Fig. 23.1
The endocrine system (Reprinted from OpenStax, Anatomy & Physiology, OpenStax. 25 April 2013. Creative Commons Attribution 4.0 International License. Download for free at ► http://cnx.org/content/col11496/latest/)
Hormones can be excreted in different fashions. Endocrine effects occur when the hormone exerts an effect on distant organs. Paracrine effect is when 1 cell produces effects on a neighboring cell in the same organ. Autocrine effect occurs when a hormone acts on the same cell it is excreted by. Finally, a newly discovered intracrine effect is the process whereby a hormone is synthesized and acts intracellularly in the same cell. All of these modalities give the endocrine system an exquisite amount of feedback with local and distant end organ control.
Hormones circulate freely or are protein bound. Most carrier proteins are synthesized in the liver, and in states of liver dysfunction carrier protein and total hormone concentrations can be affected. Unbound hormones can be cleared from the circulation by metabolic clearance.
23.2 Hypothalamus
The hypothalamus is the main coordinating center for the endocrine system. It responds to different environmental stimuli and integrates this information with other areas of the brain and produces an endocrine response that maintains homeostasis (◘ Fig. 23.2). Thirst, food intake, energy metabolism, body temperature, and the sleep-wake cycle are under hypothalamic control. Most of the hypothalamic response is mediated through its control of the pituitary (◘ Fig. 23.3). The hypothalamus controls the release of hormones from the anterior pituitary through stimulation/inhibition via release of hypophysiotropic peptide hormones (◘ Fig. 23.4). The posterior pituitary neuropeptides are directly synthesized in the hypothalamus and released through the hypothalamo-hypophyseal tract (◘ Fig. 23.5).
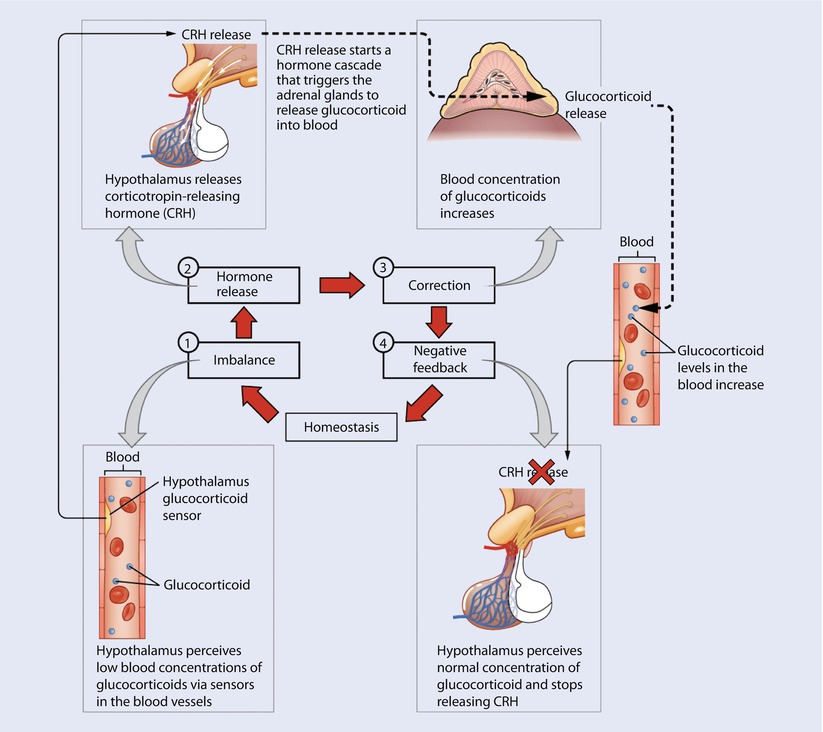
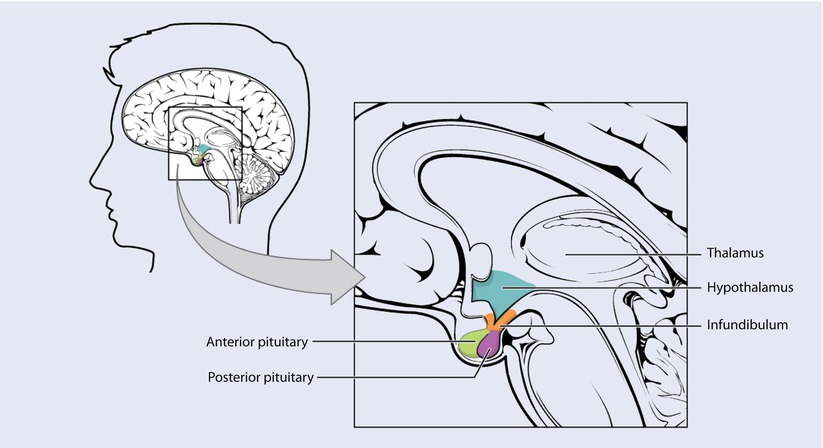
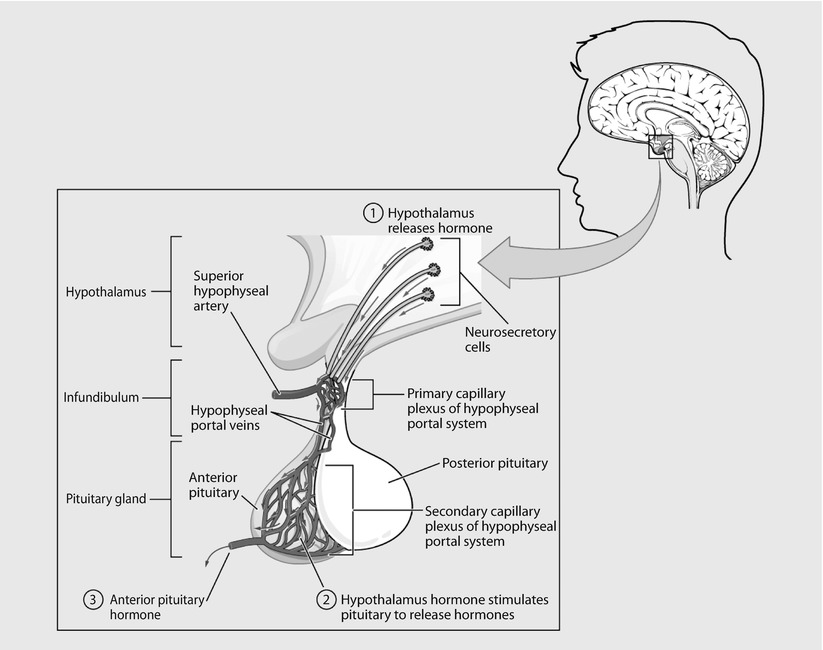
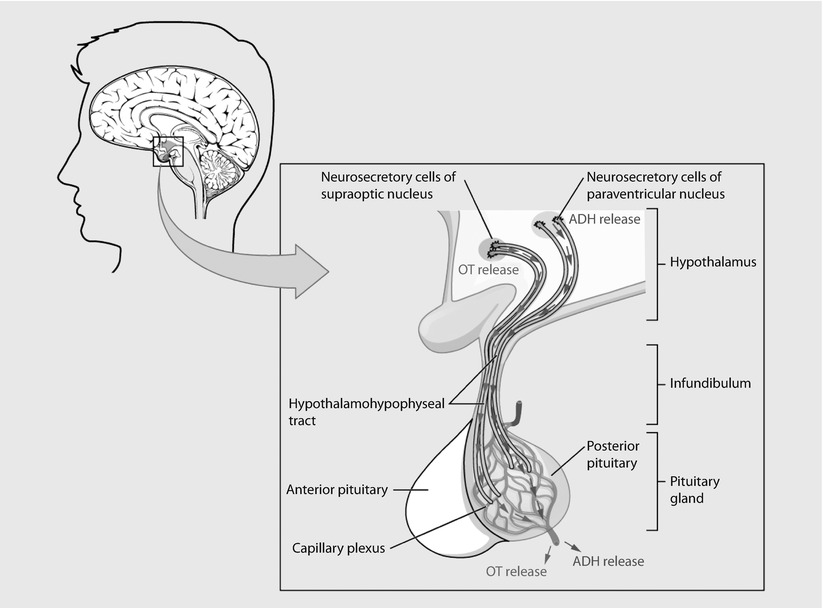
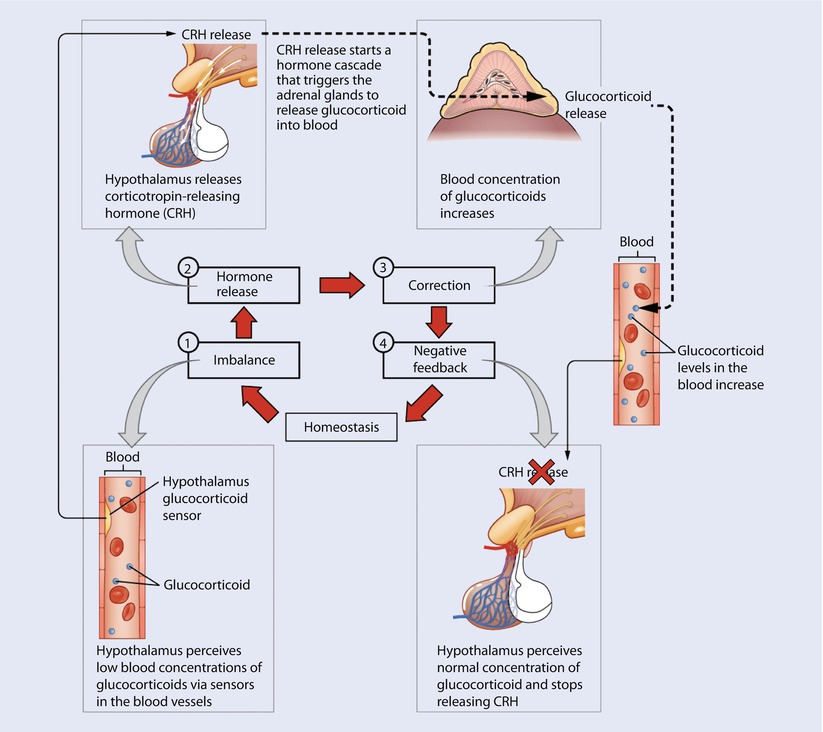
Fig. 23.2
The hypothalamus responds to different environmental stimuli and produces an endocrine response to maintain homeostasis (Reprinted from OpenStax, Anatomy & Physiology, OpenStax. 25 April 2013. Creative Commons Attribution 4.0 International License. Download for free at ► http://cnx.org/content/col11496/latest/)
