Chapter 14 Physiological monitoring
Principles and non-invasive monitoring
Introduction
Monitoring the state of both the patient’s physiology and the function of the anaesthesia delivery system is now an integral part of anaesthetic practice in the developed world. In some countries standards of monitoring are not enforced by law, yet it is difficult to prove that the absence of such monitoring is responsible for significant morbidity, since the trials required to prove this would be unethical. Anaesthesia has become safer in many countries over the last few decades, despite this fact it is difficult to demonstrate that the presence of multi-mode monitoring unequivocally reduces morbidity and mortality.1 The cost of purchasing and maintaining such monitoring means it is unavailable to anaesthetists in many countries throughout the world and so the clinical monitoring skills of the anaesthetist remain crucial. Nonetheless, even in the developed world, all such standards emphasize the need for the continual presence of trained anaesthetic personnel.
The purpose monitoring is to advise the clinician of deviations from the normal and to warn of any unexpected, physiologically threatening events. In a study of closed claims in relation to deaths and cerebral damage under anaesthesia, two-thirds were found to be due to human error; of these, two-thirds were deemed to be due to problems with securing the airway, endotracheal intubation, ventilation and hypoxia.2 A series of studies concluded that of the errors reported, 80% could be avoided by routine use of the pulse oximeter which, if used in conjunction with a capnometer, would lead to avoidance of 93% of such errors.3 Fig. 14.1 shows a graphical representation of the development of a critical incident and avoidance of the harmful results which might follow.4 Arguably, it is the function of monitors to help the anaesthetist detect a critical incident early and avoid development of injury to the patient.
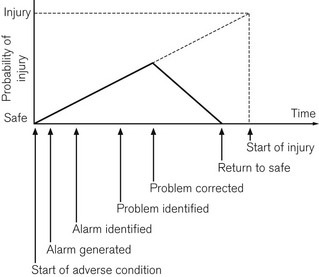
Figure 14.1 Development of a critical incident and its detection by a monitor.
From Magee P, Tooley M (2005) The physics, clinical measurement and equipment of anaesthetic practice. By permission of Oxford University Press.
There are a number of guidelines, published by various authorities such as the Association of Anaesthetists of Great Britain and Ireland (AAGBI), on minimum monitoring standards.5 These help the clinical anaesthetist select monitoring appropriate to the circumstances. It is notable how much the AAGBI guidelines defer to the anaesthetists’ own clinical judgement in this respect, since the ultimate ‘monitors’ are the anaesthetists themselves.6
Classification of monitoring equipment
There are numerous ways of classifying monitoring equipment relevant to the anaesthetist. One way might specify the physiological system that it monitors: respiratory (including gas concentration, volume, flow rate and pressure); cardiovascular (e.g. ECG, arterial and venous pressures, cardiac output); neurological (e.g. EEG, neuromuscular junction); or metabolic (e.g. temperature or blood sugar). Another might include the degree of invasiveness of the monitoring: non-invasive monitoring, such as blood pressure and electrocardiogram (ECG); partially invasive, such as naso-pharyngeal temperature; or invasive, such as central venous pressure and arterial pressure. A third method might include classification according to whether the electrical signal being monitored is naturally generated by the body (e.g. a biological electrical potential such as the ECG), transduced from another signal modality (e.g. invasive arterial blood pressure to waveform on an electronic display), or a manifestation of some form of energy input to the body (e.g. pulse oximetry, ultrasound or magnetic resonance imaging) (Fig. 14.2).
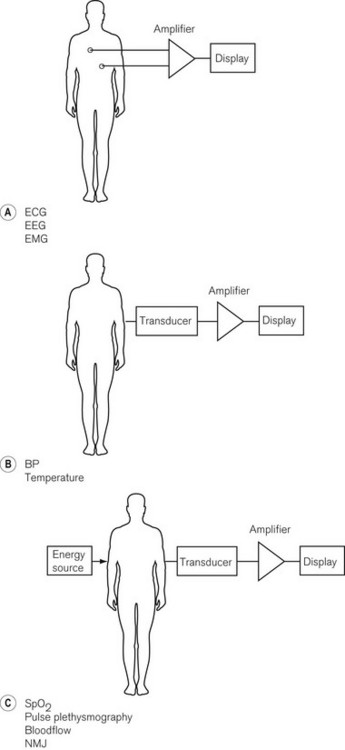
Figure 14.2 Basic classification of monitoring systems and some examples. A. Monitoring electrical signals generated by the patient. B. Conversion of measured variable to an electrical signal with a transducer. C. Passing energy through a patient and measuring the effect the patient has on it.
