Pearls
- •
Clinical acumen and knowledge of physiology is needed to distinguish between the need for an inotropic agent, which is used to increase cardiac contractility, and the need for a vasopressor agent, which is used to increase vascular tone.
- •
The failing myocardium may need to be supported with an agent that increases contractility and reduces afterload, such as milrinone or dobutamine.
- •
Interindividual variation in pharmacokinetics and heterogeneity of the pathology seen in critically ill children mandate that response to vasoactive medications be monitored closely and that titration be based on clinical targets rather than on specific dosage levels.
In many pediatric critical care units, disorders of the cardiovascular and respiratory systems are the most frequent reasons for admission. Children with these disorders may require pharmacologic support to maintain adequate end-organ perfusion and oxygenation. The catecholamines are the class of drug most often used for this support; they remain a mainstay of therapy for the pediatric critical care physician, although the role of other agents has expanded. Milrinone, a bipyridine, is used to support patients with hemodynamic compromise of varying etiologies. Vasopressin is employed in the management of patients with vasodilatory shock or after cardiopulmonary bypass. This chapter examines the clinical pharmacology of the five clinically useful catecholamines, vasopressin, the bipyridines, and the venerable cardiac glycosides.
Mechanisms of response
Pharmacologic manipulation of the cardiovascular system often entails increasing the inotropic state of the myocardium or altering systemic vascular tone to improve perfusion. The final common mediator for both processes is the concentration of calcium in the cytosol. The pathway by which pharmacologic agents affect this parameter is a function of their specific cell surface receptors.
Adrenergic receptors
Catecholamines modify cellular physiology through their interaction with specific adrenergic receptors. The classic paradigm of α and β classes of adrenergic receptors remains unchanged, although new subtypes and sub-subtypes continue to be identified. Currently, three subtypes of α 1 -receptors (A, B, and D), three subtypes of α 2 -receptors (A, B, and C) and three subtypes of β-receptors (β 1 , β 2 , and β 3 ) are recognized. , Advances in the biology of the adrenergic receptor have led to a greater understanding of the role of the α-receptor in the heart, adrenergic receptor regulation of cardiac myocyte apoptosis, and the coupling of the β 2 -receptor to more than one G protein. The discovery of various genetic polymorphisms for the adrenergic receptors has added even more complexity, but the clinical relevance of many of these polymorphisms and their role in the pathogenesis of disease continue to be elucidated. Despite this increase in our understanding of the adrenergic receptor, the clinical classification of the catecholamines into α and β agents remains functionally unchanged ( Table 31.1 ).
Receptor | G Protein | Physiologic Response | Agonist | Antagonist |
---|---|---|---|---|
α 1 | G q | Increase InsP 3 , 1,2-DG, and intracellular Ca 2 +; muscle contraction; vasoconstriction; inhibit insulin secretion | E > NE > D | Prazosin |
α 2 | G i | Decrease cAMP; inhibit NE release; vasodilation; negative chronotropy | E > NE | Yohimbine |
β 1 | G s | Increase cAMP; inotropy, chronotropy; enhance renin secretion | I > E ≥ D ≥ NE | Propranolol, metoprolol |
β 2 | G s | Increase cAMP; smooth muscle relaxation; vasodilation; bronchodilation; enhance glucagon secretion; hypokalemia | I ≥ E > D > NE | Propranolol |
D 1 | G s | Increase cAMP; smooth muscle relaxation | D | Haloperidol, metoclopramide |
D 2 | G i | Decrease cAMP; inhibit prolactin and β-endorphin | D | Domperidone |
Signal transduction
Adrenergic receptors mediate their effects through G proteins; as such, they are classified as G protein–coupled receptors. The adrenergic receptor itself contains seven membrane-spanning α-helical domains, an extracellular N-terminal segment, and a cytosolic C-terminal segment ( Fig. 31.1 ). G proteins are heterotrimeric proteins consisting of α, β, and γ subunits, each of which has multiple subfamilies. The action mediated by a ligand binding to a particular adrenergic receptor is a function of the specific subunits comprising the G protein receptor complex.

Adrenergic receptors typically are coupled to one of three types of G proteins: G s , G i , or G q . G s proteins produce an increase in adenylate cyclase activity, while G i proteins inhibit adenylate cyclase activity. G q protein receptors stimulate phospholipase C to generate diacylglycerol and inositol 1,4,5-triphosphate. Events involving interaction of G proteins, the receptor protein, and adenylate cyclase are summarized in Fig. 31.2 . In the example of the G s protein, ligand binding to the coupled receptor causes a conformational change in the G protein, resulting in guanosine diphosphate (GDP) disassociating from the Gsα subunit and guanosine triphosphate (GTP) binding to the α subunit. This GTP-Gα complex then disassociates from the Gβγ subunit and binds to adenylate cyclase, leading to an increase in activity of this enzyme. Adenylate cyclase catalyzes the conversion of adenosine triphosphate (ATP) to cyclic adenosine monophosphate (cAMP), thus increasing cellular levels of cAMP. G i proteins have a different α subunit; when the G i α GTP complex binds to adenylate cyclase, the enzyme is inactivated. By inhibiting this enzyme, G i -coupled receptor agonists produce a decrease in the cellular concentration of cAMP. The specific cellular response that follows an alteration in the concentration of cAMP depends on the specialized function of the target cell.

β-adrenergic receptors
Myocardial β 1 -adrenergic receptors are associated with G s . When this receptor type is engaged by an agonist agent, the result is enhanced activity of adenylate cyclase and a rise in the concentration of cAMP. This process activates protein kinase A (PKA), which, in turn, phosphorylates voltage-dependent calcium channels, increasing the fraction of channels that can be opened and the probability that these channels are open, producing an increase in intracellular calcium concentration ( Fig. 31.3 ). Calcium then binds to troponin C, allowing for actin-myosin cross-bridge formation and sarcomere contraction. In addition, PKA phosphorylates phospholamban, relieving the disinhibitory effect of the unphosphorylated form on calcium channels in the sarcoplasmic reticulum. The accumulation of calcium by the sarcoplasmic reticulum increases the rate of sarcomere relaxation (lusitropy) and increases the amount of calcium available for the next contraction. This process leads to both enhanced contractility and active diastolic relaxation.

β 2 -receptors predominate in vascular smooth muscle. The β 2 -receptor is coupled to G s , thus promoting formation of cAMP. However, the activation of cAMP-dependent protein kinase in vascular smooth muscle stimulates pumps that remove calcium from the cytosol and promotes calcium uptake by the sarcoplasmic reticulum. As cytosolic calcium concentration decreases, smooth muscle relaxes and the blood vessel dilates.
α-Receptors
Vascular smooth muscle contraction is mediated via α 1 -adrenergic receptors, of which there are three subtypes: 1A, 1B, and 1D. The individual contributions of each of these subtypes to the control of vascular tone is an active area of investigation. Each subtype may be expressed in all of the vascular beds, but it is thought that one type predominates in any particular bed. α 1A – and α 1B -receptors are thought to be involved in both the heart and vasculature. , While α-receptors may have less inotropic effect than β-adrenergic receptors, they do have significant effects in the myocardium. Interestingly, in patients with heart failure, downregulation of β-receptors has been noted while α-receptors are preserved. In fact, data suggest that α 1 -receptors may display cardioprotective effects, including activation of adaptive hypertrophy, increased contractility, and prevention of myocyte death. , The α 1 -receptor is coupled to the family of G q/11 proteins, which act independently of cAMP. Signal transduction across this receptor is initiated by the activation of phospholipase C, which causes a release of calcium into the cytosol and promotes movement of extracellular calcium into the cell ( Fig. 31.4 ). In vascular smooth muscle, medium light chain kinase is activated and phosphorylates myosin light chain 2, leading to smooth muscle contraction. A similar mechanism underlies the inotropic effect of the α 1 -receptor in the myocardium. The α 1 -receptors also activate calcium influx through voltage-dependent and voltage-independent calcium channels.