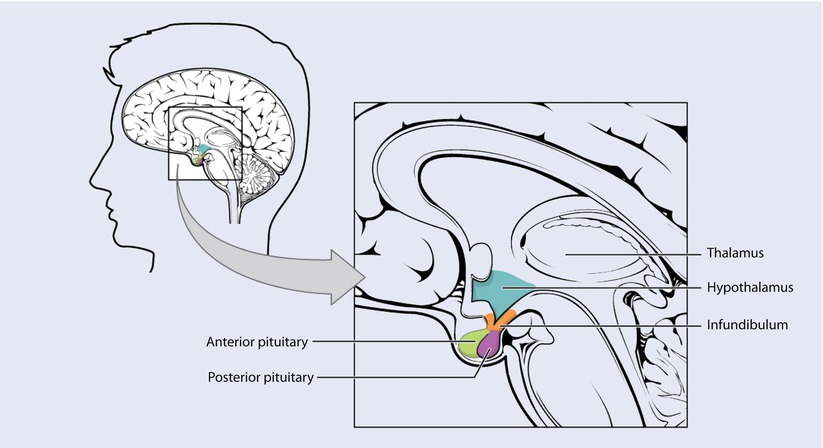
Fig. 23.3
The hypothalmus-pituitary complex. The hypothalamus connects to the pituitary gland by the stalk-like infundibulum. The pituitary gland consists of an anterior and posterior lobe, with each lobe secreting different hormones in response to signals from the hypothalamus (Reprinted from OpenStax, Anatomy & Physiology, OpenStax. 25 April 2013. Creative Commons Attribution 4.0 International License. Download for free at ► http://cnx.org/content/col11496/latest/)
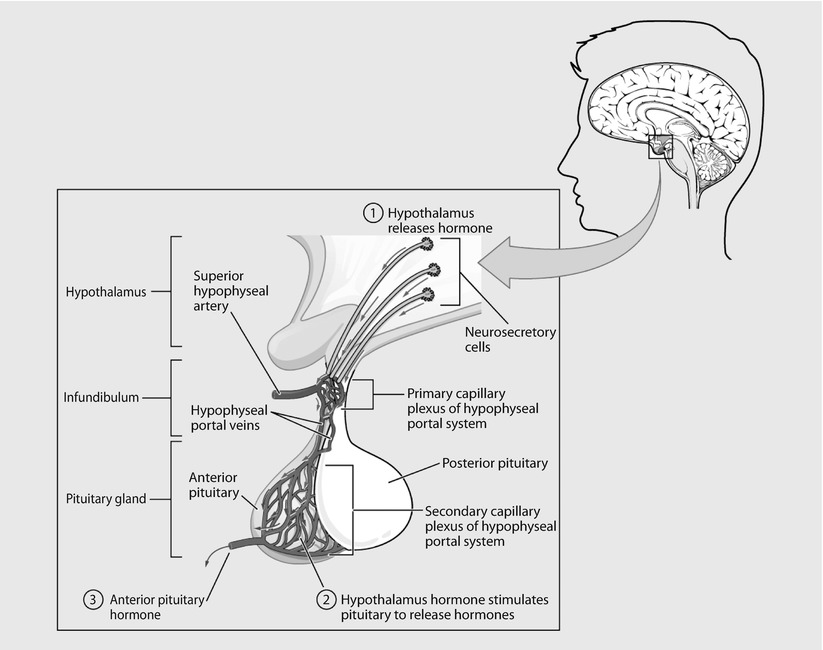
Fig. 23.4
Anterior pituitary. The anterior pituitary manufactures seven hormones. The hypothalamus produces separate hormones that stimulate or inhibit hormone production in the anterior pituitary. Hormones from the hypothalamus reach the anterior pituitary via the hypophyseal portal system (Reprinted from OpenStax, Anatomy & Physiology, OpenStax. 25 April 2013. Creative Commons Attribution 4.0 International License. Download for free at ► http://cnx.org/content/col11496/latest/)
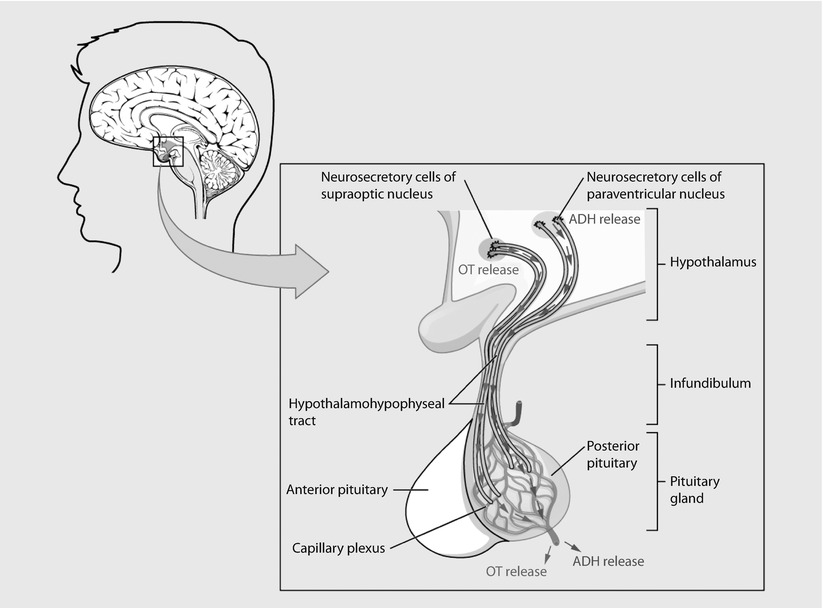
Fig. 23.5
Posterior pituitary. Neurosecretory cells in the hypothalamus release oxytocin (OT) or antidiuretic hormone (ADH) into the posterior lobe of the pituitary gland. These hormones are stored or released into the blood via the capillary plexus (Reprinted from OpenStax, Anatomy & Physiology, OpenStax. 25 April 2013. Creative Commons Attribution 4.0 International License. Download for free at ► http://cnx.org/content/col11496/latest/)
At the inferior pole of the third ventricle, the hypothalamus joins to form the median eminence. This important anatomic structure is where a portal drainage system exists. The superior hypophyseal arteries form the primary capillary plexus that supplies blood to the median eminence. From this capillary system, the blood is drained in hypophyseal portal veins into the secondary plexus. The peptides released at the median eminence enter the primary plexus capillaries. From there, they are transported to the anterior pituitary via hypophyseal portal veins to the secondary plexus. The secondary plexus is a network of fenestrated sinusoid capillaries that provide blood to the anterior pituitary.
The median eminence is also the structure that contains axons from the hypothalamus that travel down to form the posterior pituitary gland. Short portal vessels connect the posterior to the anterior pituitary allowing function to be closely associated.
