Introduction
Acute spinal cord injury (SCI) is a devastating medical condition because of the impact of permanent disability produced by paraplegia or quadriplegia. Treatment of the initial injury and reintegration of the injured patient into society place a large burden on health-care systems and society as a whole. Furthermore, acute SCI is particularly taxing from an emotional standpoint, because the patient often remains fully aware of the disability and the health- care team recognizes that most injuries are permanent and refractory to medical treatment. For these reasons, efforts aimed at the preservation of spinal cord function are primarily focused on the prevention of injury. Once injury has occurred, treatment is largely supportive.
SCI can be classified according to etiology as ischemic, toxic, or mechanical. The underlying mechanisms of spinal cord injury can be further categorized into primary versus secondary mechanisms. Primary mechanisms of injury include immediate neuronal cell death from infarction, exposure to toxic substances, or direct mechanical forces (e.g., contusion, shear, laceration, distraction, or compression). Primary mechanisms of injury may also be a consequence of direct injury to blood vessels, glial cells, or the vertebral column necessary for spinal cord function. Secondary mechanisms of injury are more complex and are the consequence of biochemical, cellular, and pathophysiologic responses to the primary injury. Secondary mechanisms exacerbate the initial injury by way of inflammation, apoptosis, intracellular protein synthesis inhibition, glutaminergic dysfunction, electrolyte shifts, neurotransmitter derangements, vascular changes, edema, and loss of energy metabolism. Secondary mechanisms result in the eventual death (over the course of days to weeks) of an additional population of neuronal or glial cells that had initially survived the primary injury. Finally, spinal cord injuries can be further characterized as complete or incomplete based on the total absence or partial presence of neurologic function below the level of the injury.
Classifying spinal cord injuries according to underlying etiology and mechanism is important because it highlights potential targets for medical intervention. Ischemic injuries may be prevented, limited, or treated by strategies directed at improving spinal cord perfusion. The severity of mechanical injuries may be abated by surgical stabilization or decompression. Medical therapies aimed at limiting secondary mechanisms of injury, often referred to as neuroprotection strategies, can be instituted after the primary injury has occurred with the aim of preventing further cell death and functional disability. In theory, preserving even a small quantity of functioning neuronal structures can result in marked differences in rehabilitation potential. However, the available evidence supporting the effectiveness of neuroprotection strategies remains controversial.
Traumatic Spinal Cord Injury
The global incidence of traumatic SCI was approximately 23 cases per million persons (179,312 cases per year) in 2007. The reported incidence of traumatic SCI in the United States alone ranged from 25 to 59 cases per million persons (12,400 cases per year) in 2010. The average age at the time of injury is 37.1 years. The large majority of new traumatic SCI cases are a result of falls, motor vehicle accidents, and injury secondary to firearms. The rate of acute in-patient mortality after traumatic SCI was 7.5% between 2010 and 2012. Survival is predicted by the patient’s age, level of spinal cord injury, and severity or grade of cord syndrome. Compared with patients with T1–S5 spinal cord injuries, patients with C1–C4 injuries had a 3.27 times greater odds of first-year mortality, and patients with C5–C8 injuries had a 2.30 times greater odds of first-year mortality. The large majority of deaths occurring within the first year post-injury are the result of cardiovascular or respiratory complications. The cumulative expense of direct medical care over the lifetime of one SCI patient ranges from 500,000 to 2 million US dollars.
The primary injury in SCI accounts for the majority of subsequent neurologic dysfunction. However, the amount of dysfunction occurring from secondary injury may also be clinically significant, and the prevention of secondary injury may be critical for long-term outcome. The approach to the prevention of secondary injury after traumatic SCI includes stabilization of cardiovascular and pulmonary function, immobilization of the spine, determination of the level and extent of injury, initiation of pharmacologic neuroprotection strategies, and surgical stabilization of the spine.