Receptor downregulation
There are numerous sites at which receptor activity of the system can be modified, thereby affecting the sensitivity of target cells to both exogenous and endogenous catecholamines. The best-documented type of modification involves agonist-mediated receptor desensitization. Exposure of receptors to agonists markedly reduces the sensitivity of the target cell to the agonist. Within seconds to minutes after agonist binding, the receptor may be uncoupled as a result of receptor phosphorylation. Agonist-bound receptors may be phosphorylated by PKA or protein kinase C (PKC) or by a member of the family of G receptor kinases (GRKs). , Sequestration of receptors within the target cell and degradation of sequestered receptors is another mechanism by which receptors are downregulated. The desensitization of α 1 -receptors has been extensively reviewed. Homologous desensitization is mediated by GRKs, which are activated by soluble Gβγ subunits and phosphatidylinositol biphosphate. As with the other adrenergic receptors, once phosphorylated, the receptors are internalized into vesicles. The α 1 -receptors also demonstrate heterologous desensitization, in which a second messenger kinase, generated as a result of ligand binding, inactivates the receptor and prevents any further signaling. In addition to agonist-mediated desensitization, endotoxin, tumor necrosis factor, and congestive heart failure (CHF) have been implicated in downregulation.
Polymorphisms
Several types of genetic polymorphisms are involved in adrenergic signaling, including single-nucleotide polymorphisms (SNPs), copy number variants (CNVs), and variable number tandem repeats (VNTRs).
Few examples of functional genetic variants in adrenergic signaling that affect critical illness in children have been documented. Although these variants affect some properties of receptor function, clinical links are still being explored. , , The Arg 389 β 1 variant has been shown to moderate the effect of β-blockers on various cardiovascular measures. It may become appropriate to test for this variant when considering therapy with an agent of this class. Interestingly, the Arg 389 β 1 variant has also been associated with increased cAMP generation, poorer prognosis in heart failure, and an increase in the predisposition to hypertension.
Vasopressin receptors
Arginine vasopressin (AVP) is a nonpeptide hormone synthesized in the supraoptic and paraventricular nuclei of the hypothalamus. Three subtypes of vasopressin receptors exist, known as V 1 , V 2 , and V 3 (or V 1b ). V 2 -receptors are present in the renal collecting duct; V 1 -receptors are located in vascular beds, kidney, bladder, spleen, and hepatocytes, among other tissues. Vasopressin is released in response to small increases in plasma osmolality or large decreases in blood pressure or blood volume. The plasma osmolality threshold for release of AVP is 280 mOsm/kg. Above this threshold, there is a steep linear relation between serum osmolality and vasopressin levels. Changes in blood volume of at least 20% are needed to effect a change in vasopressin levels, although levels may then increase by 20- to 30-fold. Hypovolemia also shifts the vasopressin response curve to osmolality changes to the left and increases the slope of the curve ( Fig. 31.5 ). Vasopressin can produce vasoconstriction through V 1 -receptors in the vascular bed (discussed later), but it also activates V 1 -receptors in the central nervous system (CNS), including receptors in the area postrema. This region is responsible for the reflex bradycardia seen with vasopressin infusion, which attenuates the increase in blood pressure that would result from the vasoconstrictor effects of vasopressin. , In fact, vasopressin causes a greater reduction in heart rate than other vasoconstrictors. If this feedback loop is abolished, vasopressin induces a greater vasopressor response than other agents.

V 1 Receptors
Vasopressin receptors belong to the family of G protein–coupled receptors. V 1 -receptors are coupled to G q and V 2 -receptors are coupled to G s . When vasopressin binds to the V 1 -receptor, phospholipase C is activated, with the eventual production of InsP 3 and 1,2 DG. These molecules serve to increase the release of calcium from the endoplasmic reticulum as well as increase the entry of calcium through gated channels ( Fig. 31.6 ). The increase in intracellular calcium leads to an increase in the activity of myosin light chain kinase. This kinase acts upon myosin to increase the number of actin-myosin cross-bridges, enhancing contraction of the myocyte. Of note, vasopressin has been shown to produce vasoconstriction in the skin, skeletal muscle, and fat while producing vasodilation in the renal, pulmonary, and cerebral vasculature. This effect may be mediated though nitric oxide or may be a function of the isoform of adenyl cyclase with which the receptor is coupled. Vasopressin also has been shown to augment the pressor effects of catecholamines. V 1 -receptors have been demonstrated to have a weakly positive inotropic effect in the heart, although the clinical significance of this effect has not been established.

As with adrenergic receptors, vasopressin receptors undergo downregulation. Vasopressin promotes the phosphorylation of its own receptor immediately after binding. The receptor is removed from the cell surface within 3 minutes after binding. G protein–coupled receptor kinases (GRKs) catalyze the phosphorylation of the receptor. PKC also mediates this reaction and may serve as the means by which other agents downregulate the vasopressin receptor in a heterologous manner.
Although numerous mutations in the V 2 -receptor have been implicated in nephrogenic diabetes insipidus, much less is known about the V 1 -receptor. The molecular basis for individual genetic variation remains an active area of investigation since discoveries in this area may inform risk stratification, prognostic accuracy, and individual response to therapy.
Phosphodiesterase regulation of cyclic adenosine monophosphate
Phosphodiesterases are a class of enzyme that catalyze the hydrolysis of cAMP and cGMP into AMP and GMP, respectively. These enzymes can downregulate the signals transduced by cAMP, such as PKA activity (discussed previously). Several families of this enzyme exist, each with subtypes. Phosphodiesterase 3 (PDE3) is present in cardiac myocytes, vascular smooth muscle cells, adipocytes, platelets, and pancreatic islet cells and is functionally a cAMP esterase. Different isoforms of PDE3 are present in cardiac (PDE3A1) and vascular smooth muscle cells (PDE3A2) and are localized to different cellular compartments. Thus, they are able to regulate the function of their target enzymes in response to specific cellular signals. The bipyridines, such as milrinone, are competitive inhibitors of PDE3; that is, they bind to PDE3, preventing the enzyme from binding to cAMP. , Inhibition of PDE3 produces an increase in cAMP, resulting in a positive inotropic effect in the myocardium and vasodilation in the systemic and pulmonary vasculature. In contrast, methylxanthines such as theophylline, which inhibit all phosphodiesterases, cause levels of both cGMP (thought to decrease contractility) and cAMP to increase. This dual increase attenuates the overall inotropic effect. Bipyridines may also enhance contractility by increasing the sensitivity of myofilaments to cytosolic calcium. Milrinone has been shown to enhance the sarcomere uptake of calcium and, thereby, augment left ventricular relaxation (lusitropy). In the peripheral vasculature, PDE3 inhibitors produce vasodilation via a cGMP mechanism. The clinical effect of bipyridine administration is a combination of positive inotropy, lusitropy, and afterload reduction.