Two types of neurons mediate the endocrine function of the hypothalamus. Magnocellular neurons are located in the paraventricular and supraoptic nuclei of the hypothalamus and produce oxytocin and vasopressin (AVP). Magnocellular neurons are unmyelinated and form the hypothalamo-hypophysial tract that travels through the median eminence to form the posterior pituitary. Parvicellular neurons are the cluster of neurons that terminate in the median eminence and release hypophysiotrophic hormones that control the anterior pituitary (andenohypophysis). Parvicellular neurons release CRH (corticotropin releasing hormone), LHRH (leutinizing hormone releasing hormone), TRH (thyrotropin releasing hormone), GHRH (growth hormone releasing hormone), somatostatin, and dopamine. Each hypophysiotrophic hormone then acts on the anterior pituitary causing release of specific hormones that then act on target cells. Dopamine acts as an inhibitor of prolactin release. Prolactin is the hormone that acts on lactotrophs in the breast causing milk production. Antipsychotic medications with antidopaminergic action remove inhibition, causing increased circulating prolactin levels; this is the physiology behind galactorrhea in patients on antipsychotic medications. Light plays an important role in the hypothalamic suprachiasmatic nucleus serving as an overall biologic internal clock generating circadian rhythms of hormone secretion.
23.3 Pituitary
The pituitary consists of an anterior (◘ Fig. 23.4) and a posterior lobe (◘ Fig. 23.5). The adenohypophyis, or anterior lobe, is comprised of the pars anterior and the pars intermedia separated by a remnant of Rathke’s pouch. The anterior pituitary is derived from epithelial cells from the ectodermal lining of the top of the mouth. The anterior pituitary has individual cells that are named respectively according to the different hormones each release. Corticotrophs produce adrenocorticotropic hormone (ACTH), Thyrotrophs produce thyroid-stimulating hormone (TSH), somatotrophs produce growth hormone (GH), lactotrophs produce prolactin, and gonadotrophs produce gonadotropins leutinizing hormone (LH) and follicle-stimulating hormone (FSH). The gonadotrophs (LH and FSH producing) and the somatotrophs (GH) cells are more prone to injury due to their location in the postero-lateral region.
