused by histamine.
Adrenergic Receptors
Adrenergic receptors in BSM are classified into α and β 2 types. The α2 agonists clonidine and dexmedetomidine have been shown to produce bronchodilation in central airways, which may be mediated through α2 central vagolytic effects. Clinically, the β 2 -receptor subtypes play an important role in BSM responsiveness. Stimulation of β 2 adrenoceptors causes cAMP-mediated relaxation via activation of protein kinase A and subsequent Ca 2+ efflux out of the cell and into the SR. Interestingly, asthma, as well as allergy- and methacholine-induced bronchospasm, do not appear to be genetically linked to a dominant β 2 -adrenoceptor gene.
Respiratory epithelium releases substances that modulate BSM tone. Removal of the epithelium enhances contractile responses to ACh, histamine, or serotonin in large airways and decreases relaxation responses to isoproterenol in small airways. These responses are similar to the effect of endothelial damage on vascular smooth muscle tone. Notably, cardiopulmonary bypass does significantly affect porcine bronchiolar epithelium-mediated bronchomotor activity, in contrast to vascular endothelium-mediated smooth muscle dysfunction. While endogenous epithelial factors have been identified, endogenous NO produced in respiratory epithelium likely plays a bronchodilatory role similar to that of vascular endothelium. Endothelin-1 is a potent constrictor of vascular smooth muscle and BSM that activates the IP 3 pathway and produces greater pulmonary effects compared to the systemic circulation. Clinically, evaluation of the effects of the inhalational agents may be framed against a background of the major physiologic mechanisms governing normal and pathophysiologic BSM activity. These include airway disease (asthma and COPD), the overarching complex chemistry of calcium release as the BSM stimulus-contraction coupler, intrinsic factors (afferent and efferent ACh signaling, α2 receptor signaling, NO and endothelin-1, allergy and histamine release), and extrinsic factors (physical and chemical irritants, e.g., endotracheal tubes), capable of promoting reflex bronchoconstriction.
Effects of Inhaled Anesthetics
In general, all volatile anesthetics are bronchodilators, making them a good choice for patients with increased airway resistance. Using computed tomography (CT), Brown and colleagues demonstrated that halothane causes dose-dependent bronchodilation in a dog ( Fig. 21.2 ).

Eliminating indirect effects of arterial carbon dioxide (CO 2 ) tension is important when examining the actions of volatile anesthetics on bronchial tone, especially during spontaneous ventilation. This is because hypercapnia-induced bronchodilation and hypocapnia-induced bronchoconstriction are both attenuated by isoflurane. Thus a concentration-dependent bronchodilator effect of volatile anesthetics may be indirectly attributable to increase in CO 2 tension. The structure of the respiratory epithelium changes from pseudostratified columnar cells of the large airways to thinner, cuboidal cells of the bronchioles, and thus a relatively large amount of histologic heterogeneity exists between these regions. The specific effects of volatile anesthetics on the bronchioles depend on the location in and the structure of the respiratory tree. In vitro, isoflurane preferentially relaxes the bronchioles rather than the bronchi. Isoflurane and halothane dilate fourth order bronchi at equivalent minimum alveolar concentration (MAC) values. Similarly, at up to 1 MAC, isoflurane, sevoflurane, and desflurane attenuate methacholine-induced bronchoconstriction in open-chest, pentobarbital-anesthetized rats. Isoflurane and sevoflurane also appear to have greater inhibitory effects on bronchial contraction as compared with tracheal smooth muscle contraction. Furthermore, halothane, desflurane, and isoflurane relax distal airways (e.g., bronchioles) to a greater extent than proximal airways (e.g., bronchi). These differential effects appear to be related to the type of voltage-dependent Ca 2+ (VDC) channels that are present in these regions.
Inhalation of 1 or 2 MAC halothane, enflurane, sevoflurane, and isoflurane did not alter baseline pulmonary resistance and dynamic pulmonary compliance, but these anesthetics significantly attenuated increases in airway resistance and decreases in dynamic respiratory compliance in response to intravenous histamine. Halothane was the most effective bronchodilator, whereas responses to isoflurane, enflurane, and sevoflurane were similar. In an isolated lung model, desflurane produced bronchodilation at 1 MAC but increased airway resistance at 2 MAC, presumably resulting in part from significant increases in inspired gas density at higher MAC. Isoflurane, sevoflurane, and desflurane at 1.0, 1.5, and 2.0 MAC in 25% O 2 were all examined in a two-chamber test lung model with fixed resistance. All anesthetics demonstrated increased density and calculated resistance at higher MAC, with desflurane producing the highest increase in resistance at 2.0 MAC. In a randomized clinical trial evaluating the same agents at 1.0 and 1.5 MAC, total inspiratory resistance (R[rs]), minimal resistance (R[min]), and effective resistance (D[Rrs]) were calculated using the end-inspiratory occlusion technique. No significant differences of those parameters were observed during administration of the three agents at 1 MAC for 30 min. At 1.5 MAC, desflurane caused a maximum increase in R(rs) by 26% and in R(min) by 30% above baseline, in contrast to isoflurane and sevoflurane, which did not have a significant effect on R(rs) and R(min). Presumably, this increased resistance is due at least in part to the greater net viscosity of the higher absolute concentration of desflurane at 1.5 MAC. Other factors may also cause the reduction in the bronchodilation of desflurane at higher concentrations, especially in smokers. Halothane, enflurane, and sevoflurane are equivalent at dilating third- or fourth-generation bronchi as directly measured with a fiberoptic bronchoscope in vivo.
It appears that desflurane can both augment or inhibit bronchoconstriction (see below). At 1 MAC desflurane and sevoflurane blocked, to a similar degree, the increase in central airway resistance after a cholinergic challenge in rabbits. Both anesthetics reduced basal bronchial tone by 30% to 40%. This effect was consistently observed in the presence or absence of allergic airway inflammation and bronchial hyperresponsiveness. When the mechanism of constriction is centrally mediated, such as with a cholinergic challenge, desflurane appears to play a beneficial role in attenuating bronchoconstriction. However, when the mechanism of airway constriction is due to nonadrenergic, noncholinergic activation with tachykinins, desflurane may augment and worsen constriction. Thus clinicians tend to avoid using desflurane in patients with reactive airway disease.
Effects of Inhaled Anesthetics on Bronchomotor Tone in Humans and the Work of Breathing
Sevoflurane (1 MAC) reduced respiratory system resistance by 15% in patients undergoing elective surgery, whereas desflurane had no effect. Rooke and associates compared the bronchodilating effects of halothane, isoflurane, sevoflurane, desflurane, and thiopental–nitrous oxide in healthy patients undergoing induction of anesthesia and tracheal intubation. In contrast to thiopental–nitrous oxide, all volatile agents with the exception of desflurane significantly reduced respiratory resistance ( Fig. 21.3 ).

The work of breathing is defined as pressure or force multiplied by the tidal volume during inspiration. Respiratory work is further divided into elastic work (required to overcome the recoil of the lung) and resistive work (required to overcome airway flow resistance and viscoelastic resistance of pulmonary tissues). The work of breathing is usually derived from transpulmonary pressure volume curves. Volatile anesthetics increase the work of breathing in adults and children. In rats, sevoflurane reduced pulmonary compliance at the lung periphery rather than at the airway level, thereby increasing viscoelastic and elastic pressures in the lung. In addition, in a murine model of chronic asthma, sevoflurane significantly decreased resistance in central and distal airways and also lowered resistance in the lung periphery. These data suggest that sevoflurane exerts a beneficial effect in the presence of chronic airway obstruction and indicate that sevoflurane might reduce the work of breathing in comparison to other drugs ( Fig. 21.4 ).

Studies in humans demonstrate a ceiling effect where low concentrations of volatile anesthetics significantly reduce upper airway resistance , reflecting changes in airway smooth muscle tone in the major airways. In contrast, distal airways and lung parenchyma lack a smooth muscle component (with lower airway and alveolar resistance being more a measure of viscoelastic changes in the lung). Increasing concentrations of inhalational agents have diminished effect on these more distal pulmonary components and thus do not further reduce total lung resistance ( Fig. 21.5 ).