ATPase inhibition
Membrane-bound sodium-potassium adenosine triphosphatase (Na + /K + -ATPase) is responsible for maintaining electrochemical gradients across the cellular membrane. It does so by extruding three molecules of sodium from the cell and incorporating two molecules of potassium into the cell, both against their respective concentration gradients. This process occurs at the cost of one molecule of ATP. The enzyme consists of an α and β subunit; there are four subtypes of α and three of β. The β subunit may be involved in the trafficking of the enzyme. The α subunit contains both the binding site and catalytic site. The isoforms expressed are dependent on the type of tissue. Cardiac glycosides (e.g., digoxin) inhibit the Na + /K + -ATPase pump, thereby increasing intracellular sodium. The elevation in intracellular sodium alters the transmembrane gradient, thus inhibiting the activity of the voltage-gated sodium/calcium exchange (NCX) pump. This pump exchanges three molecules of extracellular sodium for one molecule of intracellular calcium. , The net result is a rise in intracellular calcium and, in the cardiac myocyte, enhanced contractility. No evidence exists to suggest the development of tolerance to digoxin with long-term use.
Developmental issues
It is often stated that the immature myocardium is less sensitive to inotropic agents than the adult heart. The mechanism responsible for these differences represents an area of active investigation. The majority of studies involve animal models or isolated human tissue.
Age-related differences in the response of the developing myocardium to inotropic agents, receptor regulation, and calcium handling depend on the species studied and model of illness to which the animals are exposed.
Because there are inherent differences between intact healthy animal models and the ill child, as well as pharmacokinetic differences between the infant and adult patient, caution must be exercised when extrapolating laboratory data to the bedside. Nonetheless, a brief review of developmental differences is appropriate.
Structural and ultrastructural differences exist between immature and mature hearts. Reduced ventricular compliance, greater ventricular interdependence, and a reduced ratio of myocardial contractile to noncontractile protein are characteristic of the immature heart. The net effect is that the immature myocardium neither responds to nor tolerates volume loading as well as the adult heart. In addition to this diminished “preload reserve,” the baseline heart rate of infants and children is quite high, which limits the extent to which tachycardia can augment cardiac output before diastolic filling is compromised.
Developmental differences also may exist in the expression of PDE isoforms. In a model of rabbit ventricular myocytes, administration of IBMX (3-isobutyl-1-methylxanthine, a nonselective PDE inhibitor) and rolipram (a PDE4 inhibitor) increased intracellular calcium currents at baseline and in response to isoproterenol in neonatal cells but not in adult cells. In contrast, milrinone (a PDE3 inhibitor) increased intracellular calcium currents at baseline and in response to isoproterenol only in the adult cells. Thus, it would appear that in the neonatal myocardium, PDE4 may be the dominant isoenzyme that regulates intracellular calcium currents.
The combination of impaired preload reserve, limited chronotropic reserve, and reduced sensitivity of the heart and peripheral vasculature to adrenergic agents implies that the response of the immature organism to inotropic and vasopressor agents may differ from the pattern noted in adults. , Indeed, a meta-review of the use of dobutamine in pediatrics concluded that while there is evidence to support that it improves cardiac output in neonates, animal studies do not adequately define the age-related effects of dobutamine on various physiologic measures or organ systems. This limitation is likely to apply to other agents employed for the treatment of shock. When coupled with the variation in cardiac anatomy due to congenital heart disease, individual differences in response to cardiovascular drugs may be difficult to predict. Therefore, meticulous attention to response is essential for safe administration of these drugs to children.
Sympathomimetic amines
Virtually all sympathomimetics currently used to support hemodynamics are catecholamines. This class includes the endogenous compounds epinephrine, norepinephrine, and dopamine and the synthetic products isoproterenol and dobutamine. Catecholamines have a β-phenylethylamine core with hydroxyl (OH) substituents at the 3 and 4 aromatic ring positions ( Fig. 31.7 ). Minor differences in molecular substitution about the N-terminus or the α or β carbons produce marked differences in activity. Structure-activity relationships are complex for the catecholamines. However, in general, increasing size of the substituent on the amino group enhances β-adrenergic activity, whereas decreasing size is associated with α-adrenergic selectivity. Tyrosine serves as the base compound for the synthesis of catecholamines. Tyrosine hydroxylase catalyzes the conversion of tyrosine to dopa, which undergoes decarboxylation, producing dopamine. Dopamine β-hydroxylase converts dopamine to norepinephrine. In the adrenal medulla, norepinephrine is converted to epinephrine by N-methyltransferase ( Fig. 31.8 ).


Catecholamines are subject to several different elimination processes. For example, a small proportion of dopamine is excreted unchanged in the urine, and it is likely that a proportion undergoes neuronal reuptake. The principal means of elimination appears to be O-methylation by catechol O-methyltransferase (COMT) to form metanephrines, followed by either sulfoconjugation (by phenolsulfotransferase) or by deamination (by monoamine oxidase [MAO]) to homovanillic acid. Substitution at the α carbon determines the rate of deamination by MAO. The contribution of each pathway to total body clearance of catecholamines varies with age and the particular drug and circulatory bed involved. In newborn lambs, the lungs accounted for 35% of the total body clearance of norepinephrine and 15% of the clearance of epinephrine. Inhibition of MAO by desipramine decreased pulmonary clearance to near zero and decreased total body clearance of norepinephrine and epinephrine by 51% and 30%, respectively. In adult rabbits, inhibition of COMT and MAO simultaneously decreased pulmonary clearance of norepinephrine, epinephrine, and dopamine but had only minor effects on total body clearance. Inhibition of COMT did not change extracellular levels of catecholamines in the CNS. Furthermore, individual differences in COMT activity are not well correlated with dopamine clearance. The liver and gut have been shown to clear between 30% to 52% of the circulating norepinephrine and epinephrine. , It is likely that processes or drugs that impair organ function and disturb these routes of elimination will decrease the overall metabolic clearance of catecholamines. Liver dysfunction is known to reduce clearance and increase the blood concentration of dopamine during a given infusion rate of the compound.
The properties of catecholamines can be divided into inotropic and vasopressor effects. An inotropic agent increases stroke work at a given preload and afterload. Typically, these agents engage receptors of the β 1 -adrenergic class. Agents that stimulate β 1 -adrenergic receptors also tend to increase heart rate modestly unless other properties of the drug prevent this increase. Some inotropic agents also activate β 2 -receptors, promoting peripheral vasodilation and reflex tachycardia. The improvement in cardiac output produced by these agents may also permit a reflex relaxation of vascular tone and systemic vascular resistance (SVR).
A vasopressor agent increases peripheral vascular tone, elevating SVR and blood pressure. Typically, vasopressors engage α 1 -adrenergic receptors, causing contraction of vascular smooth muscle. In principle, the physician will use a vasopressor agent to treat peripheral vasoplegia and an inotropic agent when the major problem is impaired cardiac contractility. In practice, most available agents display a blend of inotropic, chronotropic, and vasopressor activity that is often dose dependent. Norepinephrine has both inotropic and vasopressor effects, although it is most commonly used as a vasopressor agent. Phenylephrine, which is not a catecholamine, has considerable specificity for the α-adrenergic receptor. Thus, it is almost a pure vasopressor. Isoproterenol and dobutamine have little α-adrenergic agonist activity but have considerable activity at the β-receptor; they are mostly used as inotropes. Epinephrine and dopamine have both inotropic and vasopressor activity. At relatively low infusion rates, they enhance myocardial function and increase heart rate (β 1 and β 2 ). At higher rates, vasopressor activity (α 1 ) becomes manifest.