The hormones of the anterior pituitary are classified into glycoproteins, propiomelanocortin (POMC) derived, and GH and prolactin type. The glycoprotein hormones are TSH, FSH, and LH. These hormones share a common α(alpha) subunit and a unique β(beta) subunit that confers specificity. POMC type hormones are ACTH, β(beta) endorphin, and melanocyte-stimulating hormone (MSH). Growth hormone and prolactin share a similar structure to human placental lactogen.
Thyroid-stimulating hormone (TSH) is secreted by thyrotrophs and binds to a g-protein coupled receptor in the thyroid gland leading to hormone synthesis. TSH secretion is regulated by stimulation from TRH from the hypothalamus and negative feedback is provided by T3 and T4.
Gonadotropins produce LH and FSH, engaging the testes and ovaries causing sex hormone synthesis, spermatogenesis, folliculogensis, and ovulation. The gonadotrophs are under exquisite feedback control that allows for cyclical release leading to menstruation and sexual maturity. Testosterone and estrogen provide negative feedback control.
Corticotrophs release POMC type hormones that are post-translationally cleaved into ACTH, β(beta) endorphin, and melanocyte-stimulating hormone (MSH). ACTH is released in response to stress and binds to the adrenal cortex that leads to release of glucocorticoids and mineralocorticoids. MSH binds to skin melanocytes to increase melanin synthesis. Diseases involving adrenal dysfunction lead to overproduction of ACTH and MSH causing the pathognomonic skin tanning in Addisons disease. β(beta) endorphin is an endogenous opioid that produces analgesia along with neuromodulatory effects on behavior and nervous function. Negative feedback is provided by cortisol.
Growth hormone is released by somatotrophs (acidotrophs) in pulsatile bursts that interact with metabolism, sex steroids, adrenal glucocorticoids, thyroid hormone, and renal and hepatic functions. Growth hormone is inhibited by somatostatin and stimulated by GHRH release from the hypothalamus. The release of growth hormone is stimulated by thyroid hormone, dopamine, catecholamines during stress, excitatory amino acids, and hypoglycemia.
The majority of GH circulates bound to GH-binding protein and its half-life is 6–20 min. The principal effect of growth hormone is to promote longitudinal growth and also functions to regulate metabolism, adipocyte differentiation, maintenance and development of the immune system, and regulation of brain and cardiac function. In adipose tissue, GH causes oxidation of free fatty acids raising levels of circulating lipid available for energy metabolism. GH acts in skeletal muscles to effect anabolic growth. Insulin-like growth factor 1 (IGF-1) is produced in the liver in response to GH. The IGF-1 binds to the insulin receptor and to IGF-1 receptor leading to bone formation, protein synthesis, and glucose uptake by muscle, neuronal survival, and myelin synthesis. The cellular message is transduced by the phosphorylation of Jak 2 tyrosine kinase receptors and secondary messengers. The half-life of free IGF-1 is 15–20 min, however, most of it circulates bound to IGF binding proteins. IGF-1 participates in negative feedback loops that decrease GH release. The immune system cellular function is dependent on GH for activation of B cells, natural killer cells, macrophages, and T cells.
An excess of growth hormone causes the syndrome of acromegaly. Adult patients with acromegaly can present with symptoms that result from mass effect secondary to excessive growth hormone: they can have headache, visual field defects, rhinorrea, skeletal overgrowth, soft tissue overgrowth, and connective tissue overgrowth causing recurrent laryngeal nerve palsy and carpal tunnel syndrome. Intraoperative considerations include visceromegaly, glucose intolerance, osteroporosis, hyperhidrosis, and increased lung volumes. Patients with acromegaly may present for transphenoidal resection of the pituitary gland due to GH-secreting tumor. These patients should be treated as difficult airways as a result of reduction in the size of the glottic opening, hypertrophy of aryepiglottic folds, calcinosis of the larynx, recurrent laryngeal nerve injury, prognathism, and hypertrophy of the tongue.
Prolactin is released from lactotrophs. Prolactin is under inhibitory control by dopamine in the hypothalamus and also gamma-aminobutyric acid (GABA) and somatostatin. Tonic inhibition by dopamine is released by sucking of the nipple and increased levels of ovarian steroid hormones, primarily estrogen. TRH, oxytocin, vasoactive intestinal peptide, and neurotensin may weaken the inhibition of prolactin release. Prolactin binds to receptors in the mammary gland causing growth, milk production, and milk expulsion. Interestingly, prolactin also plays a role in maternal and sexual behavior.
The posterior pituitary is formed by the axons from the magnocellular neurons of the hypothalamus. The posterior pituitary releases AVP and oxytocin (◘ Fig. 23.5). Both are synthesized as part of a larger precursor protein (neurophysin 2) that is cleaved. AVP and oxytocin circulate unbound and have short half-lives of 1–5 min.
Oxytocin is released during suckling on the breast and stretch on the cervix during childbirth. Oxytocin stimulates the contraction of myoepithelial cells in the mammillary ducts causing milk to be expelled. Its other primary function is to induce smooth muscle contraction on the uterus that allows for labor and regression of the uterus after birth. Receptor levels and gap junctions between the smooth muscle of the uterus are increased during pregnancy, which lends to a much stronger response to circulating oxytocin. Oxytocin release is inhibited by loud noise, pain, and elevated body temperature. Deficiency of oxytocin can impair breast feeding. Patients on the labor and delivery floor that are on extended durations of oxytocin infusions are at an increased risk of postpartum hemorrhage and decreased milk production as a result of oversaturation of the oxytocin receptors.4.
Arginine vasopressin (AVP) or antidiuretic hormone (ADH) is the other hormone released from the posterior pituitary gland. AVP is released in response to elevated plasma osmolarity or a decrease in circulating blood volume. Changes in the release of AVP are more sensitive to changes in osmolarity than blood volume: A small increase of 1% plasma osmolarity is effective whereas it takes a 10% reduction in circulating blood volume to induce release. The effective plasma osmolarity is sensed by special osmoreceptor neurons in the hypothalamus. Decreases in blood volume are detected in the cardiac atria, aorta, and carotid sinus through the 9th and 10th cranial nerves. AVP regulates water reabsorption by altering water permeability at the distal convoluted tubule of the nephron. AVP also causes smooth muscle vasoconstriction in the arterioles increasing vascular resistance. Drugs and hormones such as estrogen, progesterone, opiates, nicotine, alcohol, and atrial natriuretic peptide influence AVP release.