Expiration is passively affected by the recoil characteristics of the lung during normal breathing. In anesthetized patients, the ventilatory response to expiratory resistance is reduced to a greater extent than the response to inspiratory resistance. Conscious and anesthetized humans exhibit decreases in respiratory rate when expiratory resistive loads are applied, but only anesthetized subjects develop rib cage–abdominal wall motion dyssynchrony that causes less effective ventilation and reduction in minute alveolar ventilation. This concept may be particularly important in spontaneously breathing anesthetized patients who demonstrate expiratory obstruction, such as in cases of asthma, COPD, airway secretions, or during hypopharyngeal obstruction or partial breathing circuit occlusion.
Experimental studies demonstrating the equal potency of sevoflurane and isoflurane and the higher potency of halothane for bronchodilation must be extrapolated with caution because histamine-mediated experimental bronchospasm may not closely mimic tracheal intubation–induced bronchospasm in humans. Indeed, Arakawa and colleagues showed that similar inspired concentrations of halothane, isoflurane, and sevoflurane produced nearly identical reductions in airway resistance in a patient with status asthmaticus. Volatile anesthetics may thus be an effective therapeutic modality in status asthmaticus when conventional therapy has failed.
β-Adrenoceptor agonists may be beneficial for treating acute bronchospasm in patients anesthetized with halothane, but their use may not be beneficial with other volatile anesthetics. For example, the β 2 -adrenergic agonist fenoterol reduced respiratory system resistance after endotracheal intubation but did not further decrease resistance when administered in the presence of 1.3% isoflurane. These findings should be interpreted with caution because the technique used to determine respiratory system resistance incorporates alterations in lung and chest wall resistance, as well as tissue viscosity. The most important functional change that occurs in the presence of lung disease is increased resistance. Resistance to airflow is typically thought of as being determined by the flow rate and airway smooth muscle tension. However, nonmuscle elements, such as lung inflammation, airway thickening, altered lung volumes, lung recoil, airway wall remodeling, mucous hypersecretion, and loss of lung elastance, also play a clinically significant role in the amount of airway narrowing. The role of volatile anesthetics in altering many of these non–smooth-muscle elements responsible for airway resistance needs further elucidation.
The effects of volatile anesthetics on bronchomotor tone are also dependent on the substance used to produce contraction in vitro. Relaxation of tracheal smooth muscle by halothane and isoflurane is greatest in the presence of the endogenous mediator serotonin (resulting from anaphylactoid or immunologic reactions) compared with ACh (representing the neutrally derived mediator of reflex bronchospasm). Thus inhaled anesthetics may remain effective bronchodilators, even in the presence of severe serotonin- or histamine-induced bronchospasm that is refractory to β 2 -adrenoceptor therapy. It is important to note that in anesthetized patients, volatile anesthetic–induced decreases in bronchomotor tone and neurally mediated airway reflexes may be partially opposed by a simultaneous reduction in functional residual capacity (FRC). The well-known increased risk for morbidity and mortality in patients with asthma may be at least partially attributed to these FRC-mediated increases in airway resistance. Low temperature abolished the inhibitory effects of volatile anesthetics on carbachol-induced contraction of airway smooth muscle in dogs, suggesting that intraoperative hypothermia may also attenuate volatile anesthetic–induced bronchodilation.
Bronchospasm may occur in conditions and with respiratory diseases other than asthma. For example, healthy patients undergoing surgical stimulation of lung parenchyma or airways including tracheal stimulation by an endotracheal tube are at risk of developing bronchospasm. Preoperative medications, sedatives, neuromuscular blockers, and volatile anesthetics are all important factors that may trigger or attenuate bronchospasms. Regardless of the airway sensitivity prior to induction, the different pathways involved in an individual patient may yield different responses to the volatile anesthetics. Iwasaki and colleagues demonstrated that sevoflurane-induced relaxation of airway smooth muscle and VDC channels were dependent on the type of hyperreactive airway model. Sevoflurane had smaller effects in a model of chronic tobacco smoking (enlarged alveolar ducts and less muscarinic hyperreactivity), compared with an antigen-acute asthmatic (ovalbumin-sensitized) model. The morphologic changes in the peripheral airway may be responsible to some degree for a decrease in the efficacy of volatile anesthetics as bronchodilators in tobacco smokers, but sevoflurane and isoflurane still decrease respiratory system resistance in patients with COPD.
In children undergoing elective imaging studies, sevoflurane produced progressive reductions in the cross-sectional area of upper airway, resulting in pharyngeal airway collapse (also see Chapter 93). As observed with isoflurane in animal models, the effects of sevoflurane were not uniformly distributed along the upper airway. In healthy children, sevoflurane slightly decreased airway resistance, but desflurane had the opposite effect, presumably via reduced airway size. Children with documented airway susceptibility, such as those with asthma or a recent upper respiratory tract infection, exhibit significant increases in airway resistance. In these pediatric patients, desflurane should be avoided.
Mechanisms of Action of Volatile Anesthetics
Volatile anesthetics attenuate airway smooth muscle tone by reducing smooth muscle contractility. This action is the result of the direct effects on bronchial epithelium and airway smooth muscle cells, combined with the indirect inhibition of reflex neural pathways. Several intracellular mediators responsible for Ca 2+ mobilization are potential sites for the action for volatile anesthetics. Inhibition of cell membrane-associated VDC channels reduces Ca 2+ entry into the cytosol. Volatile anesthetic–induced increases in cAMP concentrations cause decreases in intracellular-free Ca 2+ by stimulating Ca 2+ efflux and increasing Ca 2+ uptake into the sarcoplasmic reticular (SR). In addition, a decrease in Ca 2+ sensitivity attributable to inhibition of PKC activity, inhibition of G-protein function, and attenuation of Rho/Rho-kinase signaling pathways also play important roles. Volatile anesthetics may also alter pulmonary resistance by affecting the density of the gas mixture. In an experimental lung model of fixed pulmonary resistance, high concentrations of volatile anesthetics increased the density of the gas mixture and the calculated resistance, with desflurane producing the largest increase ( Fig. 21.6 ). Such an effect is particularly profound for 55 xenon which has a molecular weight of 131.2 Daltons, making it four times as dense as room air.

The effects of volatile anesthetics on proximal, compared with distal, airways may be related to the differential effects on VDC channels and the relative distribution of these channels. Long-lasting (L-type) VDC channels appear to be the predominant mechanism for Ca 2+ entry in tracheal smooth muscle, whereas both transient (T-type) and L-type VDC channels are present in bronchial smooth muscle. Isoflurane and sevoflurane inhibit both types of VDC channels in a dose-dependent fashion, and their effects on T-type VDC channels in bronchial smooth muscle are even more pronounced ( Fig. 21.7 ).

The differential effects of volatile anesthetics on tracheal smooth muscle, compared with bronchial smooth muscle, may also be related to actions on Ca 2+ -activated chloride channel activity or differential sensitivities of K + channel subtypes.
The proposed signaling pathways underlying volatile anesthetic–induced bronchodilation are depicted in Fig. 21.8 .