Dopamine
Basic pharmacology
In the enzymatic pathway leading from tyrosine to epinephrine (see Fig. 31.8 ), decarboxylation transforms l -dopa to dopamine. Dopamine is a central neurotransmitter found in sympathetic nerve terminals and in the adrenal medulla, where it is the immediate precursor of norepinephrine.
Clinical pharmacology
Dopamine stimulates dopamine (D 1 – and D 2 -) receptors located in the brain and in vascular beds in the kidney, mesentery, and coronary arteries. It also stimulates α- and β-receptors, although the compound’s affinity for these receptors is lower. D 1 -receptors are coupled to G s and thus enhance adenylate cyclase and produce a rise in cAMP, which evokes vasodilation. This increases blood flow to these organs and enhances renal solute and water excretion by the kidney. Dopamine modulates release of aldosterone and prolactin (via D 2 -receptors), which also may affect renal solute clearance.
Low infusion rates of dopamine augment renal sodium excretion; intermediate rates (5–10 μg/kg per minute) produce chronotropic and inotropic effects, and still higher infusion rates increase vascular resistance. Renal blood flow, glomerular filtration rate, and sodium excretion are maintained or even increase during dopamine infusion in patients with poor cardiac output.
Research into the dose-physiologic response relationships in patients treated with dopamine have yielded inconsistent results, suggesting that a patient’s response to a given dose depends on underlying condition, hemodynamics, and adrenergic receptor status, and highlights the importance of titration to individual response when using catecholamines in critically ill patients. Despite these observed variations in dose-response, there is now consensus that the augmentation in urine output seen in patients with poor cardiac output who are started on low-dose, “renal” dopamine results from improved renal blood flow rather than a direct, receptor-mediated diuretic effect on the kidney.
Both the receptor-mediated activity and clinical effects of dopamine depend in part on developmental factors such as age and, in the case of newborns, the degree of prematurity. However, trials in newborns have yielded conflicting results. Nevertheless, dopamine remains one of the most widely used vasoactive medications in the neonatal intensive care unit (ICU) amid ongoing controversies concerning the indications for pharmacologic intervention and specific therapeutic goals (i.e., optimal blood pressure vs. adequate organ perfusion) in this unique population. In addition, uncertainty persists regarding the long-term effects of dopamine on neurologic development in premature infants.
While there is limited evidence to support the observation that the inotropic and peripheral vasoconstrictor effects of dopamine predominate in the neonatal period, controversies remain regarding the vasodilator effects on the renal, coronary, and cerebral vascular beds. Much of the experimental evidence for diminished sensitivity to dopamine in infants emerged from studies in immature animals, and questions remain as to whether the results can be directly applied to humans. Perez and associates found that in critically ill neonates, infusion rates greater than 20 μg/kg per minute were needed to achieve cardiovascular stability. However, unlike in adults, reductions in urine output or peripheral perfusion were not observed. Bhatt-Mehta and coworkers, found that dopamine at doses as low as 0.5 to 1.0 μg/kg per minute increased cardiac output and stroke volume before heart rate, while increases in SVR did not occur until infusion rates exceeded 8 μg/kg per minute. Seri and colleagues attributed the increase in blood pressure that they observed in critically ill premature infants at doses of 2.5 to 7.5 μg/kg per minute to the enhanced α-adrenergic sensitivity of the immature myocardium, but reduced clearance of dopamine in this age group has also been proposed.
Dopamine crosses the blood-brain barrier in preterm neonates; in one study, Wong et al. demonstrated improved cerebral venous saturations and coupling of cerebral blood flow to cerebral metabolic rate of oxygen consumption.
Pharmacokinetics
Plasma dopamine clearance ranges from 60 to 80 mL/kg per minute in normal adults and is lower in patients with renal or hepatic disease. , In subjects with normal renal function, the elimination half-life of infused dopamine is approximately 2 minutes. Among critically ill children, the elimination half-life is 26 ± 14 minutes. In neonates, the elimination half-life is 5 to 11 minutes. , Wide interindividual variations in the rate of dopamine clearance have been reported in critically ill children as well as in healthy adults. , Age has a striking effect on clearance of dopamine; clearance in children younger than 2 years of age is approximately twice as rapid as it is in older children (82 vs. 46 mL/kg per minute). Allen and colleagues demonstrated that clearance of infused dopamine decreased by almost 50% during the first 20 months of life, while an additional 50% decrease occurs between the ages of 1 and 12 years. Another study did not show a correlation between advancing age and dopamine clearance, although the patients in this study had a mean age of 37 months. Dopamine clearance may also decrease after 24 hours of continuous infusion. Vernon and colleagues studied the pharmacokinetics of dopamine in 15 patients ranging in age from 3 days to 8 years and noted nonlinear behavior; the authors questioned the utility of evaluating total body clearance in this age group. Differences in the rate of sulfoconjugation as a route of elimination also may contribute to the wide variations in the clearance of dopamine in critically ill children. , , The effect of coadministration of dobutamine on dopamine clearance has been suggested by some authors. However, an in vitro study showed that, although dopamine and dobutamine both compete for COMT and MAO, the concentrations achieved under clinical conditions are unlikely to produce clinically significant levels of inhibition. , Pharmacokinetic differences between children and infants, rather than a difference in vascular or myocardial receptor sensitivity, may account for the observation that infants require and tolerate higher infusion rates.
Clinical role
Dopamine has been shown to be an effective inotropic and vasopressor agent in neonates and infants with a variety of conditions associated with circulatory failure. Fewer data evaluating the efficacy of dopamine in older children are available. Until recently, dopamine was used as a first-line treatment in children with fluid refractory septic shock or distributive shock, but updated guidelines for the management of septic shock now recommend norepinephrine or epinephrine as the agent of choice. Dopamine may be appropriate for children with mild impairment of myocardial function. , Severe impairment of vascular tone or cardiac contractility are indications for other agents, and children with primary myocardial disease not complicated by hypotension will benefit from a more selective inotropic agent such as milrinone or dobutamine. Infusion rates of dopamine necessary to improve signs of severe myocardial dysfunction may be associated with tachycardia, dysrhythmia, and increased myocardial oxygen consumption—these adverse effects often outweigh any potential benefit.
Adverse effects
The clinical signs of dopamine toxicity are mainly cardiovascular: tachycardia, hypertension, and dysrhythmia. However, dopamine is less likely to produce severe tachycardia or dysrhythmias than either epinephrine or isoproterenol. With the possible exception of the bipyridines, all inotropes increase myocardial oxygen consumption because they increase myocardial work. If the resulting increase in oxygen consumption is balanced by improved coronary blood flow, the net effect on oxygen balance is beneficial. The effect of dopamine on myocardial oxygen balance is better than that of isoproterenol but not as good as dobutamine and milrinone. In the setting of cardiogenic shock, the improvement in myocardial contractility with the addition of an inotrope may reduce preload and afterload, improve coronary perfusion pressure, increase myocardial oxygen supply, and prolong diastolic coronary perfusion by reducing heart rate. If the same drug is administered to a patient with normal myocardial contractility, the result may be an increase in cardiac oxygen consumption without an increase in oxygen delivery to the myocardium. Tachycardia, which can both increase oxygen consumption and shorten diastole, is a particular burden.