Disorders of vasopressin include diabetes insipidus (DI) and syndrome of inappropriate antidieuretic hormone (SIADH). Diabetes insipidus is insufficiency of AVP, causing diluted urine and hypernatremia, and may be central or peripheral. Central DI, where there is a low level of AVP, may be caused by brain tumors, traumatic brain injury, and cerebral ischemia. Hypernatremia secondary to central DI is a common finding in brain dead patients presenting for organ harvest. In nephrogenic DI, damage to the renal tubules by methoxyflurane and other nephrotoxic agents (lithium, demeclocycline, ofloxacin, polycystic kidney disease) leads to receptor insensitivity to AVP, leading to a decrease in the kidney’s ability to concentrate urine. Lithium toxicity and hypercalcemia are the 2 most common medical conditions. Amyloidosis and polycystic kidney disease are also common causes for nephrogenic DI.
SIADH presents with hyponatremia and very small amounts of concentrated urine. SIADH can be the presenting symptom in tumors of the lung and brain as well as sequelae of traumatic brain injuries. Cerebral salt wasting is a rare complication of brain injury that mimics SIADH, however, the diagnosis is made when the patient shows signs of hypovolemia (◘ Table 23.1).
Table 23.1
Comparative differences between diabetes insipidus, syndrome of inappropriate antidieuretic hormone, and cerebral salt wasting
Diabetes insipidus | Syndrome of inappropriate antidieuretic hormone | Cerebral salt wasting | |
---|---|---|---|
Urine output | Polyuric | Decreased | Polyuric |
Serum sodium | High | Low | Low |
Urine sodium | Low | High | High |
Serum osmolarity | High | Low | Can be low or normal |
Urine osmolarity | Low | High | Can be low or normal |
Central venous pressure | Can be normal or low | High | Low |
Tumors, traumatic brain injury, apoplexy (acute vascular infarction or hemorrhage of the pituitary), and infection can lead to pan hypopituitarism. This disease is usually marked by an absence of hormones controlled by sections of the pituitary. If there is a tumor, visual defects (bitemporal hemianopsia) may be seen from compression of the optic chiasm. The most common cause of hypopituitarism is pituitary adenoma with compression of the normal surrounding brain tissue. The most sensitive cells to damage are those that secrete growth hormone and gonadatropic hormones. Diagnosis of hypopituitarism is made by measuring a low basal hormone level in an effector site gland in combination with a low pituitary hormone level. Treatment of hypopituitarism aims to restore levels of the hormone by giving exogenous supplement while also attempting to remove the offending cause and address any other repercussions from the deficiency.
Patients presenting for resection of pituitary tumors should have their endocrine function evaluated as well as imaging studies to rule out suprasellar extension. Most commonly, patients present with headache, hormonal excess or symptoms of hypopituitarism and visual disturbances; it is rare to have increased intracranial pressure (ICP) as the presenting symptom. If ICP is elevated, anesthetic techniques should be taken to prevent further increases. Interestingly, in cases of suprasellar extension of pituitary tumors, controlled hypercarbia may shift the tumor into the surgeon’s view assisting in resection. Stress doses of steroids may be considered if measured levels of cortisol are low or if the patient develops signs of Addisonian symptoms.
23.3.1 Thyroid