Volatile anesthetic–induced reductions in ICa 2+ result from the inhibition of both VDC and receptor-gated Ca 2+ channels. In addition, volatile anesthetics deplete Ca 2+ stores in SR by increasing Ca 2+ leakage. Ca 2+ influx occurring in response to depleted SR Ca 2+ stores is known as store-operated Ca 2+ entry (SOCE). By depleting Ca 2+ stores in SR, volatile anesthetics may be expected to enhance SOCE. Nevertheless, at clinically relevant concentrations, volatile anesthetics (isoflurane more than sevoflurane) also inhibit SOCE in airway smooth muscle to further reduce available Ca 2+ .
Volatile anesthetic–induced reductions in SR Ca 2+ appear to occur through enhancement of IP 3 and ryanodine-receptor channel activities. Kai and co-workers demonstrated that halothane attenuates ACh-induced Ca 2+ sensitization in canine tracheal smooth muscle to a greater extent than does sevoflurane, whereas isoflurane has effect at concentrations equivalent to 2 MAC. This effect appears to be mediated, at least in part, by an increase in smooth muscle protein phosphatase, modulation of G proteins (specifically G q and G I that exert actions on cyclic guanosine monophosphate [cGMP]), or modulation of the Rho/Rho-kinase signaling pathways. Volatile anesthetics interact with the muscarinic receptor–heterotrimeric G protein complex to prevent agonist-promoted nucleotide exchange at the Gα subunit of the G protein. Halothane, sevoflurane, and, minimally, isoflurane exert direct effects on muscarinic receptor–mediated contraction of isolated airway smooth muscle. The inhibitory effects of volatile agents on the biochemical coupling between the M 3 muscarinic receptor and the Gα q heterotrimeric G protein is completely reversible with time. Isoflurane-induced relaxation of precontracted bronchial smooth muscles is significantly augmented by pretreatment with a Rho-kinase inhibitor, whereas sevoflurane-inhibited guanosine-5′-triphosphate (GTP) γ S-stimulated contraction and membrane translocation of both Rho and Rho-kinase in a concentration-dependent manner. These latter actions play important roles in Ca 2+ sensitization. The final pathway in airway smooth muscle contraction is the generation of force and shortening of smooth muscles regulated by myosin cross-bridge number and kinetics. Isoflurane modulates both cross-bridge number and cycling rates of isolated rat airway smooth muscle.
The bronchodilatory effect of volatile anesthetics is also mediated by GABA A (GABA A ) receptors in the brainstem or GABA B (GABA B ) receptors on preganglionic cholinergic nerves in the lung similar to the observations with propofol. Indeed, GABA A and GABA B receptors, as well as glutamic decarboxylase (enzyme responsible for GABA synthesis), are located in airway epithelial and smooth muscle cells. GABA levels increase and localize to airway smooth muscle after contractile stimuli in upper airways. Furthermore, a GABA agonist reversed augmentation of cholinergic-induced tracheal ring contraction.
Inhaled but not intravenous halothane attenuated bronchoconstricting effects of low inhaled concentrations of CO 2 in rat distal bronchi, suggesting that halothane exerts a direct action on airway muscle tone or local neural reflex arcs rather than centrally controlled reflex pathways. Consistent with this hypothesis, halothane-, isoflurane-, sevoflurane-, and desflurane-induced dilation of distal bronchial segments is partially dependent on the presence of bronchial epithelium. Prostanoids (e.g., prostaglandin E 2 or I 2 ) or NO may be responsible for the bronchodilatory effects of volatile anesthetics under these conditions. Focal epithelial damage or inflammation may occur in small airways in patients with asthma or allergen exposure, resulting in a reduced bronchodilatory response to volatile anesthetics. Nevertheless, the most pronounced bronchodilatory action of volatile anesthetics in patients with chronic reactive airway disease occurs primarily in the proximal rather than distal airways.
Direct stimulation of intrinsic airway nerves in vitro produces a contractile response that is inhibited by atropine. Volatile anesthetic–induced bronchodilation also occurs by modulation of this airway cholinergic neural transmission mediated through prejunctional and postjunctional mechanisms. The combination of atropine and halothane did not increase airway caliber over that attained with either drug alone, suggesting that halothane dilates airways by blocking vagal tone during unstimulated conditions. Potent tracheal constriction also occurs in response to the endogenously produced endothelin-1. Isoflurane attenuated the endothelin-1–induced airway smooth muscle contraction in rat tracheal rings, suggesting another potential mechanism for airway smooth muscle relaxation.
Mucociliary Function and Surfactant
Normal Mucociliary Function
The upward clearance of mucus from the tracheobronchial tree is responsible for removal of foreign particulate matter, microorganisms, and dead cells and is a primary pulmonary defense mechanism. Although ciliated respiratory epithelium cells are present throughout the respiratory tree, the density of ciliated respiratory epithelium progressively decreases from the trachea to the terminal bronchioles. Cilia are hair-like appendages, consisting of hundreds of proteins organized around a microtubular architecture. They are anchored to the apical cytoplasm by a basal body and extend from the cell surface into the extracellular space. Cilia are classified as either motile or immotile (primary). Motile cilia were thought to be responsible for generating extracellular fluid movement or propelling individual cells, whereas primary cilia were believed to be vestigial. However, primary cilia are important environmental sensors. In human bronchial epithelium, they play a key role in sensing and transducing extracellular mechanochemical signals and are also capable of identifying smooth muscle injury. Indeed, ciliopathies are responsible for a variety of pediatric disorders such as primary ciliary dyskinesia and autosomal recessive polycystic kidney disease. Christopher et al. studied the effects of temperature (15°C-37°C) with varying combinations with fentanyl, dexmedetomidine, and isoflurane on the cilia of mouse tracheal epithelia. There was a linear correlation between cilia motility and temperature. Fentanyl exerted stimulatory effects on cilia, while dexmedetomidine and isoflurane both inhibited cilia function. When added together, fentanyl, dexmedetomidine, and isoflurane were all cilia inhibitory. In contrast, fentanyl plus dexmedetomidine did not significantly alter ciliary function, with results suggesting complex drug-drug and drug-temperature interactions not predicted by simple summation of effects.
Ciliary motion consists of a rapid stroke in a cephalad direction, followed by a slower caudal recovery stroke. Movements of cilia are closely coordinated to move matter efficiently toward the trachea in a wave motion known as metachronism . Each motile cilium is organized in nine peripheral microtubule pairs surrounding a central pair (9+2). During a beat, dynein arms undergo an adenosine triphosphate (ATP)–dependent attachment, retraction, and release cycle with the adjacent doublet, which results in a sliding motion. The basal bodies of the motile cilia are anchored to the microtubules, nexin links, and radial spokes and are further restricted by the ciliary membrane. This anatomic constraint converts the sliding motion into a bend.
The quantity and physical properties of the mucous layer may also promote the coordination of ciliary beats. Mucus is a mixture of water, electrolytes, and macromolecules (e.g., lipids, mucins, enzymes) secreted by goblet cells and mucosal glands. Thicker layers of mucus slow the removal of surface particles from the airway, whereas low-viscosity mucus promotes more rapid ciliary transport. Impaired mucociliary function in the upper airways correlates with low levels of nasal NO, although the clinical significance of this finding is unclear. Direct nervous system control of ciliary coordination has not been demonstrated in vertebrates, but mucociliary clearance is closely related to autonomic nervous system activity, most likely related to changes in physical characteristics of respiratory secretions (also see Chapter 103).
Several factors affect mucociliary function in mechanically ventilated patients contributing to lung atelectasis and hypoxemia. For example, insufficiently humidified inspired gases reduce ciliary movement and desiccate mucus. In dogs, the flow rate of tracheal mucous was maintained within the basal range during a 40-minute exposure to inspired dry air at a temperature higher than 32°C. However, 3 hours of inhalation of dry air caused a complete cessation of the flow of tracheal mucus, which was restored by the subsequent use of inspired gases with 100% relative humidity at 38°C. Several anesthesia-related factors also reduce the rate of mucous movement, including high-inspired oxygen (O 2 ) concentrations, adjuvant medications (e.g., corticosteroids, atropine, β-adrenoreceptor antagonists) that affect bronchial tone, the presence of an endotracheal tube, and positive-pressure ventilation.
Effects of Inhaled Anesthetics on Mucociliary Function
Volatile anesthetics and nitrous oxide diminish the rates of mucous clearance by decreasing ciliary beat frequency, disrupting metachronism, and altering the physical characteristics or quantity of mucus. In contrast to many intravenous anesthetics, halothane, enflurane, isoflurane, and sevoflurane all reduce ciliary movement and beat frequency in vitro. Among the volatile anesthetics, sevoflurane exhibited the weakest cilioinhibitory effects in rat cultured tracheal epithelial cells in vitro ( Fig. 21.9 ).