Dopamine depresses the ventilatory response to hypoxemia and hypercarbia by as much as 60%. β-Agonists, including dopamine, decrease partial pressure of arterial oxygen by interfering with hypoxic vasoconstriction. In one study, dopamine increased intrapulmonary shunting in patients with acute respiratory distress syndrome (ARDS) from 27% to 40%. The effect of dopamine on perfusion to the splanchnic bed is widely debated. Evidence suggests that in patients with sepsis, dopamine may increase splanchnic blood flow, gastric pH, and lead to less lactate production than epinephrine. Dopamine may also increase oxygen delivery and reduce oxygen consumption in septic patients. , On the other hand, experiments in animals suggest that dopamine is capable of modulating cellular immune functions in sepsis and may decrease survival. In a murine model, survival in dopamine-treated septic animals decreased by nearly 40%. An increase in splenocyte apoptosis and decrease in splenocyte proliferation and interleukin-2 (IL-2) release, both of which indicate attenuated immune system function, were also observed .
In infants and children who have undergone cardiac surgery, dopamine may have several endocrinologic effects, including decreases in prolactin and thyrotropin levels as well as a reduction in the pulsatility of growth hormone.
In patients with shock, dopamine can cause limb ischemia, gangrene of distal parts and entire extremities, and extensive loss of skin. This is owing to release of norepinephrine from synaptic terminals and in vivo conversion to norepinephrine. Hence, it is more often associated with limb ischemia than other adrenergic compounds. Extravasations of dopamine should be treated immediately by local infiltration of phentolamine (5–10 mg in 10 mL of normal saline solution) administered with a fine hypodermic needle.
Preparation and administration
Dopamine hydrochloride is available as premixed solutions for infusion. The use of standard concentrations and electronic “drip calculators” are encouraged to prevent dosing errors. Dopamine is administered by central vein to avoid the risk of skin injury from extravasation, although it can be given safely through a peripheral intravenous catheter while central access is being secured. Dopamine may also be administered via the intraosseous route. Dopamine is not compatible with some parenteral nutrition solutions or with sodium bicarbonate. Dopamine is stable in solution for 24 to 84 hours. ,
Interactions
Dopamine is metabolized by MAO, and concurrent use of an MAO inhibitor potentiates its effect. Both α-adrenergic blockers and β-adrenergic blockers antagonize the effects of dopamine. Other dopamine antagonists, such as metoclopramide or haloperidol, also may attenuate its effects. An increase in the infusion rate will often overcome the receptor blockade.
Summary
Dopamine has been used to treat mild to moderate cardiogenic or distributive (septic, hypoxic-ischemic) shock associated with hypotension. In the absence of hypotension, acute severe cardiac failure is best treated with dobutamine or milrinone. When septic or cardiogenic shock is complicated by severe hypotension, epinephrine or norepinephrine is preferred, depending on hemodynamics and myocardial function ( Table 31.2 ).
Blood Pressure or SVR | |||
---|---|---|---|
Hemodynamic Pattern | Normal | Decreased | Elevated |
Septic Shock | |||
Stroke index ↑ or ↔ | Norepinephrine | ||
Stroke index ↓ | Dobutamine or dopamine | Dopamine or epinephrine (or dobutamine and norepinephrine) | Dobutamine plus vasodilator and/or milrinone |
Cardiogenic shock | Dobutamine or dopamine or milrinone | Dopamine or epinephrine | Dobutamine plus vasodilator and/or milrinone |
Myocardial dysfunction a (complicating critical illness) | Dobutamine or dopamine or milrinone | Dopamine or epinephrine | Dobutamine plus vasodilator and/or milrinone |
Congestive heart failure | Dobutamine or dopamine or milrinone | Dobutamine plus vasodilator and/or milrinone | |
Bradycardia | Isoproterenol |
a For example, acute respiratory distress syndrome or anthracycline therapy.
Norepinephrine
Basic pharmacology
Dopamine is hydroxylated at the β-carbon to produce norepinephrine, the principal neurotransmitter of the sympathetic nervous system (see Figs. 31.7 and 31.8 ). Because there is no substituent on the N(amino)-terminus, norepinephrine has little β 2 activity and is considerably less potent at that receptor than epinephrine. It is a moderately potent α- and β 1 -agonist.
Clinical pharmacology
In normal subjects, norepinephrine elevates SVR because α-adrenergic stimulation is not opposed by β 2 stimulation. Reflex vagal activity reduces the rate of sinus node discharge, blunting the expected β 1 -chronotropic effect. In normal subjects renal, splanchnic, and hepatic blood flows decrease. The increase in afterload may augment coronary blood flow. This effect may be enhanced by α-adrenergic receptors located in the coronary arteries, although in coronary arteries from explanted human hearts, the vasodilation in response to norepinephrine was mediated via β 2 -receptors. Norepinephrine does have inotropic effects on the heart, mediated via α 1 – and β 1 -receptors. The degree of inotropic response related to α 1 stimulation may be affected by the pressure load on the right ventricle. In the failing heart, the relative contribution from each type of adrenergic receptor appears to be equal. In healthy volunteers, norepinephrine produces a decrease in creatinine clearance because of the effect on renal blood flow. However, in patients with hypotension, the improvement in global perfusion produces an increase in urine output.
The acute hemodynamic effects of norepinephrine are compared with those of epinephrine and isoproterenol in Fig. 31.9 . Experience in critically ill children indicates that the hemodynamic responses are not different from those observed in adults. Use of norepinephrine in 18 neonates with persistent pulmonary hypertension–associated heart dysfunction produced significant increases in systemic blood pressure and left ventricular output, along with improvement in postductal transcutaneous oxygen saturation, pulmonary-to-systemic blood pressure ratio, and an increase in the velocity of pulmonary artery blood flow. In a separate study in 22 neonates with septic shock refractory to fluid support and dopamine or dobutamine, norepinephrine significantly increased mean arterial blood pressure and urine output while decreasing blood lactic acid concentrations.

Pharmacokinetics
There is limited information about the pharmacokinetics of norepinephrine in children. Basal plasma levels of norepinephrine are much higher than basal plasma levels of epinephrine (250–500 vs. 20–60 pg/mL). The minimum concentration at which norepinephrine produces detectable hemodynamic activity is at least 1500 to 2000 pg/mL, suggesting that endogenous plasma norepinephrine simply represents “spillover” from sympathetic activity and that norepinephrine is not a true hormone. The clearance of norepinephrine in healthy adults is 24 to 40 mL/kg per minute, with the half-life averaging 2.0 to 2.5 minutes. The clinical effect of norepinephrine ceases within 2 minutes of the infusion being stopped. Norepinephrine is inactivated by reuptake into nerve terminals, with some elimination occurring by enzymatic degradation in the liver, adrenal glands, and kidney, either by methylation to normetanephrine (by COMT) or by oxidative deamination. 3-Methoxy-4-hydroxymandelic acid is the major metabolite in the urine.