The highest management priority in traumatic SCI is cardiopulmonary resuscitation and medical stabilization of the patient because the maintenance of adequate perfusion pressure and oxygen delivery to the injured spinal cord may be critical in preventing secondary ischemic injury. Management goals in the immediate post-injury period are to ensure an adequate airway, ventilation, oxygenation, and blood pressure without worsening the spinal cord injury. Supplemental oxygen should be administered and an airway established with the head and neck immobilized in a neutral position. The treatment of airway compromise in a patient with suspected traumatic SCI is challenging because it is difficult to prevent movement of the cervical spine during emergency maneuvers to establish a patent airway. All airway maneuvers cause some amount of cervical spine movement. However, the degrees of movement are often small and the contribution of these movements to secondary injury is not clearly established. In a patient with evidence of airway obstruction and hypoventilation, the jaw-thrust maneuver should be tried initially. This maneuver is performed by placing the palms of both hands on either side of the patient’s head to provide in-line stabilization of the neck and using the first two or three fingers of each hand to lift the mandible forward and outward, without extending the cervical spine. The jaw-thrust maneuver can be combined with the use of an oral or nasopharyngeal airway or used to facilitate mask ventilation ( Fig. 23.1 ).

If ventilation is inadequate, tracheal intubation should be performed with the two-person orotracheal or nasotracheal technique. The two-person technique requires one rescuer to provide manual in-line immobilization of the head and neck while the other rescuer performs tracheal intubation. Manual in-line immobilization is performed by cradling the occiput in the palms of the hands and gently applying forces that are equal and opposite to those being applied during laryngoscopy and tracheal intubation, with the goal of keeping the head and neck in a neutral position. It is important to avoid traction of the neck during manual in-line immobilization because it could cause distraction of the spine at the site of injury. If time permits and equipment is available, tracheal intubation can be performed using fiberoptic bronchoscopic techniques instead of direct laryngoscopy to decrease the risk of spinal movement. However, if the airway is not adequately topicalized during fiberoptic bronchoscopy, subsequent coughing may lead to increased motion of the spine. Moreover, visualization by fiberoptic bronchoscopy can be challenging in the presence of secretions, blood, or emesis. Ultimately, outcome data are lacking that demonstrate the advantage of any specific technique for tracheal intubation. If an airway cannot be established by oral or nasotracheal intubation, emergency cricothyroidotomy should be considered.
Blood pressure should be measured and treatment directed to maintain a mean arterial pressure (MAP) in the range of 85 to 90 mmHg during the first week after traumatic injury. Hypotension associated with traumatic SCI may be caused by hypovolemic or neurogenic shock with sympathetic denervation. The etiology of hypotension should be determined and treated with intravascular volume expansion, vasopressor therapy, or inotropic medications. Expert opinion, observational studies, and published clinical guidelines support the treatment of hypoxia and hypotension to lessen secondary ischemic injury and to improve outcomes after acute traumatic SCI.
After initial resuscitation of the patient with suspected acute traumatic SCI, the next priority is immobilization of the head, neck, and body until radiographic assessment for spinal fracture can be performed. Optimally, immobilization should be performed concurrently with resuscitation, but there may need to be a compromise if it interferes with efforts to reestablish cardiopulmonary function or treatment of other immediately life-threatening injuries. The entire spine, including the cervical spine, should be immobilized if the site of injury is not known. Clinical indications for immobilization of the spine include trauma from motor vehicle accidents, diving accidents, or acceleration–deceleration injuries that have a high risk of associated spinal cord injury. Indications for immobilization also include physical signs of tenderness along the posterior spine, decreased cervical range of motion, pain with motion, neurologic deficits, deformities, or decreased level of consciousness prohibiting neurologic examination.
The first step in immobilization is manual stabilization of the head without applying traction until the application of an appropriate-sized rigid or semi-rigid cervical collar. The patient can then be log-rolled with the spine in a neutral position onto a rigid spine board. The body is positioned on the board with the arms straightened with the palms against the body and the legs straightened with the ankles secured to each other with padding between them. Straps are applied to the lower extremities, the pelvis, and then the trunk, in that order, to secure the body snugly against the board. Tape is then applied across the forehead and the cervical collar to prevent head and neck movement. Wedges or a lightweight head block provide additional stability to prevent lateral movement of the head and neck. If there is obvious deformity of the spine, immobilization should be modified to maintain a neutral position of the deformity.
The objective of immobilization is to maintain the spine in a neutral position. Neutral position can be defined as the normal anatomic position of the head and torso when standing and looking straight ahead. Achieving a neutral cervical spine position in adults requires elevating the occiput approximately 2 cm above the spine board. The occipital elevation distance may need to be greater than 2 cm in patients with truncal obesity. In contrast, achieving a neutral cervical spine position in children on a spine board may require elevating the back and shoulders to accommodate a relatively larger head.