Gamsu and colleagues measured the rate of tantalum (a powder that adheres to airway mucus) clearance from the lungs of postoperative patients who received general anesthesia for intraabdominal or lower extremity surgeries. Tantalum retention was closely correlated with the retention of mucus and shown for as long as 6 days after intraabdominal surgery. The administration of halothane (1%-2%) and nitrous oxide (60%) rapidly decreased the rate of mucous movement in females undergoing gynecologic surgery. Little or no mucous motion was observed after 90 minutes of halothane–nitrous oxide inhalation. Bronchial mucosal transport velocity was also determined using radiolabeled albumin microspheres distally deposited in the mainstem bronchi using a fiberoptic bronchoscope in healthy patients. In contrast to the findings of the study with halothane, mucous velocity was unchanged during the administration of 1.5 MAC isoflurane.
Impairment of ciliary beat frequency, mucociliary clearance, and bronchial mucous transport contribute to pulmonary complications, including retention of secretions, atelectasis, and lower respiratory tract infection. A reduction in bronchial mucous transport of as little as 3.5 mm/min (normally 10 mm/min) was associated with an increase in pulmonary complications in patients who were mechanically ventilated for 4 days in the intensive care unit (ICU). Thus pulmonary therapy directed at enhancing clearance of secretions from the airways may be beneficial in the immediate postoperative period, regardless of the type of volatile anesthetic chosen.
Compared to nonsmokers, tobacco smokers have significantly slower bronchial mucous transport velocities and are prone to more frequent pulmonary complications when undergoing major abdominal or thoracic surgeries. The specific effects of volatile anesthetics on mucous movement in smokers have not been well studied. However, it is likely that an additive or synergistic negative effect on mucous transport may be present. Impairment of mucociliary function also occurs after lung transplantation. The mechanism for this dysfunction may be related to alterations in the surface properties of mucus and significant impairment of mucociliary transport distal to bronchial transection and re-anastomosis. The effects of volatile anesthetics on mucous transport in lung transplant patients have not been described, but the baseline reductions in mucociliary movement would certainly predispose to postoperative respiratory complications.
Effects of Inhaled Anesthetics on Surfactant
Pulmonary surfactant decreases the work of breathing by reducing surface tension at the fluid-gas interface. Surfactant is a mixture of proteins and phospholipids synthesized by alveolar type II cells. Similar to mucus, surfactant plays a role in removing foreign particles from airways while also enhancing the bactericidal actions of alveolar macrophages. Halothane and isoflurane produced a transient dose-dependent reduction in phosphatidylcholine (a major component of surfactant) synthesis by alveolar cells during a 4-hour exposure. High concentrations of halothane also disrupted the energy metabolism of cultured alveolar cells as indicated by reduced ATP content and enhanced glycolytic metabolism. Halothane and isoflurane potentiated a hydrogen peroxide–induced reduction of phosphatidylcholine content in alveolar type II cells by affecting cell energetics. Halothane decreased the Na + /K + –adenosine triphosphatase (Na + /K + -ATPase), and Na + channel activity in alveolar type II cells, probably related to alterations in ICa 2+ or ATP depletion. A similar decrease in type II alveolar cell Na + /K + -ATPase occurs after the administration of isoflurane. Transepithelial Na + transport helps regulate alveolar fluid balance, and, as a result, impairment of this transport system may contribute to alveolar edema. This observation may be especially relevant in surgical patients because volatile anesthetics decrease alveolar epithelial fluid clearance.
The phospholipid composition of surfactant is critical to its functional integrity. Another crucial component of surfactant is the hydrophobic surfactant-associated protein C synthesized by alveolar type II cells. This protein facilitates the adsorption and spreading of phospholipids to form the surfactant monolayer and enhances the lipid uptake into alveolar type II cells. Exogenous surfactants that contain surfactant-associated protein C are effective at decreasing barotrauma and mortality in vivo. Clinically relevant concentrations of halothane increase surfactant-associated protein C messenger ribonucleic acid (mRNA) in vitro but cause the opposite effect in mechanically ventilated rats. Extrapolation of these findings to patients should be approached with caution, but halothane and mechanical ventilation may play a potentially additive and deleterious role on surfactant production and homeostasis of the alveolar space, particularly in the presence of ALI. In a rat model, sevoflurane produced deterioration in surfactant composition and viscosity with impaired lung mechanics promoting alveolar collapse ; again, caution is warranted in extrapolating these findings to humans. Bilgi and colleagues compared the effects of low-flow and high-flow inhalational anesthesia with nitrous oxide and desflurane on mucociliary activity and pulmonary function tests in humans. The forced vital capacity and forced expiratory volume and mucociliary clearance of saccharin powder were better preserved with low-flow rather than a high-flow technique, suggesting that heated and humidified gases may have a greater impact than the inhaled anesthetics themselves.
Prolonged administration of volatile anesthetics may produce mucous pooling and impair alveolar cell surfactant metabolism. These actions may result in adverse effects on pulmonary function including development of atelectasis and infections. Patients with excessive or abnormal mucous or surfactant production and patients with ALI, chronic bronchitis, asthma, cystic fibrosis, and chronic mechanical ventilation are at the greatest risk. Clinical studies of the effects of volatile anesthetics on mucociliary function, surfactant metabolism, and immunomodulation in patients with compromised pulmonary function are still sparse.
Pulmonary Vascular Resistance
Regulation of Pulmonary Vascular Tone
The pulmonary vascular bed is a low-pressure, high-flow system. Normal pulmonary arterial (PA) pressure is approximately one-fifth of the systemic arterial pressure. Correspondingly, PVR is lower than systemic vascular resistance. The main pulmonary artery and its major branches have a thinner media and less vascular smooth muscle than the aorta and major proximal arterial vessels. Changes in pulmonary vascular smooth muscle tone alter PVR by affecting the slope of pressure-flow relation. Such direct changes may be produced by a rapid rise in cytoplasmic ICa 2+ of the pulmonary vascular smooth muscle cell or by alterations in sympathetic nervous system activity, arterial O 2 and CO 2 tension, acid-base balance, or plasma catecholamine concentrations. Hypercapnia at constant pH (i.e., isohydria) does not alter tone in isolated pulmonary arteries, but normocapnic acidosis relaxes isolated pulmonary arteries by an endothelium-independent mechanism. However, pulmonary endothelial dysfunction potentiates hypercapnia-induced vasoconstriction. Changes in PA pressure and PVR exert important effects on pulmonary gas or fluid exchange. An increase in PVR occurring in conjunction with a corresponding increase in PA pressure promotes interstitial fluid transudation. An acute increase in PVR can be caused by large tidal volumes, high positive end-expiratory pressure, alveolar hypoxia, hypercarbia, acidosis, and critical closing pressure. Hypoxia and acidosis have a synergistic effect increasing PVR. In contrast to acute pulmonary hypertension, the development of chronic pulmonary hypertension involves endothelial dysfunction with persistent vasoconstriction due to imbalance between endogenous vasoconstrictors (including thromboxane A 2 , angiotensin 2, and endothelin 1) and vasodilators (including NO and prostacyclin), smooth muscle proliferation, platelet aggregation, vascular remodeling and thrombosis, and formation of plexiform lesions that irreversibly obliterate pulmonary arterioles. Clinically, the use of positive inotropic medications (e.g., milrinone) or expanded blood volume can passively decrease PVR by increasing cardiac output. Volatile anesthetics may also have indirect effects on PVR through a reduction in lung volumes during spontaneous ventilation. To assess the total effect of the volatile anesthesia, the volume status and mechanical ventilation on the pulmonary pressures, and right heart function, either transthoracic or transesophageal echocardiography should be considered.
Endogenously produced NO plays an important role in the regulation of PVR both in the healthy, normoxic lung and during hypoxia. NO is generated via oxidation of a terminal guanidinium nitrogen on the amino acid L-arginine. The reaction utilizes molecular O 2 and NADPH as substrates, requires the presence of tetrahydrobiopterin, flavoproteins, calmodulin, and thiols as cofactors, and yields NO in addition to the co-product L-citrullin. This oxidation is catalyzed by a single enzyme protein, NO synthase (NOS), which exists in three distinct isoforms. Constitutive calcium-dependent isoforms of the enzyme were originally purified from neuronal tissue (nNOS) and vascular endothelium (eNOS). When stimulated by bacterial endotoxin and proinflammatory cytokines, a third calcium-independent isoform (iNOS) is induced in various cell types including endothelial and vascular smooth muscle cells, macrophages, and fibroblasts. Once expressed, iNOS is independent of calcium, and NO production continues at a maximum rate. All three NOS isoforms are widely distributed in the lung, extensively involved in vascular homeostasis, and intimately linked to the pulmonary O 2 environment.
NO diffuses away from its point of synthesis and interacts with various intracellular molecular sites both within the generating cells and the target cells. The best-characterized target for NO is iron, bound within certain proteins, either as a heme group or as an iron-sulfur complex. The interaction of NO with the heme component of soluble guanylate cyclase stimulates enzymatic conversion of guanosine triphosphate to cGMP. In turn, increased intracellular levels of cGMP cause relaxation of systemic and pulmonary vascular and nonvascular smooth muscles by several mechanisms. Besides lowering free intracellular calcium and attenuating calcium transients, cGMP causes hyperpolarization of muscle cells through activation of potassium channels.
Inhalation of gaseous NO produces selective pulmonary vasodilation in well-ventilated lung areas and may be beneficial in the treatment of neonatal pulmonary hypertension resulting from a variety of congenital heart diseases, hypoplastic lung, and meconium aspiration. Inhaled NO has also some benefits in the treatment of acute pulmonary hypertension in adults, provided that the PVR is not fixed by remodeling and hypertrophy of pulmonary vasculature. Additionally, the use of inhaled NO to reduce PVR in pediatric and adult patients during cardiac surgeries has become common (also see Chapters 67, 94, and 104).
Prostacyclin is another endogenous vasodilator produced by the endothelium that causes smooth muscle relaxation through adenylyl cyclase stimulation and production of cAMP. Prostacyclin is used in inhaled or injected formulations to produce pulmonary vasodilation in patients with chronic PA hypertension. Another group of pulmonary vasodilators, including sildenafil and tadalafil, acts by inhibiting cGMP-specific phosphodiesterase type 5 (an enzyme that is responsible for cGMP degradation) and is used for therapy of refractory PA hypertension. Finally, riociguat, a novel drug that directly stimulates soluble guanylate cyclase independently of NO, has been recently approved for treating patients with chronic pulmonary hypertension and chronic thromboembolic pulmonary hypertension.
Mechanisms of Hypoxic Pulmonary Vasoconstriction
Changes in PVR affect the regional distribution of blood flow within the lung and produce changes in ventilation-perfusion matching and gas exchange. The distribution of PA blood flow and ventilation in the lung is mainly a gravity-dependent phenomenon that is primarily mediated by the asymmetric branching of airways and blood vessels, thereby establishing regional heterogeneity. An increase in PVR occurring within an area of atelectasis optimizes gas exchange by shifting blood flow away from the atelectatic segment to well-ventilated regions of the lung. This phenomenon, termed hypoxic pulmonary vasoconstriction (HPV), is unique to the pulmonary circulation because other vascular beds (e.g., coronary and cerebral) dilate in response to hypoxia. Thus HPV maintains oxygenation, while medications (including volatile anesthetics) that interfere with HPV may adversely affect gas exchange. HPV plays an especially important role in the presence of atelectasis, pneumonia, reactive airway disease, acute respiratory distress syndrome (ARDS), or one-lung ventilation (OLV). It usually does not contribute to pulmonary blood flow heterogeneity under normal conditions in supine humans.
HPV is a locally mediated phenomenon that occurs when alveolar O 2 tension falls below approximately 60 mm Hg and is maximal when O 2 tension is approximately 30 mm Hg. HPV was first identified in 1894, and the precise mechanism is only now becoming clear. Specialized O 2 -sensing cells modulate respiratory and circulatory function to maintain a normal O 2 supply. Hypercapnia-induced acidosis increases PVR in intact animals and in isolated, perfused lungs. Acidosis-induced increases in PVR are relatively small at normal alveolar O 2 tensions but are dramatically enhanced during alveolar hypoxia. Local acidosis and increases in alveolar CO 2 tension augment HPV and further improve arterial oxygenation in healthy lungs. High CO 2 concentrations reduce NO levels, but whether this action plays a part in hypercarbia-induced improvements in the ventilation-perfusion ratio is unclear.
Although the hypoxia-mediated endothelial-derived vasoconstrictor has yet to be identified, hypoxia releases Ca 2+ from smooth muscle SR via ryanodine receptors, enhances Ca 2+ sensitization, and modulates voltage-gated K + channels in smooth muscles. Other mediators of the hypoxic-response coupling have been identified. Wang and colleagues have recently demonstrated that both connexin 40-mediated retrograde endothelial signal conduction for O 2 sensing, as well as Ca 2+ influx at TRP1 channel V4 on pulmonary arterioles are required for HPV. Ultimately, there are a number of contenders for the role of HPV oxygen sensor, yet there is still no consensus on how this happens in vivo, with most of the proposed mechanisms interlinked, depending on the phase of HPV, the degree of hypoxia, involvement of anesthetic or other agents.
Inhaled Anesthetics and Hypoxic Pulmonary Vasoconstriction
All volatile anesthetics dilate the pulmonary vascular bed. In a thorough review, Akata discussed the mechanisms by which inhaled anesthetics produce this vasodilation, including a reduction in free cytosolic Ca 2+ and inhibition of myofilament Ca 2+ sensitivity. However, the vasodilator effect of volatile anesthetics is modest in normal lungs, and the small attenuation of PVR is usually offset by a concomitant reduction in cardiac output. The net effect of these hemodynamic changes results in minor, if any, changes in PA pressure concomitant with a small decrease in total pulmonary blood flow. In contrast to their action as direct pulmonary vasodilators, volatile anesthetics also attenuate K ATP channel-mediated and endothelium-dependent pulmonary vasodilation in chronically instrumented dogs. This inhibition of pulmonary vasodilation is not a uniform observation under all experimental conditions. For example, isoflurane and halothane, but not enflurane, enhance isoproterenol-mediated vasodilation. Unlike the findings with other volatile anesthetics, K ATP channel–mediated pulmonary vasodilation in response to a K + channel agonist (lemakalim) is preserved during sevoflurane anesthesia. Indeed, evidence suggests that halothane, enflurane, and isoflurane, but not sevoflurane, differentially modulate pulmonary vascular tension through Ca 2+ -activated or voltage-sensitive K + channels, at least in isolated rabbit lungs. Halothane- and enflurane-induced constriction of pulmonary vessels was potentiated by voltage-gated potassium (Kv) channel inhibition in this isolated rabbit lung preparation. In contrast, isoflurane did not affect pulmonary vessels when Kv channels were inhibited. Further, sevoflurane dilated pulmonary vessels and this dilation was unaffected by K + channel subtype inhibitors ( Fig. 21.10 ).