Clinical role
Norepinephrine improves perfusion in children with distributive or septic shock who are hypotensive but in whom cardiac output is preserved or elevated. Norepinephrine is administered in conjunction with repletion of intravascular volume; titration is best guided by estimates of cardiac output and SVR. The experience in adult patients provides a rationale for using this agent to treat hypotension that is unresponsive to volume repletion —norepinephrine is now recommended for warm shock refractory to fluid loading in children. A randomized study (in adults) indicates that high-dose norepinephrine is superior to high-dose dopamine for treating hypotension associated with hyperdynamic septic shock. Coronary and renal blood flow increased in lambs at a dose of 0.4 μg/kg per minute while mesenteric blood flow decreased. Thus, titration is important and may entail rapid escalation of dosage. One of several studies in children with septic shock treated with norepinephrine suggested that higher doses might be needed in this population to restore adequate blood pressures and perfusion. A single-center retrospective review of 144 children with septic shock treated with norepinephrine between 2000 and 2010 reported a decrease in mortality from 82% to 17% over the study period and an associated increase in norepinephrine use. Mean doses ranged from 0.5 μg/kg per minute to 2.5 μg/kg per minute. The only complications observed were arrhythmias (not requiring treatment) in 2 patients and hypertension, which resolved with lowering of the norepinephrine dose. Notably, the authors reported no complications in 27 patients receiving the drug through a peripheral intravenous catheter for as long as 3 hours.
Norepinephrine is most valuable in the context of tachycardia because, unlike dopamine, at doses required to induce a vasopressor effect, norepinephrine does not elevate and may even lower heart rate through reflex mechanisms. Norepinephrine has also been shown to improve right ventricular performance in adults with hyperdynamic septic shock.
The usual starting dosage for an infusion is 0.05 μg/kg per minute ( Table 31.3 ), with a goal of providing adequate perfusion pressure. Arbitrary values of SVR or blood pressure are not useful end points for therapy. The lowest infusion rate that improves perfusion (skin color and temperature, mental status, urine flow, and reduction in lactate level) should be used.
Clinical Indication | ||
---|---|---|
Agent | Inotropic | Pressor |
Dopamine | 2–15 | >12 |
Epinephrine | 0.05–0.5 | 0.10–1 |
Norepinephrine | 0.05–1 | |
Vasopressin (U/kg/min) | 0.0005–0.002 b | |
Dobutamine | 2.5–20 | |
Milrinone | 0.25–0.75 | |
Isoproterenol | 0.05–1 |
a Vasopressin is dosed in units or mU/kg/min for shock.
b Optimal dosage and infusion rate have not been established in children.
Other causes of distributive shock (e.g., vasodilator ingestion and intoxication with CNS depressants) also respond to norepinephrine when the predominant hemodynamic problem is low SVR and blood pressure.
Adverse effects
The increase in afterload that norepinephrine produces can potentially increase myocardial oxygen consumption, but norepinephrine may reflexively decrease heart rate, reducing oxygen consumption and improving diastolic coronary perfusion. Norepinephrine may lead to compromised organ blood flow in the setting of hypovolemia and may elevate blood pressure without improving perfusion. Poor clinical response is usually associated with a low cardiac index, stroke volume, left ventricular stroke work index, and an elevated pulmonary artery occlusion pressure. , Employing excessive dosages or using norepinephrine to elevate blood pressure without improving perfusion may result in multiple-organ system failure.
Preparation and administration
Norepinephrine should be diluted in 5% dextrose or 0.9% sodium chloride. Norepinephrine is administered only by central venous catheter, except in extreme emergency. Extravasation of norepinephrine should be treated immediately by local infiltration of phentolamine administered with a fine hypodermic needle. , As with dopamine, norepinephrine should be administered by a device that permits controlled and precise titration.
Interactions
Tricyclic antidepressants potentiate the action of norepinephrine by reducing neuronal uptake of the compound. MAO inhibitors do not appear to enhance norepinephrine activity. α-Adrenergic blocking agents reduce efficacy of norepinephrine.
Summary
Norepinephrine is the agent of choice in patients with hypotension with low SVR and a normal or high cardiac output after fluid resuscitation (see Table 31.2 ). Recent septic shock guidelines recommend norepinephrine as the first-choice vasopressor in patients with warm (vasodilatory) shock. , It is frequently useful in other conditions associated with distributive shock.
Epinephrine
Basic pharmacology
Epinephrine is synthesized in the adrenal medulla, where it is formed from norepinephrine by addition of a methyl group to the N-terminus. The reaction is catalyzed by N-methyltransferase (see Fig. 31.8 ). Epinephrine is a hormone; endogenous levels change with the physiologic state of the organism via afferent input to the adrenal medulla. Resting levels are less than 50 pg/mL; heavy exercise produces concentrations of 400 pg/mL or greater. Epinephrine activates α-, β 1 -, and β 2 -receptors. It is a principal hormone of stress and produces widespread metabolic and hemodynamic effects.
Clinical pharmacology
Epinephrine activates β 1 -receptors in the myocardium and conducting systems at low concentrations, which accelerates phase 4 of the action potential. The heart rate increases, and systolic time intervals are shortened. The inotropic state of the myocardium is also enhanced, producing an increase in force of contraction. Evidence indicates that changes in myocardial oxygen consumption are disproportionate to the increase in force of contraction, thereby decreasing myocardial efficiency. High concentrations of epinephrine or exposure to the compound when the myocardium is sensitized by infarction, operation, or myocarditis may produce serious atrial and ventricular dysrhythmias.
At low plasma concentrations, stimulation of peripheral β 2 -receptors promotes relaxation of resistance arterioles; SVR decreases and diastolic blood pressure falls (see Fig. 31.9 ). The decrease in SVR enhances the direct chronotropic effect of epinephrine. Higher concentrations are associated with activation of vascular α-receptors, and SVR increases. The effect of epinephrine on pulmonary vasculature may also vary with the dosage used. Higher doses are associated with an increase in pulmonary vascular resistance (PVR), both from a direct effect and as a result of increased venous return to the right side of the heart. During infusion of epinephrine, hepatic and splanchnic blood flows increase, while renal blood flow may be reduced.
The thresholds for producing these effects in healthy adults have been examined. Normal basal levels are around 40 pg/mL. As levels increase, the heart rate accelerates first, followed by increases in systolic blood pressure and decreases in diastolic blood pressure. Various metabolic effects (hyperglycemia, cytogenesis, and glycolysis), hypophosphatemia, and hypokalemia may also occur at higher levels. Desensitization to epinephrine occurs rapidly and may be present prior to administration of exogenous catecholamines in the ICU.
Pharmacokinetics
In healthy male volunteers, the plasma clearance of epinephrine is 35 to 89 mL/kg per minute. , The elimination half-life is approximately 1 minute. Epinephrine is methylated by COMT to metanephrine in the liver and kidneys or deaminated via the action of MAO. It also may be metabolized by extraneuronal uptake. The resulting catabolites are then conjugated to sulfate or glucuronide and excreted in the urine. A wide interindividual variation in clearance is observed in healthy adults. In critically ill children receiving epinephrine at doses from 0.03 to 0.2 μg/kg per minute, plasma concentrations at steady state were linearly related to dose. In this study, wide interindividual variation in clearance was observed.