The proper radiographic evaluation of a patient with suspected SCI varies based on the availability of imaging modalities, level of consciousness, and the presence of systemic or neurologic deficit. The most conservative approach would be to require immobilization until radiologic evaluation of the patient is obtained. However, two sets of criteria have been developed to identify low-risk patients who do not require radiographic clearance. The National Emergency X-Radiography Utilization Study (NEXUS) identified five criteria that must be met in order to exclude patients from radiologic evaluation: lack of midline cervical tenderness, lack of neurologic deficits, normal consciousness, no intoxication, and lack of significant systemic injuries that affect the ability to examine the patient adequately ( Table 23.1 ). This decision tool demonstrated a 99.8% negative predictive value for cervical spine injury (99% sensitivity, 12.9% specificity). The Canadian C-Spine Rule for Radiography (CCSRR) after trauma examined only alert and medically stable patients. The decision to image the cervical spine was based on whether the patient had any high-risk factors (age greater than 65 years, paresthesia, mechanism of injury) or low-risk factors (simple rear-end motor vehicle collision, sitting position in emergency department, ambulatory at any time since injury, delayed onset of neck pain, or absence of midline cervical spine tenderness) for injury, followed by the ability to rotate the head actively 45 degrees to the right and to the left ( Fig. 23.2 ). This study demonstrated 100% sensitivity and 42.5% specificity for identifying clinically significant cervical spine injuries in 8924 patients. Patients with potential cervical spine injury who meet both the NEXUS and CCSRR criteria can have spinal precautions relaxed and their cervical spines cleared without radiologic evaluation.
Cervical-spine radiography is clinically indicated for patients with trauma unless all of the following criteria are met:
|

In patients with suspected cervical spinal injury based on NEXUS and CCSRR criteria, radiographic evaluation begins with a three-view cervical series (lateral, anteroposterior, and odontoid). When done with adequate technique, three-view cervical spine plain films have a sensitivity for detecting injury approaching 90%. A large percentage of cervical spine films are inadequate because of lack of visualization of the craniocervical and cervical-thoracic junction. The combination of cervical spine radiographs and supplemental computed tomography (CT) through the areas that are poorly visualized increases negative predictive values to 99% ( Fig. 23.3 ). Clearance in the awake and neurologically normal patient is then completed by moving the neck actively and evaluating for pain. In a patient who cannot be evaluated clinically but is at high risk of a cervical fracture, the three-view cervical series, supplemented by high resolution CT imaging, reduces the risk of missing a fracture to less than 1%. In patients who have normal plain films and cervical CT imaging, but who are suspected of having spinal cord injury (e.g., presence of neurologic deficits or cervical pain), magnetic resonance imaging (MRI) should be considered for clearance. The benefit of MRI in the evaluation of traumatic SCI is not well defined, but it may help to identify occult ligamentous injury, disc herniation, or epidural hematoma.

Guidelines for performing a detailed neurologic assessment for spinal cord injury have been developed by the American Spinal Injury Association (ASIA). Testing for sensation is performed in response to pinprick and light touch over each of the 28 dermatomes (C2 to S4–5) on both the right and left sides of the body. Sensation to light touch and pin prick is scored as 2 (normal), 1 (altered), or 0 (absent), compared with sensation on the patient’s cheek as the normal frame of reference. The sensory level is defined by the most caudal dermatome with normal sensation. Motor function is examined by testing key muscle functions corresponding to 10 paired myotomes (C5–T1 and L2–S1). Strength is rated on a six-point scale ranging from 0 to 5, with 0 indicating total paralysis and 5 indicating full strength against resistance. The motor level is defined by the lowest key muscle function with a grade of at least 3 (anti-gravity function). This standardized neurologic examination and the ASIA scale has been shown to correlate with clinical outcomes and therefore can be used to stratify patients according to the severity of neurologic injury ( Fig. 23.4 ). Deep anal pressure awareness (innervated by the somatosensory components of the pudendal nerve from S4–5) is evaluated by applying gentle pressure to the anorectal wall. The external anal sphincter (innervated by the somatic motor components of the pudendal nerve from S2–4) is evaluated by the absence or presence of reproducible voluntary contractions around the examiner’s finger.