Important components of a monitoring system, such as an automatic non-invasive blood pressure monitor, may, therefore, include some or all of the following (Fig. 14.3):
• an energy input source to the body (e.g. mechanical electrical, electromagnetic, infrared, ultrasound)
• a transducer to convert the signal from a measured physiological variable, usually to an electrical signal which can be processed. Transducers need to be zeroed and calibrated: calibration is often done at the factory and the clinician merely has to zero the transducer
• signal processing hardware and software, which amplifies the input signal, ensures the patient is safe from electrical hazards (see Chapter 23), is robust enough not to be damaged by other equipment such as diathermy apparatus or devices containing electromagnetic fields, and provides a good-quality output, free from electrical interference
• a display for easy reading and interpretation by the clinician, who should not be obliged to become preoccupied with the monitor7
• an alarm system, with visual display and annunciation that is helpful rather than distracting, and with reasonable default limits and the ability to change those limits8,9
• modern monitoring systems also have data storage ability for subsequent data analysis, and a means of data transmission to allow the data to be used by other systems such as automated record keeping10 (Chapter 22)
• a monitoring system must be intuitive and easy to use, ergonomically designed, and reliable when used under the conditions for which it is intended.
Almost all monitoring equipment is now controlled by microprocessors, making it possible to design equipment that needs minimal user calibration, offers self-diagnostics for fault conditions and also almost endless variability in user configuration of parameters.11 Single modality monitoring is becoming increasingly rare as a result of the flexibility and miniaturization possible with microprocessor control. Even greater user variability is offered in integrated monitors (one display for several variables) by the use of a modular system, in which the module for each monitored modality may be replaced during use (hot swapped) in the event of a fault or to perform a different function.
The remainder of this chapter will cover non-invasive monitoring, whatever the physiological system being monitored or the source of the electrical signal being processed. Chapter 15 covers gas monitoring and Chapters 16 and 17 cover other aspects of cardiovascular and neurophysiological monitoring.
Monitoring biological electrical potentials
All living cells have a potential difference between the outside and the inside of the cell membrane, which generates small electric currents within and between cells. Such a potential difference, when associated with excitable cells acting in unison, such as muscle or nerve, generates a current sufficient to be detected by a measurement system. Depending on the origin of the biological potential, there is a wide range of amplitudes and frequencies to be measured, processed and displayed, as shown in Table 14.1.
Table 14.1 The range of amplitudes and frequencies generated by biological potentials from different sources
SOURCE OF BIOLOGICAL ELECTRICAL POTENTIAL | AMPLITUDES | BANDWIDTH (Hz) |
---|---|---|
ECG | 0.5–4.0 mV | 0.01–250 |
EEG | 5–300 µV | DC-150 |
EMG | 0.1–5.0 mV | DC-10000 |
The quality of the electronics within these monitors must, therefore, be of a high order to measure accurately the currents produced by such small electrical potentials within a wide range of frequencies. Amplifiers used to process biological electrical potentials should have the following properties:12
• The signal to noise ratio of the amplifiers should be high. This is the ability of an amplifier to ensure preferential amplification of the signal being measured in comparison to any electrical noise interfering with this process.
• The common mode rejection ratio should be high; this is achieved by having two input ports, one of which inverts the input signal, so that any random noise signals, such as inductively or capacitatively coupled signals, which are common to both ports, cancel each other out before entering the amplifier, while signal inputs, which are not common to both inputs to the same extent (such as the biological potential itself), are allowed to enter the amplifier. The difference between the two inputs is then subjected to electronic amplification. Fig. 14.4 shows single and double input amplifiers:


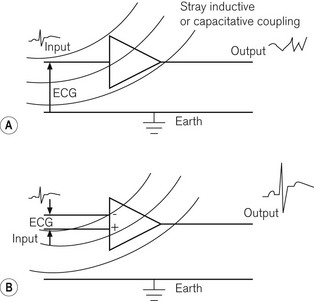
Figure 14.4 A. The output of a single input amplifier is adversely affected by stray signal coupling. B. The output of a differential input amplifier is relatively unaffected by stray signal coupling.