Clinical role
Epinephrine is used to treat shock and low cardiac output states associated with myocardial dysfunction. Thus, it is appropriate for treatment of cardiogenic shock or for inotropic support following cardiac surgery. In a model of right ventricular injury, epinephrine increased pulmonary artery blood flow and right ventricular power with greater efficiency than did dopamine or dobutamine. It can also be used to increase pulmonary flow across left to right shunts. Epinephrine is most likely to be useful in patients with sepsis and “cold shock,” that is, in the setting of poor perfusion and low cardiac index that does not respond to fluid resuscitation. , At modest infusion rates (0.05–0.10 μg/kg per minute), SVR decreases slightly; heart rate, cardiac output, and systolic blood pressure increase. At intermediate infusion rates, α 1 -adrenergic activation becomes important but is balanced by the improved cardiac output and activation of vascular β 2 -receptors. Although epinephrine constricts renal and cutaneous arterioles, renal function and skin perfusion may improve. Very high infusion rates (>1–2 μg/kg per minute) are associated with significant α 1 -adrenergic-mediated vasoconstriction; blood flow to individual organs will be compromised and the associated increase in afterload may further impair myocardial function. Studies have shown decreased splanchnic blood flow, decreased oxygen uptake, and increased lactate with epinephrine compared with norepinephrine despite similar increases in global oxygen delivery. Dopamine led to a decrease in lactate and an increase in arterial pH, whereas epinephrine was associated with increases in lactate and metabolic acidosis despite similar increases in cardiac index and oxygen delivery. In newborn piglets, high-dose epinephrine increased SVR, PVR, and lactate while decreasing hepatic blood flow and oxygen delivery. Other studies have shown that the degree of shock also may influence splanchnic blood flow. In a study of adult patients with septic shock, stepwise increases in epinephrine were associated with linear increases in cardiac rate, mean arterial pressure, cardiac index, left ventricular stroke work index, oxygen consumption, and oxygen delivery. Neither PVR nor SVR was affected. Epinephrine is first-line treatment for severe anaphylaxis in both the prehospital and hospital settings, and the case fatality rate from food-related anaphylaxis has declined since the introduction of the epinephrine autoinjector.
Epinephrine has been evaluated in very-low-birth-weight infants with hypotension who did not respond to dopamine at doses as high as 15 μ/kg per minute. Blood pressure and heart rate increased while urine output was maintained. Urine output increased among infants who had been oliguric.
Epinephrine is the most frequently used medication during pediatric cardiopulmonary resuscitation. Bolus injections of epinephrine are used to treat hemodynamically significant bradycardia, asystole, and pulseless arrest. Earlier studies in animals , demonstrated improved survival after primary cardiac arrest in subjects treated with epinephrine. This was attributed to improved coronary (and therefore myocardial) perfusion from an increase in aortic diastolic pressure. A study in adults following cardiopulmonary arrest attributed to ventricular fibrillation showed that early use of epinephrine was associated with improved survival to hospital discharge, while later initiation of the drug was not. , The recommended initial dosage is 0.01 mg/kg (10 μg/kg or 0.1 mL/kg of the 1:10,000 solution).
Epinephrine may be given by endotracheal tube; the dosage is 100 μg/kg. Intraosseous administration is appropriate for both bolus and continuous administration of epinephrine. The dosage is the same as for intravenous injection. Epinephrine infusion is also the agent of choice for hypotension following successful treatment of cardiac arrest and, in most cases, of primary cardiogenic shock.
Preparation and administration
Epinephrine for injection at a concentration of 1:10,000 may be administered undiluted. The 1:1000 injection must be diluted with 0.9% sodium chloride prior to administration. Epinephrine should be infused by a pump capable of precise titration into a central vein, although low-dose infusions may be administered safely via peripheral intravenous catheters if central venous access is not available.
Adverse effects
Epinephrine produces CNS excitation manifested as anxiety, dread, nausea, and dyspnea. Enhanced automaticity and increased oxygen consumption are the main cardiac toxicities. Extreme tachycardia carries a substantial oxygen penalty, as does hypertension. A severe imbalance of myocardial oxygen delivery and oxygen consumption produces characteristic electrocardiogram changes of ischemia. A subischemic but persistently unfavorable ratio of oxygen delivery to consumption also may be harmful to the myocardium. Epinephrine may be arrhythmogenic. Increases in infusion rate lead to successively more serious events, including atrial and ventricular extrasystoles, atrial and ventricular tachycardia, and, ultimately, ventricular fibrillation. Ventricular dysrhythmias in the pediatric age group are not common but may occur in the presence of myocarditis, hypokalemia, or hypoxemia. Hypokalemia during infusion of epinephrine results from stimulation of β 2 -adrenergic receptors, which are linked to Na + /K + -ATPase located in skeletal muscle. Hyperglycemia results from β-adrenergic-mediated suppression of insulin release. Increases in blood lactate levels have also been observed. Epinephrine infiltration into local tissues or intraarterial injection can produce severe vasospasm and tissue injury but with less frequency than with norepinephrine, dopamine, or vasopressin.
Epinephrine overdose can be fatal. Several neonates died when inadvertently subjected to oral administration of huge amounts of epinephrine. The syndrome mimicked an epidemic of neonatal sepsis with shock and metabolic acidosis. Intraaortic injection in infants (per umbilical artery) produces tachycardia, hypertension, and renal failure. Intravenous overdose of epinephrine may cause myocardial infarction, ventricular tachycardia, extreme hypertension (up to 400/300 mm Hg), cerebral hemorrhage, seizures, renal failure, and pulmonary edema. Bradycardia also has been observed. Manifestations of acute overdose are treated symptomatically. β-Receptor antagonists such as propranolol are contraindicated (see later discussion). Hypertension is treated with short-acting antihypertensives (e.g., nitroprusside).
Interactions
Tricyclic antidepressants and antihistamines such as diphenhydramine may potentiate the effects of epinephrine; use of fluorinated anesthetic agents such as halothane may increase the frequency of ventricular dysrhythmia. , Administration of epinephrine with a β-adrenergic antagonist such as propranolol may be dangerous because of residual unopposed α 1 activity. The result can be severe hypertension and bradycardia terminating in asystole. The concomitant use of α- or β-adrenergic antagonists also may antagonize the therapeutic effects of epinephrine.
Summary
Epinephrine is useful in treating shock and low cardiac output states associated with myocardial dysfunction. In critically ill pediatric patients, the most frequent indications for epinephrine infusion are cardiogenic shock, septic shock associated with hypotension and reduced stroke volume, and shock following severe hypoxemia-ischemia (see Table 31.2 ).
Isoproterenol
Basic pharmacology
Isoproterenol is the synthetic N-isopropyl derivative of norepinephrine (see Fig. 31.7 ). The bulky N-terminal substituent confers β 1 – and β 2 -receptor specificity; the compound does not affect the α-adrenergic receptor. Thus, the principal cardiovascular activities of isoproterenol relate to its inotropic, chronotropic, and peripheral vasodilator effects.