The main function of the thyroid gland is the synthesis and storage of thyroid hormone (◘ Figs. 23.6 and 23.7). The functional unit of the thyroid gland is the thyroid follicle, which consists of a layer of thyroid epithelial cells arranged around a large central cavity filled with colloid. The apical surface of the follicular cells face the follicular lumen where colloid is stored and the basolateral surface faces the bloodstream where iodine is absorbed and thyroid hormone is released. Thyroglobulin is found in this colloid.
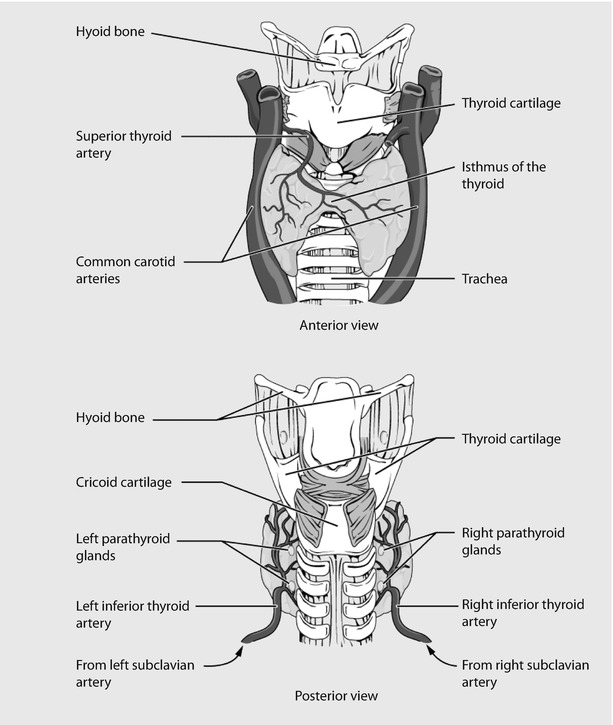
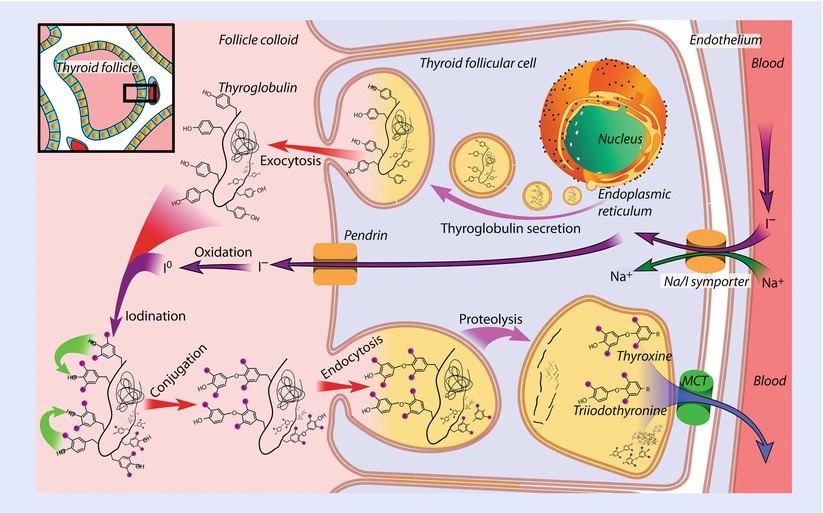
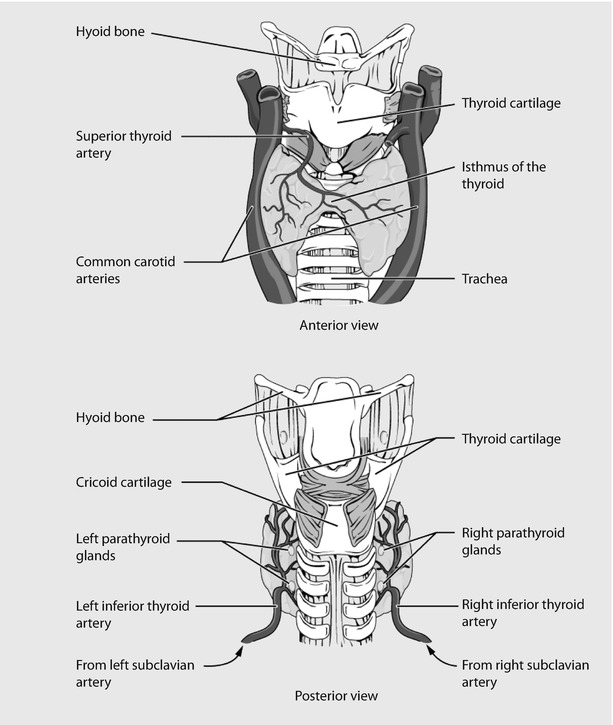
Fig. 23.6
Thyroid gland (Reprinted from OpenStax, Anatomy & Physiology, OpenStax. 25 April 2013. Creative Commons Attribution 4.0 International License. Download for free at ► http://cnx.org/content/col11496/latest/)
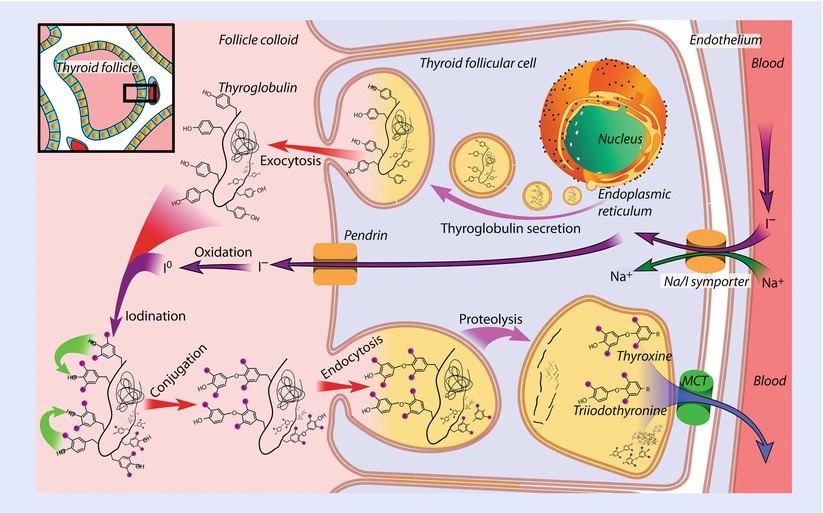
Fig. 23.7
Synthesis of the thyroid hormones, as seen on an individual thyroid follicular cell, monocarboxylate transporter (MCT) (Reprinted from Häggström [5])
TSH binds to the basolateral side of the follicular cells and causes iodide uptake through activity of the sodium-iodide symporter, transcription of thyroglobulin, and activation of thyroid peroxidase (TPO). TPO is the enzyme responsible for organification of tyrosine. TPO oxidizes iodine to iodide so that it can be added onto specific tyrosine residues on thyroglobulin causing monoiodotyrosine (MIT) and 3,5,3′-triiodothyronine (T3). Further coupling then creates diiodotyrosine (DIT) and 3,4,3′,5′-tetraiodothyronine (T4). The coupling of iodinated tyrosine residues either of 2 DIT residues or 2 MIT and 1 DIT residues is catalyzed by TPO. In the follicular lumen there is stored MIT and DIT as well as formed T3 and T4. When plasma levels of iodine are elevated 15–20-fold above normal it inhibits the organic binding of iodine within the thyroid gland: this autoregulatory phenomenon is independent of the effect of TSH and is known as the Wolf-Chaikoff effect.
TSH regulates the release of thyroid hormones from the gland. This process involves the endocytosis of vesicles containing thyroglobulin from the apical surface and these vesicles fuse with follicular epithelial phagolysosomes leading to digestion and cleavage of thyroglobulin. Once thyroglobulin is digested, the vesicles are released on the basolateral surface into the bloodstream increasing systemic levels of T4. Some of the T4 is de-iodinated to T3 in the follicular cells and the residual iodide is then recycled to participate in the synthesis of new thyroid hormone. The thyroid gland releases greater amount of T4 than T3 (40-fold greater amount of T4). Most of the circulating levels of T3 are formed through di-iodination of T4 in the periphery. T4 therefore acts as a pro-hormone for T3 because it has much less affinity for the intracellular ligand binding sites of cells. The thyroid gland can store 2–3 months of thyroid hormone in the thyroglobulin pool.
Once T3 and T4 are released in circulation, 70% is bound to thyroid-binding globulin (TBG). A small fraction of each hormone, 0.03% T4 and 0.3% of T3, are in their free form. The free form is bioavailable to enter the cell and bind to the thyroid receptor. T4 binds more tightly to TBG and therefore has a longer half-life than T3. Binding of T3 and T4 to TBG causes a circulating storage reserve pool. Thyroid hormone exerts its effects by binding to nuclear receptors TRα(alpha) and TRβ(beta) that activate DNA mRNA synthesis with subsequent protein transcription products that control calorie metabolism, anabolic growth, and development.