Smooth muscle TASK-1 channels also appear to contribute to volatile anesthetic–induced PA dilation. Rather than causing immediate vasodilation, volatile anesthetics produce a paradoxical initial dose-dependent increase in force in isolated PA strips as a result of Ca 2+ release from intracellular stores ( Fig. 21.11 ). Subsequently, a decrease in force, associated with a Ca 2+ /calmodulin–dependent protein kinase II activation, occurs. Extrapolation of these results to humans must be approached with caution, but these studies suggest that vasodilatory responses of volatile anesthetics may be more profound in patients with reduced SR Ca 2+ stores (e.g., neonates) or those with depressed protein kinase activity (e.g., primary pulmonary hypertension).

In general, all volatile anesthetics attenuate HPV in isolated perfused lungs or in an in situ preparation with constant perfusion ( Fig. 21.12 ), whereas most intravenous anesthetics do not have such an effect. The combined administration of a Ca 2+ -channel blocker and a volatile anesthetic further reduces HPV by up to 40%, compared with either drug alone, suggesting that these drugs inhibit HPV through differing mechanisms. The mechanisms of HPV inhibition by volatile anesthetics are unclear but may be related to arachidonic acid metabolites or endothelial-derived vasodilating factors. However, other evidence suggests that anesthetic-induced inhibition of HPV may occur independent of pulmonary vascular endothelium, NO, or guanylate cyclase. Volatile anesthetics also disrupt Ca 2+ homeostasis in vascular smooth muscle and thereby interfere with pulmonary vasoconstriction. Halothane and isoflurane attenuated endothelium-dependent vasodilation by inhibiting cGMP accumulation and a K ATP channel-mediated interaction between NO and prostacyclin in isolated canine PA rings. In contrast, isoflurane modulated the HPV response, at least in part, through Ca 2+ -activated and voltage-sensitive K + channels. Attenuation of HPV by sevoflurane occurred independent of K + -channel function.

The relative efficacy of volatile anesthetics on HPV in vivo is difficult to assess because several other factors impair HPV, including temperature, pH, CO 2 tension, degree of hypoxia, size of the hypoxic area, surgical trauma, and medications. During OLV, the direct inhibitory effects of volatile anesthetics on HPV may increase perfusion in the nonventilated lung and worsen hypoxemia. However, volatile anesthetics may also affect HPV, lung perfusion, and oxygenation by indirect actions on cardiac output and mixed venous O 2 saturation. Baseline PA blood flow and pressure also modulate the effects of HPV. Elevated PA pressures may cause passive distension of constricted vascular beds and thereby reverse HPV. Alternatively, reflex pulmonary and systemic vasoconstriction in response to hypotension may increase PVR in healthy lung segments, leading to a shift of pulmonary blood flow to hypoxic areas of lung.
Early studies suggested that nitrous oxide attenuates HPV in animal models in vivo. As opposed to the findings with isoflurane, sevoflurane or desflurane did not inhibit HPV in chronically instrumented dogs subjected to gradual occlusion of the right main PA ( Fig. 21.13 ). Nitrous oxide, desflurane, and isoflurane, but not xenon, reduced the mixed venous O 2 saturation, cardiac output, and arterial oxygenation during OLV in pigs. However, nitrous oxide, xenon, desflurane, and isoflurane did not alter perfusion of the nonventilated lung or reduce the shunt fraction during OLV. In animals with a preexisting impairment of gas exchange attributable to a pneumoperitoneum, sevoflurane, but not isoflurane, caused more profound abnormalities in gas exchange than did propofol. Thus although reductions in HPV by volatile anesthetics have been relatively small in vivo, preexisting pulmonary disease may worsen anesthetic-induced gas exchange abnormalities.

Effects of Volatile Anesthetics on Pulmonary Vasculature in Humans
General anesthesia often impairs pulmonary gas exchange. In addition to specific effects of volatile anesthetics, many other factors including gravity, posture, atelectasis, differences in vessel conductance between lung regions, intrathoracic pressure, and HPV may all affect the distribution of pulmonary blood flow and ventilation during the administration of volatile anesthetics. Alterations in regional ventilation are related to variations in alveolar compliance, inspiratory rate, flow rate, pleural pressures, and ventilatory modes. In spontaneously breathing healthy volunteers, sevoflurane (1 MAC for 20 minutes) administered via a facemask did not alter the relative distribution of either ventilation or perfusion in a ventral to dorsal direction measured by single photon–emission CT. Similarly, no change in the distribution of ventilation, as measured by using electrical impedance tomography, occurred in spontaneously breathing adults receiving 0.7 MAC of sevoflurane via a laryngeal mask airway (LMA). Interestingly, sevoflurane reduced regional heterogeneity of the perfusion distribution and increased regional heterogeneity of the ventilation/perfusion ( <SPAN role=presentation tabIndex=0 id=MathJax-Element-1-Frame class=MathJax style="POSITION: relative" data-mathml='V˙/Q˙’>V˙/Q˙V˙/Q˙
V ˙ / Q ˙
) ratio with a tendency toward lower ratios in spontaneously breathing volunteers. This alteration may contribute to less effective pulmonary gas exchange, but these effects were relatively small compared with alterations in <SPAN role=presentation tabIndex=0 id=MathJax-Element-2-Frame class=MathJax style="POSITION: relative" data-mathml='V˙/Q˙’>V˙/Q˙V˙/Q˙
V ˙ / Q ˙
distribution that occur during mechanical ventilation. Either pressure-controlled or pressure-supported ventilation with sevoflurane caused similar ventral redistributions of ventilation.
Isoflurane produces no clinically significant effects on pulmonary shunt in healthy patients even at concentrations that cause systemic hypotension. Thoracic surgeries are usually performed in the lateral position with an open chest and dramatically affect the relative distribution of ventilation and perfusion. Under these conditions, a diseased, nondependent lung may affect the pulmonary vascular response to hypoxia, as may surgical manipulation of the lung itself. Most studies in animal models or patients undergoing OLV did not demonstrate clinically significant attenuation of HPV by volatile anesthetics. Differences in shunt fraction, PVR, or oxygenation between isoflurane and sevoflurane anesthesia in patients undergoing OLV for lobectomy for lung cancer do not appear to be significant. Some studies also demonstrated that shunt fraction is similar in patients receiving propofol or either isoflurane or sevoflurane during OLV. No significant differences in shunt fraction or arterial O 2 tension were observed during intravenous infusions of ketamine, which does not inhibit HPV, and inhalation of enflurane. In contrast, isoflurane and sevoflurane impaired oxygenation and increased shunt fraction more than an intravenous infusion of propofol during OLV. However, the differences in oxygenation observed in these studies were small and clinically insignificant. The relative depth of anesthesia may affect interpretation of the differences in oxygenation between intravenous and volatile anesthetics in these studies. When doses of propofol and sevoflurane were chosen based on a similar depth of anesthesia, assessed by a bispectral index monitor, similar reductions in arterial oxygenation were observed in patients undergoing OLV. Similar changes in shunt fraction and oxygenation were shown to occur with halothane, isoflurane, desflurane, and sevoflurane in patients undergoing thoracotomy and OLV ( Fig. 21.14 ).