Even surface electrodes must be carefully designed in order to minimize degradation of the small surface electrical potentials. A further requirement of the monitors is high-quality electrical isolation between any parts touching the patient, such as the electrodes, and any other electrical components within the device, which might lead to an electrical hazard to the patient. This is particularly important, since it is the only situation where there is a deliberate electrical connection made between the patient and a device which might be connected to a high-voltage mains frequency source. If two pieces of electrical equipment are attached to a patient and one develops a fault allowing an unwanted earth pathway to occur, there is a risk of electrocution or diathermy burns. This is discussed in detail in Chapter 23.
The section below covers the electrocardiogram and monitoring of the neuromuscular junction, while Chapter 17 covers the electroencephalogram and its derivatives.
The electrocardiograph (ECG)
Despite the increasing intraoperative use of other monitors of cardiac function, such as ultrasound (see Chapter 16), the electrocardiogram remains extremely useful at detecting ischaemic events, providing close attention is paid to lead selection, amplification and filtering.13 The electrical depolarization and repolarization of the myocardium is detectable on the surface of the skin, by the electrocardiogram in the form of the familiar PQRST complex. This can be recorded by the use of electrodes connected to limbs and chest, which look at the electrical vector of the ECG from slightly different points of view. The relationship between the electrical axis of the heart and its detection by different limb leads was described by Einthoven in 1901. The signal from the electrodes is then fed into an amplifier, which should meet the requirements discussed above.
As indicated in Table 14.1, the electrical potential from a surface ECG is in the range 0.5–4 mV, lying in the frequency range (bandwidth) of 0.01–250 Hz. The ECG is a complex waveform, which consists of a series of sinusoidal waves with different amplitudes, frequencies and phase relationships to each other. The ability of the monitor to process the ECG waveform depends on its ability to respond to the range of different frequencies of these sinusoidal components, with faithful, unattenuated and undistorted reproduction of the signal, and with the desired amplification or gain. This is a measure of the bandwidth of the monitor and the ECG monitor should ideally have the maximum required bandwidth indicated above. This is not practicable without also allowing amplification of noise within that bandwidth, thereby interfering with the ECG signal. Noise can originate from the amplifier circuit itself, from chest muscle electrical activity, from electromagnetic interference (inductive coupling), or from capacitative coupling to neighbouring electrical equipment such as diathermy apparatus (see Chapter 24). It is, therefore, more common to reduce the bandwidth to 0.05–100 Hz in a monitor with which the clinician wishes to make a range of diagnoses and to an even narrower bandwidth of 0.5–40 Hz in a monitor used in the operating room. The effect of this narrower bandwidth is to exclude high- and low-frequency components of electrical signal, which interfere with the ECG without adversely affecting its quality or the clinician’s ability to diagnose ischaemia and arrhythmias.
Fig. 14.5 shows graphically the effect of electronic filtering, which is achieved by the addition of appropriate electronic components to an amplifier circuit. A low pass filter allows low-frequency components to pass through the amplifier, blocking high-frequency components. A high pass filter does the converse, allowing through high-frequency components. A band pass filter allows through a range of frequencies, blocking signals of frequencies above and below this bandwidth. There is a significant DC voltage of up to 25 mV at the skin–electrode interface, partly due to the resistance in the layers of the skin and partly due to the electrolytic reaction between the Ag/AgCl gel and metal component of the electrode assembly. An ECG amplifier has to eliminate this DC voltage as well as the high-frequency noise and, therefore, a band pass filter is appropriate.
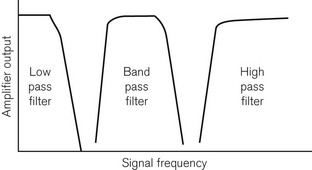
Figure 14.5 The effect of different electronic filters in allowing signals of different frequencies to pass through the amplifier.
Fig. 14.6 shows a block diagram of the components of a modern ECG monitor. As discussed above, the characteristic features include a differential input to ensure common mode rejection. Electrical isolation of the amplifier is also important for reasons discussed earlier, using either an isolation transformer with good insulation between primary and secondary windings, or an optical isolator, in which the output signal from the amplifier is converted by a light-emitting diode into a light signal and converted back into an electrical signal by a photo-detector, with good insulation between the two.