Pharmacologic therapies for the treatment of acute SCI have shown little benefit. The corticosteroid methylprednisolone has been the most extensively studied pharmacologic agent for SCI. Its mechanism of action is thought to involve reduction of cord edema, support of energy metabolism, anti-inflammatory properties, membrane stabilization, antioxidant effects, and free-radical scavenging. There is controversial class I clinical evidence to support its use in SCI. This controversy has arisen over several large clinical trials. The first National Acute Spinal Cord Injury Studies (NASCIS) trial in 1984 randomized 330 patients to either high or low dose methylprednisolone every 6 hours for 10 days. There was no placebo arm in NASCIS I. The trial demonstrated a statistically significant increase in wound infections, but no difference in motor or sensory clinical outcomes between the two treatment groups.
The landmark NASCIS II study in 1990 was a double-blind, controlled, multicenter trial in which 487 patients were randomized into one of three treatment arms: (1) methylprednisolone 30 mg/kg bolus followed by 5.4 mg/kg/h for 23 hours; (2) naloxone 5.4 mg/kg bolus followed by 0.5 mg/kg/h for 23 hours; or (3) placebo. The NASCIS II trial showed no difference in clinical outcomes at 1 year, although a secondary analysis demonstrated that patients given methylprednisolone within 8 hours of injury had a statistically significant improvement in their motor scores compared with study patients who received methylprednisolone after 8 hours.
The NASCIS III trial in 1997 was a multicenter study in which patients who presented within 8 hours of acute traumatic SCI received a bolus dose of methylprednisolone (30 mg/kg) and were then randomized into one of three treatment arms: (1) methylprednisolone 5.4 mg/kg/h for 24 hours; (2) methylprednisolone 5.4 mg/kg/h for 48 hours; or (3) tirilazad mesylate (an inhibitor of lipid peroxidation) 2.5 mg/kg every 6 hours for 48 hours. The trial showed no significant improvement in any of the primary outcome measures at 1 year. However, secondary analysis demonstrated that in patients who started treatment more than 3 hours after injury, 48 hours of methylprednisolone was associated with an improvement in motor function at both 6 weeks and 6 months compared with 24 hours of methylprednisolone treatment.
Methylprednisolone was associated with a higher complication rate in all the NASCIS trials, although mortality was similar in all treatment groups. Complications included wound infection, pneumonia, and sepsis. Even though the NASCIS trials were well-designed, randomized controlled trials, the validity of the data showing benefit has been called into question. Primary analyses in all three trials failed to show significant improvement with methylprednisolone therapy in acute SCI. Improved outcomes were only seen in secondary (and possibly post hoc) analyses. Some experts argue that such findings, while interesting, are not sufficient to support a standard of care in the treatment of patients with acute SCI. The Consortium for Spinal Cord Medicine concluded that because of insufficient evidence of clinical benefit, the routine use of methylprednisolone in acute SCI is not recommended. If it is used in this context, methylprednisolone should not be started more than 8 hours after injury. Methylprednisolone is contraindicated in patients with acute SCI and concurrent traumatic brain injury (TBI) because corticosteroid therapy has been shown to be associated with increased mortality in patients with moderate to severe TBI.
Other pharmaceuticals studied in randomized clinical trials for SCI (e.g. GM-1 ganglioside, tirilazad mesylate, naloxone) have not shown any benefit. Emerging treatments for acute SCI include therapeutic hypothermia, transplantation of progenitor or stem cells into the injured spinal cord, neuroprotective agents (e.g., riluzole, minocycline, basic fibroblast growth factor), and neuroregenerative agents (e.g., Cethrin, anti-Nogo).
Surgery for patients who have suffered traumatic SCI can be considered for stabilization of an unstable spine, to reduce a deformity, or to decompress neural tissues. Surgical stabilization of unstable spine lesions may prevent primary neurologic injury. Incomplete injuries or progressive neurologic injuries are more likely to benefit from decompressive surgery for lesions impinging on the spinal cord. Surgery to relieve a complete neurologic injury is not indicated because primary cell death has already occurred and reversal of this deficit will not occur. Most unstable injuries require anterior and posterior fixation, although some cervical injuries require anterior discectomy and fusion or posterior fixation alone. The treatment of vertebral fractures is controversial and may benefit from conservative treatment with an orthosis. Although they do not result in an unstable spine, vertebral burst fractures may require surgery because the lesion may result in collapse of the vertebral body with time, causing the loss of anterior height or kyphosis. There are no definitive guidelines regarding the timing (early versus late) of decompressive surgery. The available evidence supports early surgery in patients with cervical spine injuries. However, evidence regarding patients with injury at other spinal cord levels is limited.