Convincing evidence suggests that all volatile anesthetics may be safely used in patients undergoing thoracotomy and OLV. The increase in shunt and the decrease in oxygenation caused by halothane or isoflurane were consistent with an approximately 20% inhibition of HPV at 1 MAC. Instead of the expected 50% reduction in pulmonary blood flow in a hypoxic lung in the absence of a volatile anesthetic, blood flow decreases by 40% during hypoxia in the presence of 1 MAC isoflurane. This change in flow corresponds to an increase in pulmonary shunt by approximately 4% of the cardiac output. Carlsson and colleagues applied multiple inert gas elimination techniques to measure the true shunt fraction in humans anesthetized with volatile anesthetics and demonstrated a 2% to 3% increase in shunt fraction corresponding to approximately 20% HPV inhibition at 1.5% isoflurane. In addition, no significant effects on arterial oxygenation occurred with clinically relevant concentrations of isoflurane or enflurane. Indeed, the use of total intravenous anesthesia with propofol and alfentanil did not reduce the occurrence of hypoxemia during OLV, compared with a volatile anesthetic.
Functionally, volatile anesthetics exert only mild, if any, inhibitory effects on HPV and oxygenation. The relatively small inhibition of HPV should not significantly influence clinical decision making, especially considering the efficacy of drugs such as the peripheral chemoreceptor agonist, almitrine (which enhances HPV), or inhaled NO (which selectively increases perfusion to adequately ventilated pulmonary regions). In addition, ventilatory strategies (e.g., nondependent lung continuous positive airway pressure, permissive hypercapnia) and fiberoptic bronchoscopy to ensure proper positioning of a double-lumen endotracheal tube help alleviate hypoxemia. The net effect of volatile anesthetics on HPV is multifactorial and depends on the direct effects of these agents not only on pulmonary vasomotor tone, but also by indirect actions that occur during anesthesia and surgery.
Control of Respiration
Components of the Respiratory Regulatory System
The regulation of respiratory function is very complex, occurs both on unconscious and conscious levels, and is precisely controlled by neuronal circuits, which are mainly located in the brainstem and include the medulla oblongata, pons, and midbrain ( Fig. 21.15 ).

The neuronal networks in these areas are sufficient to generate involuntary, automatic breathing, which may be superseded by cortical centers for voluntary efforts such as speech, swallowing, laughing, sneezing, and coughing. Respiration per se is to ensure adequate gas exchange in order to meet metabolic demand during various levels of activity. Reflex inputs from the upper airways, lungs, and carotid bodies modulate respiration when inadequacy of gas exchange is sensed. Connections to the lateral hypothalamus also convey wakefulness drive to the respiratory center. Physiological status like sleep and pregnancy alter the respiratory function; so does nonphysiological status such as sedation. This chapter is not intended to discuss respiratory physiology in detail. Rather, its focus is on the major regulatory components of the respiratory system and how they are affected by inhalational anesthetics ( Fig. 21.16 ).

Readers are referred to Chapter 13, Chapter 41 for a detailed discussion of respiratory physiology and monitoring.
In general, respiratory drive is suppressed by all inhalational anesthetics except nitrous oxide. Volatile anesthetics decrease glutamatergic excitatory drive to bulbospinal respiratory neurons and increase GABA A ergic inhibitory mechanisms at the postsynaptic neuronal membrane. In hypoglossal upper airway motor neurons, volatile anesthetics hyperpolarize neuronal membrane resting potential by activating K + channels that are linked to serotonin or norepinephrine receptors. The magnitude of respiratory depression by volatile anesthetics may also depend on the position of the neuron in the neuronal hierarchy, which extends from the neurons generating excitatory respiratory drive to the respiratory pattern generator and down to the phrenic and hypoglossal respiratory output motor neurons (see Fig. 21.16 ). Consequently, volatile anesthetics, which strongly affect synaptic neurotransmission, have a greater depressant effect on polysynaptic neuronal circuits that include many synapses, compared with those comprised of only few neurons (paucisynaptic). Fortunately, most connections between the single components of the respiratory system seem to be paucisynaptic, which may explain a relative resistance of automatic breathing to the depressant effects of volatile anesthetics.
The depressant effect of inhalational anesthetics on respiratory system may be further demonstrated at the molecular and genetic levels. Heterozygous paired-like homeobox 2b (PHOX2B) gene mutations have been found in the majority of patients diagnosed with late-onset central hypoventilation syndrome and may increase sensitivity of those patients to anesthetic-induced depression of breathing. A recent study demonstrated that isoflurane can potentiate aggregation and mislocalization of PHOX2B variants, alter protein folding, and induce endoplasmic reticulum stress, indicating a mechanism by which these agents may affect respiratory neuronal function after surgery and promote the onset of the neurologic respiratory disease.
Importantly, most data on respiratory regulatory systems are derived from animal models and may not directly translate to humans, as summarized in a review by Forster and Smith. This section will therefore cover important animal study results to help understand the basic respiratory structure and function, as well as present recent clinical studies demonstrating the correlation of those findings to clinical practice.
Central Chemoreception
For many years, the prevailing view was that CO 2 /H + sensitivity was due exclusively to the central chemoreceptors. Recent studies showed that central chemoreceptors contribute about two thirds of the ventilatory response to CO 2 /H + , while peripheral chemoreceptors contribute about one third. These excitatory chemodrive mechanisms ensure automatic breathing under normoxic, normocapnic conditions in healthy individuals. Central chemoreceptors discharge in response to the decrease in extracellular pH or an increase in [H + ]. In addition, they project to other areas of the respiratory system where they form excitatory synapses. This pathway is well recognized, but its exact location remains to be defined. Animal studies have pointed to multiple chemosensitive sites in the brainstem including the retrotrapezoid nucleus (RTN), medullary raphé, locus coeruleus, nucleus tractus solitarius (NTS), lateral hypothalamus, and caudal ventrolateral medulla. The RTN is a particularly strong candidate as the absence of RTN likely causes severe central apneas in congenital central hypoventilation syndrome. Glial cells in these areas may also theoretically contribute to chemoreception through their effect on extracellular pH or by hypoxia- or CO 2 -induced ATP release potentially altering the function of chemosensitive neurons.
Central chemoreceptors also promote excitatory drive to neurons regulating upper airway patency and arousal from sleep. The genioglossus muscle, considered a typical representative for the muscles maintaining upper airway patency, receives significant tonic and phasic excitatory drive in the conscious state. Genioglossal muscle activity is reduced during sleep, but high levels of inspiratory CO 2 (>5%) are capable of recruiting near-normal levels of phasic activity. This phenomenon does not occur during rapid eye movement (REM) sleep because genioglossal muscle activity is absent, and therefore the upper airway collapses and hypoventilation follows.
Hypercapnia secondary to upper airway obstruction and hypoventilation may recruit the normal phasic activity of genioglossal muscle through central chemoreceptors and function as another mechanism (aside from hypoxia) by which patients with obstructive sleep apnea (OSA) arouse from sleep and reestablish airway patency. Residual subanesthetic concentrations of volatile anesthetics, which may be present for hours in postoperative patients, inhibit both peripheral hypoxic and CO 2 chemosensitivity and may also substantially impair the spontaneous arousal and resolution of upper airway obstruction that would otherwise occur with severe hypoxia or hypercarbia. This is a particularly important issue to recognize for same-day surgery patients, especially with a history of OSA.
Central Pattern Generator
The cyclic breathing pattern is controlled by the central pattern generator (CPG). A recent review by Abdala and colleagues nicely summarizes the progress in the field. Even though several models of the CPG network have been proposed, many of the circuit interactions and their functionalities remain unknown. CPG is believed to be located in nuclei of the pontomedullary network (see Fig. 21.15 ). In the related nuclei, the network consists of excitatory and inhibitory neurons that control the inspiratory and expiratory phase of breathing. The breathing cycle is complexly regulated, and the type of neurons and the related neurotransmitters have been well illustrated ( Fig. 21.17 ). This neuronal circuit can be pharmacologically modulated by inhaled anesthetics, affecting respiratory rate, upper airway patency, and relative contributions of the chest wall versus the diaphragm to tidal volume and minute alveolar ventilation.