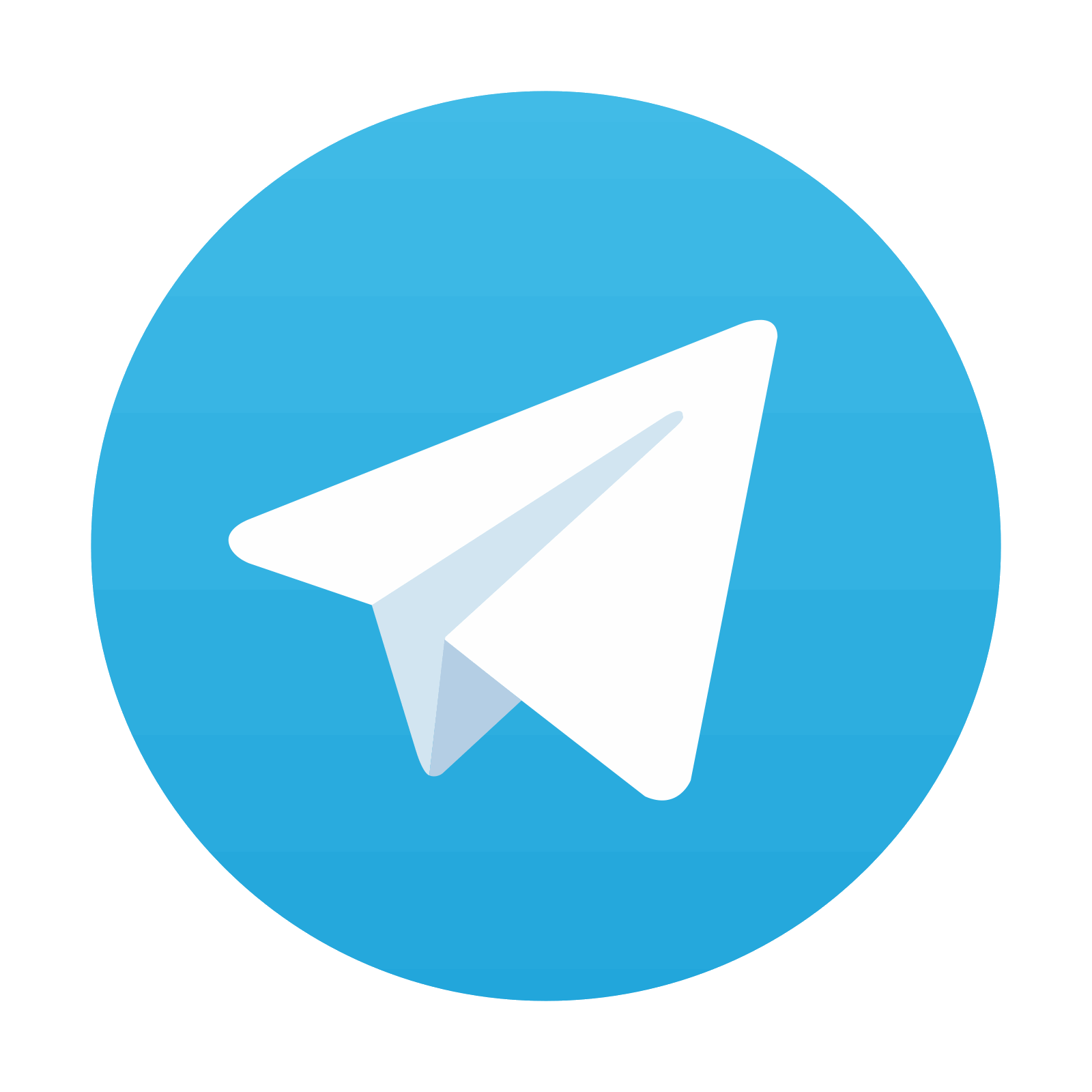
Stay updated, free articles. Join our Telegram channel
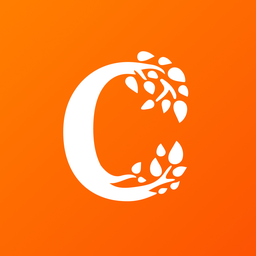
Full access? Get Clinical Tree
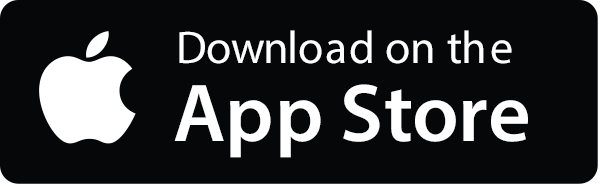
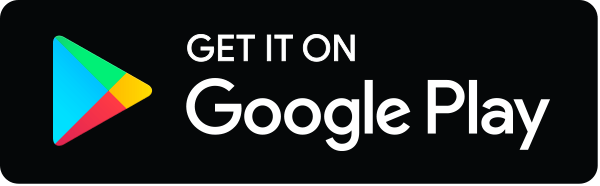