Paraplegia After Thoracic or Thoracoabdominal Aortic Aneurysm Repair
Despite improvements in surgical, anesthetic, and perioperative care, spinal cord ischemia leading to postoperative paraplegia or paraparesis remains a major cause of morbidity and mortality after open and endovascular repair of descending thoracic and thoracoabdominal aortic aneurysms (TAAA) ( Fig. 23.5 ). Spinal cord ischemia occurs as a consequence of the temporary or permanent interruption of blood flow from intercostal and segmental arteries originating within the diseased segments of the descending thoracic or thoracoabdominal aorta. Open surgical repair involves replacement of the diseased segment of the aorta with an interposition graft that requires the temporary interruption of blood flow through the aorta and the sacrifice of intercostal and segmental arteries that are not reimplanted within the replaced segment of the aorta. Endovascular repair does not require the interruption of blood flow through the aorta, but instead excludes the intercostal and segmental arteries that fall within the covered segments of the aorta. After open surgical or endovascular repair of the thoracic or thoracoabdominal aorta, spinal cord perfusion becomes more dependent on collateral supply from remaining perfused intercostal and segmental arteries, the vertebral arteries, and the hypogastric vascular network.

An understanding of the vascular anatomy of the spinal cord is critical for the prevention, detection, and treatment of spinal cord ischemia. The blood supply to the spinal cord is composed of a complex collateral network ( Fig. 23.6 ). The anterior spinal cord is primarily supplied by the anterior spinal artery, which is formed from the vertebral arteries after they branch off the subclavian arteries. The posterior spinal cord is primarily supplied by the posterior spinal arteries, which are formed from either the vertebral or posterior inferior cerebellar arteries. As the spinal arteries travel caudally toward the thoracic and lumbar regions, their contribution to spinal cord perfusion decreases while blood flow from collateral vessels become increasingly important. Collateral flow is derived from arteries originating in the thoracic aorta. More specifically, dorsal branches of the posterior intercostal arteries give rise to segmental medullary arteries that, in turn, give rise to anterior and posterior radicular arteries. These radicular arteries subsequently form a network of anastomoses with the anterior and posterior spinal arteries. Anterior radicular arteries and sacral segmental arteries also provide collateral contributions to the anterior spinal artery. One large segmental artery in particular, the arteria radicularis magna that is also known as the artery of Adamkiewicz, is believed to be a major contributor of blood supply to the lower two-thirds of the thoracolumbar spinal cord.

The incidence of spinal cord ischemia after thoracic and thoracoabdominal aortic aneurysm repair ranges between 2.1% and 37.5%. The most significant risk factors for spinal cord ischemia are the extent of diseased aorta or the length of the interposition or endovascular stent graft ( Fig. 23.7 ). Endovascular repair was initially thought to be associated with a decreased risk of spinal cord ischemia. However, subsequent analyses demonstrated that the lower incidence of spinal cord ischemia was observed among patients undergoing endovascular repair of isolated descending thoracic aortic aneurysms. When patients undergoing endovascular repair were stratified by extent of their aneurysms, the incidence of spinal cord ischemia was comparable to that associated with open surgical repair. Additional risk factors associated with spinal cord ischemia include proximity of the aneurysm to the artery of Adamkiewicz, cross-clamp time during open surgical repair, the number of segmental or intercostal arteries sacrificed during open surgical repair, perioperative hypotension, or emergency operations. Risk factors associated with spinal cord ischemia after endovascular repair include the length of the endovascular stent graft, left subclavian artery coverage, coexisting renal dysfunction, iliac artery injury, or prior distal abdominal aortic operation.