Integration of Peripheral Inputs
Afferent inputs from the periphery reach the brainstem respiratory center and influence respiratory drive. These inputs include the carotid body chemoreceptors, vagal inputs from the lungs and airways, and pulmonary baroreceptor inputs. The carotid body is the major peripheral chemoreceptor. It senses hypoxia or hypercapnia, which in turn increases carotid sinus nerve discharge. This information then reaches the RPG via NTS glutamatergic neurons, which also targets rostral ventrolateral medulla presympathetic neurons thereby raising sympathetic nerve activity (SNA). Chemoreceptors also regulate presympathetic neurons and cardio VPN indirectly via inputs from the RPG. In essential hypertension, OSA, and congestive heart failure, chronically elevated carotid body afferent activity contributes to increasing SNA but breathing remains unchanged or becomes periodic.
The function of the carotid body was discovered nearly 80 years ago, and for a long time it was thought that the carotid body chemoreceptors function independently from central chemoreceptors. However, more recent studies suggested that the peripheral and central chemoreceptors are not functionally separate but are interdependent. The sensitivity of the medullary chemoreceptors is critically determined by input from the peripheral chemoreceptors and possibly other breathing-related reflex afferents as well. This effect might be mediated through interactions within the NTS and/or through NTS projections to parafacial respiratory group/RTN integrating neurons and/or through projections to neurons of a raphe-pontomedullary respiratory network.
Afferent vagal inputs from the lungs and airways are also relayed through the NTS. Pulmonary baroreceptor inputs project to second-order pump neurons in the NTS, which can provide both excitatory and inhibitory inputs to various parts of the medullary respiratory column. In general, pulmonary baroreceptor inputs promote the phase switch from inspiration to expiration. This vagal expiratory-facilitating reflex (Hering-Breuer reflex) is most prominent in immature mammals but also modulates respiratory phase-timing during resting ventilation in adult humans. Although some of these inputs directly influence the CPG, others are integrated at the level of the bulbospinal or premotor neurons, which project to the motor neurons in the spinal cord.
Respiratory Motor Output and Upper Airway Patency
The pontomedullary respiratory network generates the respiratory pattern and then projects to and controls respiratory motor outputs in the brainstem and spinal cord. The phrenic motor neurons are the main inspiratory neurons in the spinal cord. They are located at the levels C 3 to C 5 of the spinal cord and innervate the diaphragm. Phrenic motor neurons are also directly depressed by volatile anesthetics. Expiratory motor neurons are located at approximately T 7 -T 12 of the spinal cord and innervate truncal abdominal muscles that aid with forceful expiration and with expiratory expulsive efforts such as coughing. Expiratory motor neurons receive inputs from expiratory bulbospinal neurons and the pons. The spinal motor neurons are the final neurons in the respiratory neuronal hierarchy. Their activity tends to be reduced by the cumulative effect of anesthetics on all previous steps of chemoreception and neurotransmission.
To ensure effective ventilation, inspiratory muscle activity needs to be closely coordinated with upper airway muscles that maintain airway patency. Central respiratory motor output almost simultaneously engages both the phrenic motor neurons (serving chest wall pump muscles) and hypoglossal motor neurons (serving pharyngeal muscle dilators). The hypoglossal motor nerve innervates upper airway muscles, particularly the genioglossal muscle. The strength of excitatory and inhibitory drive to hypoglossal motor neurons strongly depends on the level of consciousness and differs between the REM and non-REM sleep phases.
The onset of the sleeping state results in a reduced tonic activation of upper airway dilator musculature and enhances collapsibility. This effect is especially problematic for patients with OSA whose airway is narrower, longer, and more collapsible than that of those without OSA. Patients with OSA critically rely on compensatory activation of airway dilator muscles to maintain patency during wakefulness. The reduced lung volumes in obese recumbent patients during sleep also decrease caudal traction on the trachea, promoting pharyngeal collapse. In addition, the loss of wakefulness removes an important vigilance component in the ventilatory control system, leaving the regulation of central respiratory motor output largely under chemoreceptor and mechanoreceptor feedback control.
The mechanisms of airway collapse during sleep and anesthesia are closely related. Hypoventilation and/or hypoxia secondary to upper airway collapse during anesthesia/sedation represent a significant challenge for anesthesia care providers, particularly in ambulatory surgery patients. The effect of inhalational anesthetics on pharyngeal airway collapsibility can best be illustrated by visualizing the changes as the depth of sedation increases.
Effects of Inhaled Anesthetics on Resting Ventilation
Volatile anesthetics suppress conscious control of breathing at concentrations of less than 1 MAC and completely abolish conscious breathing drive at higher concentrations. Breathing is then largely controlled by automatic brainstem mechanisms and chemoreflex inputs. All volatile anesthetics also cause a dose-dependent decrease in minute ventilation at concentrations greater than 1 MAC because of a decrease in tidal volume. However, in a pressure chamber, Hornbein and colleagues demonstrated that nitrous oxide does not significantly reduce minute ventilation within 1.5 MAC (well beyond an atmosphere). The respiratory rate typically increases for all inhalational agents tested, except for xenon, which causes a significant reduction in respiratory rate ( Fig. 21.18 ). Indeed, there are several reports indicating that xenon can cause hypopnea or apnea.

Most volatile anesthetics cause an increase in respiratory rate by producing a decrease in both inspiratory and expiratory duration, whereas opioids cause a very prominent decrease in respiratory rate that primarily results from a large increase in expiratory duration. However, the observed modest decrease in minute ventilation with volatile anesthetics could underestimate the magnitude of the respiratory depressant effects of these agents. This is due to volatile anesthetic–induced hypoventilation increasing PaCO 2 in a closed central chemoreflex feedback loop. In turn, central chemoreceptors are stimulated, thereby increasing central chemodrive to the respiratory center and increasing minute ventilation.
Effects of Inhaled Anesthetics on Chemoreflexes
Volatile anesthetics impair peripheral chemoreceptor responses to hypoxia and hypercarbia in a dose dependent manner. In the presence of volatile anesthetic concentrations of 1 MAC or higher, breathing in humans is entirely dependent on the automatic control from the pontomedullary respiratory center and afferent excitatory inputs from the central chemoreceptors. These anesthetic concentrations lead to a complete depression of the peripheral chemoreflex loop with further respiratory depression rather than stimulation in response to hypoxia. Even very low concentrations of the agent (0.1 MAC of isoflurane and sevoflurane) depress the peripheral chemoreflex loop, without affecting the central chemoreflex loop. Desflurane at the same MAC showed no effect on peripheral and central CO 2 sensitivity. A loss of upper airway muscle tone and function accompanies this process, as does differential depression of neurotransmission at the level of the spinal cord.
The effect of inhaled anesthetics on respiratory drive may not be clinically relevant in the patient undergoing controlled ventilation. However, it does matter in spontaneously breathing patients. Because the suppressive effect of inhaled anesthetics on respiratory drive is synergetic with narcotics and frequently these two drug classes are administered concurrently, the suppression on the hypoxic and hypercarbic drive can increase the risk of postoperative respiratory complications.
Effects of Inhaled Anesthetics on the Hypercapnic Ventilatory Response
In awake humans, about one third of the CO 2 -mediated respiratory drive is through peripheral chemoreceptors and other two thirds through the central chemoreceptors. The respiratory drive mediated by the peripheral chemoreceptors is augmented by hypoxia. However, the hypoxia-mediated component is abolished by the majority of volatile anesthetics at concentration even under 1 MAC. When the anesthetic concentration increases above 1 MAC, the peripheral chemoreceptor-mediated portion of the CO 2 responsive respiratory drive are abolished, and only the central chemoreceptor loop remains functional. Depression of the respiratory drive is often described in a quantitative manner by plotting the CO 2 response curve (an increase in PaCO 2 vs. an increase in minute alveolar ventilation). Volatile anesthetics also cause a rightward shift of the apneic threshold, that is the minimum PaCO 2 required to initiate spontaneous respiration. Thus spontaneous breathing efforts do not occur if mechanical or assisted ventilation efforts drive the PaCO 2 levels below the CO 2 threshold during anesthesia.
Patients under general anesthesia with volatile anesthetics become hypercarbic if they are spontaneously breathing without any ventilatory support. Pressure support ventilation is often used to counteract the suppression of the respiratory drive by the volatile anesthetics. However, it remains to be determined if an increase in pressure support would lead to a proportional increase in minute alveolar ventilation as the intrinsic CO 2 response curve at a given level of sedation is not altered. An increase in minute alveolar ventilation secondary to increased tidal volumes from pressure support may result in a decreased respiratory rate and therefore minute alveolar ventilation may not be increased as much as expected.
Effects of Volatile Anesthetic on the Hypoxic Ventilatory Response In Humans
Hypoxic ventilatory response (HVR) is rarely encountered in conscious healthy humans at sea level. However, for special occupations, such as tunnel workers at sea level, or mountain climbers staying at high attitude, hypoxia does occur and therefore the HVR becomes an important mechanism to compensate for low inspired O 2 concentrations. One example is humans living at high altitude. At the top of Mt. Everest (8848 m), the partial pressure of O 2 of ambient air is only about 50 mm Hg compared with 159.6 mm Hg at sea level. The corresponding arterial blood PaO 2 is only 37.6 mm Hg, which is lower than the partial pressure of O 2 in the mixed venous blood in healthy resting adults living at sea level. The estimated minute ventilation is approximately 166 L/min as a result of severe hypoxic hyperventilation. However, this powerful compensation mechanism is significantly comprised under anesthesia with volatile anesthetics under 1 MAC and completely abolished at 1.1 MAC of halothane ( Fig. 21.19 ). It is important to realize that even a subanesthetic concentration (0.1 MAC) of inhalational anesthetics profoundly diminishes hypoxic respiratory drive without concurrent use of narcotics. The potency of the suppression of HVR has been proposed as the following order: halothane > enflurane > sevoflurane > isoflurane > desflurane.