Thyroid hormone is a key regulator of cardiovascular function because it increases the concentration of adrenergic receptors. Thyroid function is measured by levels of TSH in addition to free T4 or total T4 and thyroid binding ratio. Thyroid binding ratio is necessary because thyroid hormone is usually bound to thyroxine binding globulin (TBG). TBG levels can be increased during pregnancy, use of oral contraceptives, hepatitis, acute intermittent porphyria, and the neonatal state. Conversely testosterone, corticosteroids, severe illness, cirrhosis, nephrotic syndrome, and phenytoin can decrease circulating levels of TBG. Changes in the amount of TBG can impact the total amount of circulating thyroid hormone, which includes the free and protein bound forms. Increases in TBG will cause a decrease in free hormone, stimulating the release of more TSH and in turn more synthesis and release of thyroid hormones. Alternately a decrease in TBG levels will increase free thyroid hormone levels, decreasing TSH release, and decreases thyroid hormone synthesis and release.
The process of de-iodination (conversion of T4-T3) occurs primarily in the liver and kidney by the enzyme deiodinase. If the iodine is removed from the carbon 5 molecule on the outer ring of T4 it forms T3; conversely if iodine is removed from the carbon 5 molecule in the inner ring it yields reverse T3 (rT3), which has little to no biologic activity. T4 and T3 are further metabolized to T2—a biologically inactive hormone that is excreted from the body in the biliary system by glucoronidation.
Thyroid hormone exerts genomic and non-genomic effects. Non-genomic effects include stimulation of activity Ca2+ − adenosine triphosphatase (ATPase) at the plasma membrane and sarcoplasmic reticulum, increased activity of the Na + /H + antiporter, and increases in oxygen consumption. The genomic effects are mediated by thyroid hormone entering the nucleus of cells to bind to ligand-activated transcription factors that influence gene transcription. T3 has higher affinity for the ligand activated transcription factors than T4. Once the transcription factor is activated it binds to enhancer sequences on DNA called hormone response elements.
Cellular transcription of proteins in response to T3 and T4 increases several metabolic functions: Na+/K+-ATPase cell membrane proteins increase oxygen consumption, uncoupling proteins enhance fatty acid oxidation and heat generation without production of ATP, and protein synthesis leads to growth and differentiation. Thyroid hormone also plays a key role in cholesterol synthesis and fasted versus fed epinephrine-induced glycogenolysis and insulin-induced glycogen synthesis.
The effects of thyroid hormone are seen in every cell of the body due to the universal nature of the receptor (◘ Fig. 23.8). In the bone it is essential for growth and development, with excess hormone placing adults at risk for osteoporosis. In the heart, thyroid hormone acts to increase gene transcription of proteins involved in the electrical cycle of contraction/relaxation (phospholamban, Ca2+ ATPase, β(beta)-adrenergic receptors, adenyl cyclase, NA+Ca+ exchanger, Na+/K+-ATPase, and voltage-gated potassium channels), which increases cardiac output and decreases systemic vascular resistance. In fat tissue, thyroid hormone regulates triglyceride and cholesterol synthesis while controlling cell growth. In the brain, thyroid hormone causes transcription of genes involved in myelination, cell differentiation, and migration and signaling all leading to growth and development.
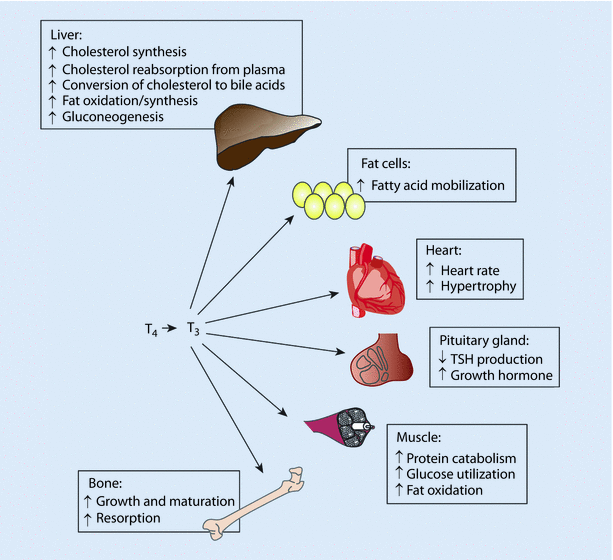
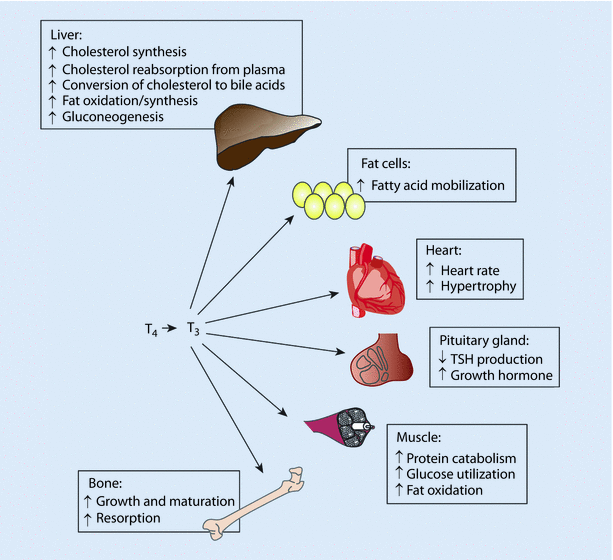
Fig. 23.8
The effects of thyroid hormone on different tissues of the body. T4 thyroxine, T3 triiodothyronine, TSH thyroid stimulating hormone (Adapted from Webb P, Phillips K, Baxter JD. Mechanisms of thyroid hormone action. ► Clinicalgate.com)
Hyperthyroidism is most commonly caused by Graves’s disease where there is a thyroid stimulating immunoglobulin that causes pathologic stimulation of the TSH receptor and release of thyroid hormone. Elevated levels of thyroid hormone can also be seen in pregnancy, thyroiditis, thyroid adenoma, choriocarcinoma, or TSH-secreting pituitary adenoma. Manifestations of hyperthyroidism are weight loss, diarrhea, warm moist skin, weakness of large proximal muscles, menstrual abnormalities, osteopenia, agitation, heat intolerance, tachycardia, cardiac arrhythmia, mitral valve prolapse, and congestive heart failure. In addition to the aforementioned classic symptomologies, mild anemia, thrombocytopenia, elevated serum alkaline phosphatase, hypercalcemia, muscle wasting, and bone loss can also occur.