Intraoperative strategies to prevent, detect, and treat spinal cord ischemia consist of surgical techniques to preserve vascular collaterals and maintain spinal cord perfusion during surgery, anesthetic techniques to decrease the metabolic demand of the spinal cord, pharmacologic agents to increase the spinal cord’s tolerance of temporary ischemia, augmentation of spinal cord perfusion, and monitoring of spinal cord function to permit early intervention ( Table 23.2 ). The original open surgical approach for TAAA repair involved the “clamp and sew” technique whereby blood flow to the descending aorta is temporarily interrupted when a clamp is placed across the proximal neck of the aneurysm while the interposition graft is sewn into place. With the “clamp and sew” technique, the risk of spinal cord ischemia is associated with the duration of aortic cross-clamping. The risk of spinal cord ischemia was 8% for cross-clamp times less than 30 minutes and up to 27% for cross-clamp times greater than 60 minutes.
Decrease the susceptibility to spinal cord ischemia |
|
Minimize the duration of spinal cord ischemia |
|
Augment spinal cord perfusion and blood flow |
|
Early detection of spinal cord ischemia |
|
Partial left heart bypass is a technique that can be used to reduce the duration of spinal cord ischemia in open surgical repairs. Partial left heart bypass involves redirecting blood from the left atrium to the distal descending aorta or femoral artery using an extracorporeal circuit while both the proximal and distal segments of the aneurysm are clamped. Perfusion is therefore maintained, at least to the areas proximal and distal to the aneurysmal segment. The distal aortic cross-clamp can be incrementally advanced toward the distal anastomosis as more proximal segments of the aneurysm are repaired. This sequential advancement of the distal aortic cross-clamp minimizes the length of descending aorta that is excluded from circulation and thereby minimizes the duration of end-organ ischemia. Occlusion or over-sewing of segmental arteries that back-bleeding into the open aorta may help to prevent vascular steal, because back-bleeding indicates the presence of collateral flow to the segmental artery.
Selective perfusion of mesenteric branch vessels can be accomplished via a perfusion circuit with individual balloon-tipped catheters. Additionally, reattachment of intercostal or segmental arteries into the interposition graft during repair may decrease the risk of spinal cord ischemia. However, the time required to reattach these vessels may ultimately prolong the duration of spinal cord ischemia. Moreover, additional vascular anastomoses to the prosthetic graft may compromise the integrity of the final repair. Surgical reimplantation of intercostal or segmental arteries is not possible during endovascular TAAA repairs. In thoracic endovascular aortic repairs (TEVAR) that require exclusion of the left subclavian artery by the endovascular stent graft, preservation of flow to the left subclavian artery may be achieved by surgical transposition of the subclavian artery onto the carotid artery or construction of a left subclavian to carotid artery bypass graft. Preservation of the left subclavian artery is thought to be important for spinal cord perfusion because its branches that include the vertebral artery supply the anterior spinal artery.
Clinical evidence to support the efficacy of pharmacologic neuroprotection strategies for the prevention and treatment of spinal cord ischemia is limited and cannot be relied upon alone for spinal cord protection. Despite the absence of definitive evidence, methylprednisolone 1 g intravenously (IV), mannitol 12.5 to 25 g IV, magnesium 1 to 2 g IV, lidocaine 100 to 200 mg IV, thiopental 0.5 to 1.5 g IV, and propofol 25–75 μg/kg of body weight per minute IV, alone or in combination, have been described and commonly used in clinical protocol as adjuncts for spinal cord protection. The use of IV naloxone 1 μg/kg of body weight per hour and intrathecal papaverine 30 mg have also been reported as part of an overall spinal cord protection strategy in several clinical studies.
Spinal cord perfusion pressure can be estimated as the difference between systemic MAP and lumbar cerebrospinal fluid (CSF) pressure. If the CSF pressure cannot be measured, spinal cord perfusion pressure can be estimated as the difference between the MAP and central venous pressure (CVP). Spinal cord perfusion pressure can be increased by decreasing the lumbar CSF pressure with CSF drainage or increasing the MAP with vasoactive drugs. At the same time, cardiac output should be maintained or augmented to increase the MAP and to maintain the CVP within a low or normal range. Drainage of CSF can be achieved by percutaneous placement of a silastic catheter into the subarachnoid space between lumbar spinal processes. The CSF pressure within the subarachnoid space is transduced via the lumbar cerebrospinal fluid catheter, and CSF is drained into a sealed reservoir for a lumbar CSF pressure exceeding 10 mmHg. The lumbar CSF drain can be placed prophylactically before open surgical or endovascular repair or after repair in the setting of delayed onset postoperative spinal cord ischemia.