Certain patient populations are particularly vulnerable to the inhibition of hypoxic respiratory drive at subanesthetic concentrations, including premature infants and patients with OSA. The depressant effect on the HVR is due to selective inhibition of the peripheral chemoreflex loop. The carotid body appears to be the most likely target. However, the mechanisms by which subanesthetic concentrations of volatile anesthetics attenuate hypoxic ventilatory drive are not completely understood. Interestingly, acute pain and central nervous system arousal do not restore impaired HVR during sevoflurane sedation. Thus compromise of the central nervous system arousal state per se does not contribute to the impairment of the acute HVR by sevoflurane. Additionally, audiovisual stimulation does not prevent the blunting of acute HVR by low-dose halothane, but by isoflurane. This finding raises the possibility that volatile anesthetics might differently affect the hypoxic chemoreflex loop.
Effects of Inhaled Anesthetics on Respiratory Muscle Activity
Humans are bipeds and very different from the quadruped mammals in terms of contribution of various muscle groups, particularly the truncal muscles, to the normal respiratory effort, probably due to differences in posture. Thus the results of respiratory control studies in animals could not be directly extrapolated to humans, whether under normal resting conditions or under anesthesia. At quiet breathing in supine and conscious nonpregnant adult humans, scalene and parasternal inspiratory activity is universally present, but abdominal expiratory activity is absent. This means that at quiet breathing, expiration is mostly passive and likely occurs via recoiling of chest wall and lungs. When breathing is stimulated by inhaling CO 2 , the inspiratory activity is enhanced and expiratory muscles are recruited.
Volatile anesthetics, such as halothane, depress various respiratory muscles differently ( Fig. 21.20 ). The diaphragm, as the main inspiratory muscle, is unique in that regard because it is relatively spared from the respiratory depression by volatile anesthetics, probably because of its low position in the hierarchy of the neurotransmission system. At 1 MAC of halothane, the tidal volume was reduced by 59%, respiratory rate was increased by 146%, and FRC was decreased by 335 ± 75 mL. The decrease in minute ventilation could be because of rib cage/chest wall activity but is not because of a reduction in abdominal breathing, and the contribution of the diaphragm is also substantially less affected. Abdominal expiratory activity is routinely recruited during halothane anesthesia in male subjects.

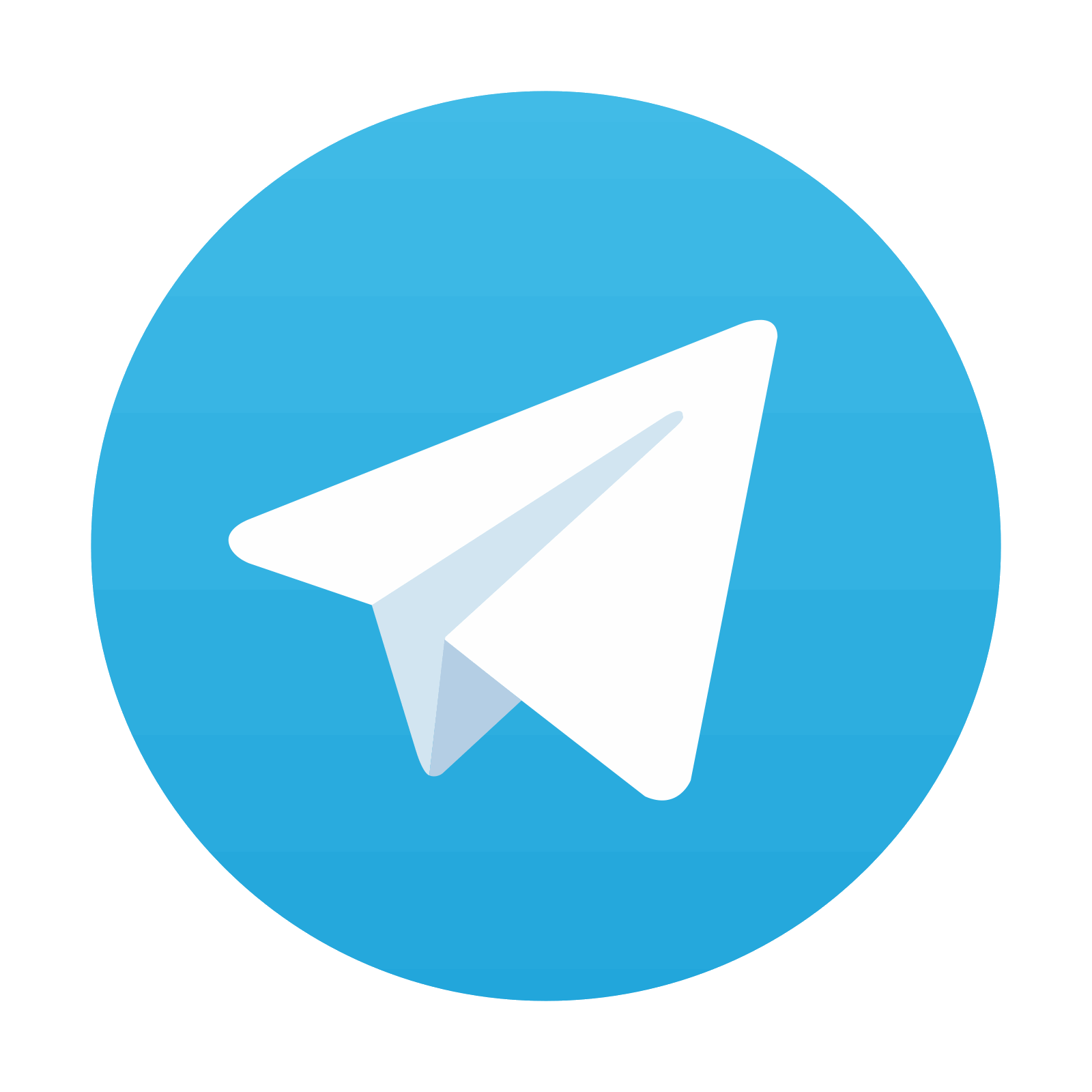
Stay updated, free articles. Join our Telegram channel
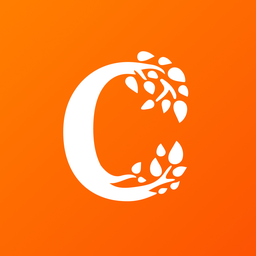
Full access? Get Clinical Tree
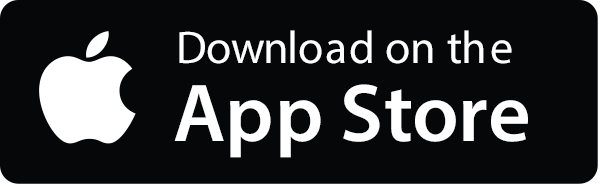
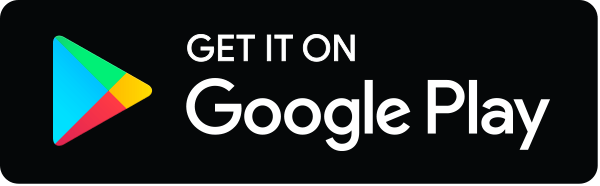
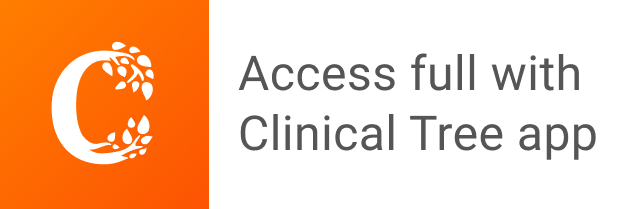