Antithyroid medications methimazole and propythiouracil work by inhibiting the thyroid peroxidase enzyme that oxidizes iodide (I−) to iodine (I0), preventing the organification of tyrosine residues on the hormone precursor thyroglobulin. Propythiouracil has an additional mechanism in the periphery where it inhibits the enzyme 5′-deiodinase (tetraiodothyronine 5′ deiodinase), which converts T4 to the active form T3. While these medications can induce remission in Graves’ disease, more recent use of propranolol and iodides allows a quicker optimization (7–14 days vs 2–6 weeks). Intraoperative control of tachycardia can be achieved with boluses of esmolol, however, this alone will not prevent the occurrence of “thyroid storm.”
“Thyroid storm” is the clinical diagnosis for a life-threatening condition seen in patients whose hyperthyroidism has been exacerbated by acute stress (surgery). The patient will exhibit hyperpyrexia, tachycardia, and altered mental status. These symptoms may mimic malignant hyperthermia, pheochromocytoma, or neuroleptic malignant syndrome. Treatment is based on fluid resuscitation, administering antithyroid medications, blocking the release of thyroid hormone with iodine by utilizing the Wolf-Chaikoff effect, and supportive therapy.
Amiodarone is a medication used for cardiac arrhythmias that can cause hyperthyroidism or hypothyroidism through its action on synthesis and peripheral conversion of T4 to T3. Patients on amiodarone therapy may warrant investigation into thyroid function as well as the cardiac dysfunction that led them to require treatment.
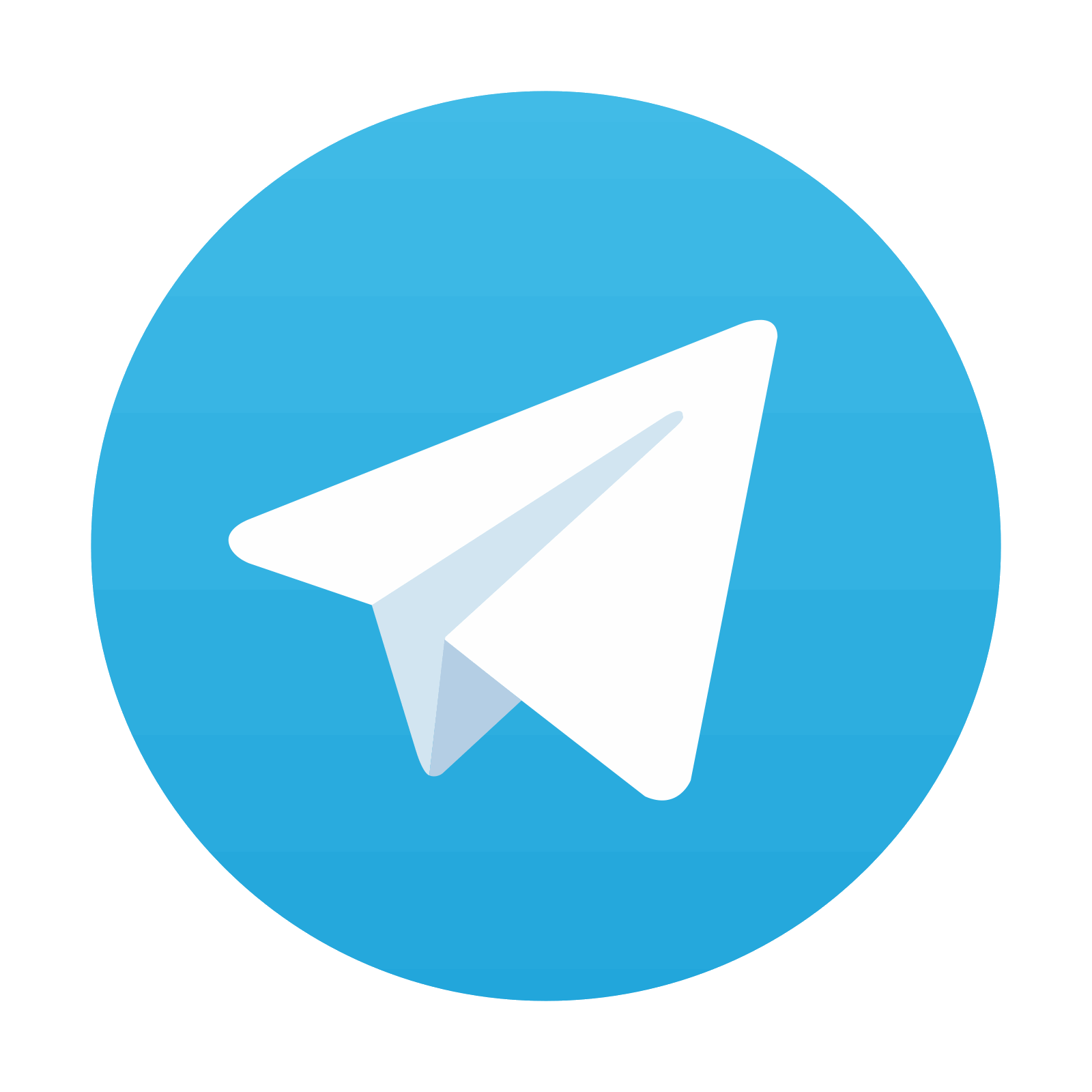
Stay updated, free articles. Join our Telegram channel
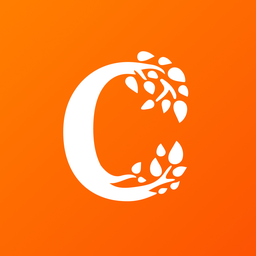
Full access? Get Clinical Tree
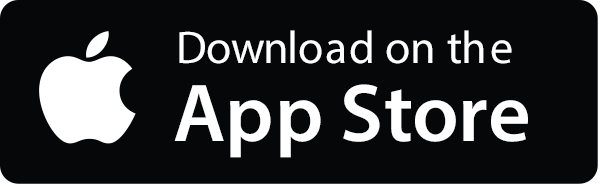
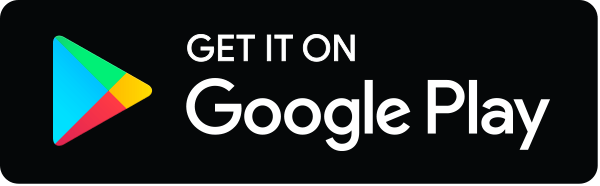