Two meta-analyses have been published to assess the efficacy of lumbar CSF drainage. These meta-analyses are based on 372 published studies, including three randomized controlled trials involving 289 patients. The meta-analysis performed by Cina et al. found an odds ratio (OR) of 0.35 ( P = 0.05), indicating that lumbar CSF drainage was effective for decreasing the risk of paraplegia. The meta-analysis performed by the Cochrane Collaborative found a pooled OR of 0.48 (95% CI, 0.25–0.92) favoring the efficacy of lumbar CSF drainage in decreasing the risk of paraplegia when three randomized controlled trials were included in the meta-analysis. However, the pooled OR did not reach significance at a value of 0.57 (95% CI 0.28 to 1.17) when one of the three randomized trials was eliminated for the administration of intrathecal papaverine in combination with lumbar CSF drainage (using data from lumbar CSF drainage-only trials). The Cochrane Collaborative ultimately concluded that there was insufficient evidence to support the efficacy of lumbar CSF drainage alone, but recommended the clinical use of lumbar CSF drainage as a component of a multimodality approach for prevention of neurologic injury.
Since the publication of these two meta-analyses, evidence in favor of lumbar CSF drainage for the prevention and treatment of spinal cord ischemia has continued to accumulate for patients undergoing both open surgical and endovascular repairs. A class I recommendation for lumbar CSF drainage, as a component of a multimodal approach in the prevention of spinal cord ischemia and neurologic injury, has been made in the American College of Cardiology Foundation and American Heart Association 2010 guidelines for the management of patients with thoracic aortic disease, the 2014 European Society of Cardiology guidelines on the diagnosis and treatment of aortic diseases, and the European Association for Cardio-Thoracic Surgery position paper on spinal cord protection during thoracic and thoracoabdominal aortic surgery and endovascular aortic repair. The safety of lumbar CSF drainage appears to be acceptable, even in patients exposed to full anticoagulation for extracorporeal circulation. Complications associated with the technique include subdural hematoma, intraspinal hematoma, remote cerebellar hemorrhage, infection, and catheter fracture. The most serious complications appear to be associated with intracranial hypotension from excessive or rapid drainage of CSF.
Arterial pressure augmentation by increasing MAP, alone or in combination with lumbar CSF drainage, to increase spinal cord perfusion pressure for the prevention and treatment of spinal cord ischemia has not been tested by randomized controlled trials, but has received a class IIa recommendation (benefit >>risk: it is reasonable to perform) based on clinical experience from case series, expert opinion, and physiologic rational. In general, vasopressor agents such as norepinephrine, phenylephrine, and vasopressin are administered as IV infusions are titrated to achieve and to maintain a MAP of at least 80 mmHg to ensure a spinal cord perfusion pressure of 70 mmHg. At the same time arterial pressure is being augmented, intravascular volume expansion, transfusion, or inotropic support may be necessary to ensure that the cardiac output is adequate for perfusion and that the CVP is not elevated. In clinical practice, the MAP is increased by increments of 5 mmHg based on neurophysiologic evidence or neurologic findings on the physical examination suggesting the presence of spinal cord ischemia. Perioperative hypotension from surgical bleeding or other causes can trigger the onset of spinal cord ischemia after TAAA repair, but clinical observations suggest that unexplained hypotension may also be an early clinical sign of spinal cord ischemia. In this situation, hypotension is a consequence of spinal cord ischemia causing autonomic dysfunction from neurogenic shock. In either situation, early and immediate treatment of hypotension associated with spinal cord ischemia is necessary to prevent infarction ( Figs. 23.8 and 23.9 ). In the course of treatment, it is important to reassess continually the benefits of arterial pressure augmentation against the risk of bleeding when implementing this technique in patients with major vascular disease in the perioperative period.
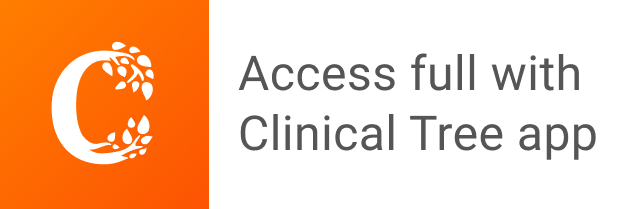