Clinical pharmacology
Isoproterenol enhances cardiac contractility and heart rate. Peripheral vasodilation produces a fall in SVR, augmenting the direct chronotropic action of the drug. Significant tachycardia ensues. Systolic blood pressure increases while mean and diastolic pressures fall (see Fig. 31.9 ). Infusion of isoproterenol decreases mesenteric and renal perfusion in healthy patients. However, the increase in cardiac output associated with isoproterenol administration in patients with shock may increase blood flow to these tissues. Isoproterenol increases myocardial demand for oxygen and decreases supply by reducing diastolic coronary filling. Hypotension may complicate initiation of isoproterenol infusion in volume-depleted patients.
Activation of β 2 -adrenergic receptors produces bronchodilation and pulmonary vasodilation, respectively. For this reason, isoproterenol by continuous intravenous infusion was employed in the past as adjunctive therapy in children with refractory or rapidly worsening status asthmaticus. Continuously nebulized albuterol and intravenous terbutaline have supplanted isoproterenol for this indication.
Hyperglycemia is not usually observed in patients receiving isoproterenol, although the drug does promote release of free fatty acids. Isoproterenol produces an increase in plasma norepinephrine levels; however, this effect relative to the hemodynamic response to isoproterenol has not been studied.
Pharmacokinetics
Isoproterenol is metabolized by COMT. The plasma elimination half-life of isoproterenol is 1.5 to 4.2 minutes. , Information about therapeutic isoproterenol concentrations in critically ill patients is not available.
Clinical role
In the past, isoproterenol was used for a variety of indications, including septic shock and cardiogenic shock associated with myocardial infarction. However, the tachycardia and increased myocardial oxygen consumption, as well as a more sophisticated understanding of the pathophysiology of shock, have limited the use of this compound to only a few specific indications.
Isoproterenol may be used to treat hemodynamically significant bradycardia. However, epinephrine is often preferable. When bradycardia results from heart block, isoproterenol may be used in the acute setting as a bridge to pacemaker placement.
Preparation and administration
Isoproterenol should be diluted prior to administration.
Adverse effects
Adverse effects associated with isoproterenol include fear, anxiety, restlessness, insomnia, and blurred vision. Other effects may include headache, dizziness, tinnitus, sweating, flushing, pallor, tremor, nausea, vomiting, and asthenia. Cardiovascular effects may include ventricular tachycardia and other life-threatening ventricular dysrhythmias. Isoproterenol may cause hypertension or severe hypotension.
Interactions
The concomitant administration of a halogenated general anesthetic such as halothane or an intravenous methylxanthine such as aminophylline may potentiate the adverse cardiovascular effects of isoproterenol.
Summary
Isoproterenol is rarely used to treat children or adults. More selective β 2 -agonists are safer to use and are preferred. The main indication in the acute setting is for the treatment of symptomatic bradycardia.
Dobutamine
Basic pharmacology
The structure of dobutamine, a synthetic catecholamine, resembles dopamine in that the β carbon is not hydroxylated. Unlike other catecholamines, there is a large aromatic substituent on the N-terminus. Like isoproterenol, dobutamine is administered as a racemate; (+) dobutamine is a strong β-agonist and an α-antagonist, and (–) dobutamine is an α-agonist and a weak β-agonist. This blend of receptor activities allows dobutamine to deliver significant inotropic and usually trivial chronotropic and vasopressor activity.
Clinical pharmacology
In adults with CHF, dobutamine increased cardiac index, decreased left ventricular end-diastolic volume, and increased the left ventricular dP/dt (derivative of pressure over time; used as a measure of contractility). Although renal function and urine output may improve as the increase in cardiac output fosters relaxation of sympathetic tone and improved perfusion, dobutamine did not improve indices of renal function compared with dopamine in critically ill patients. Dobutamine improved right ventricular systolic function and decreased PVR in piglets with right ventricular injury. In healthy children, dobutamine increased left ventricular systolic function and relaxation. In the newborn piglet, dobutamine increased superior mesenteric and renal artery blood flow, increased cardiac index, and decreased SVR. A threshold model with a log-linear dose-response relationship above the threshold has been demonstrated in critically ill term and preterm neonates and in children between 2 months and 14 years of age. , In one small study, dobutamine infusion was associated with increases in cardiac output, blood pressure, and heart rate. Dobutamine is a relatively selective inotrope with little effect on heart rate at usual infusion rates. Somewhat greater thresholds for improved cardiac output were observed in a second group of children and in infants. However, in all studies, dobutamine improved cardiac contractility without substantially altering heart rate unless high infusion rates were employed. , In a Cochrane analysis that compared dobutamine with dopamine in premature neonates with low systemic blood flow on the first day of life, dobutamine produced a significantly greater increase in superior vena cava flow whereas dopamine produced a significantly greater increase in mean blood pressure. In addition, dobutamine has been shown to increase cerebral blood flow velocity but not cerebral oxygen consumption in patients with septic shock.
Pharmacokinetics
The plasma elimination half-life of dobutamine in adults is approximately 2 minutes. CHF increases the volume of distribution. Reported clearance values in children have ranged from 32 to 625 mL/kg per minute in one study and from 40 to 130 mL/kg per minute in another. , The principal route of elimination is methylation by COMT, followed by hepatic glucuronidation and excretion into urine and bile. 3-O-Methyldobutamine also represents a major route of elimination for dobutamine, with up to 33% of the infused drug being eliminated as the sulfoconjugated compound. Dobutamine also is cleared from the plasma by nonneuronal uptake. Some investigators have reported nonlinear elimination kinetics, but other data suggest that dobutamine’s kinetics can be adequately described by a simple first-order (linear) model. , , , ,
Clinical role
In adults, dobutamine produces improvement in a variety of conditions associated with poor myocardial performance, such as cardiomyopathy, atherosclerotic heart disease, and acute myocardial infarction. Dobutamine has been used following surgery for myocardial revascularization, cardiac transplantation, and other procedures associated with postoperative myocardial dysfunction, although undesirable chronotropic effects have also been reported. Dobutamine is not first-line therapy for septic shock unless the primary disturbance is complicated by myocardial dysfunction. Although impaired myocardial performance can be demonstrated early in patients with septic shock, the main problem relates to regulation of vascular tone, and agents that increase SVR are preferred. When ventricular dysfunction complicates clinical management, dobutamine may be a useful adjunct. In this context, dobutamine alone or in combination with dopamine has produced an increase in cardiac output, left ventricular stroke work, and blood pressure. As indicated in Table 31.2 , dobutamine can be combined with norepinephrine in treating the patient with myocardial dysfunction associated with hyperdynamic shock (i.e., a child with septic shock who has received cardiotoxic chemotherapy).
Several studies in infants and children demonstrate that dobutamine improves myocardial function in a variety of settings. , , Stroke volume and cardiac index improve without a substantial increase in heart rate. SVR and PVR may decrease toward normal. Dobutamine has been evaluated in children following cardiac surgery with cardiopulmonary bypass. In a study by Bohn and colleagues, dobutamine enhanced cardiac output by increasing heart rate, while tachycardia prompted discontinuation of the infusion in several patients. The expected fall in SVR was not observed in children who received the drug after cardiopulmonary bypass. These differences in effectiveness between adults and children following be because myocardial dysfunction and CHF are generally not present in children undergoing repair of congenital heart disease. Rather, the indication for surgery involves abnormalities in cardiac architecture or circulatory anatomy. Berner and associates found that children undergoing mitral valve surgery responded to dobutamine with an increase in stroke volume, whereas children following tetralogy of Fallot repair did not, and their cardiac output increased only through a higher heart rate. A more recent report by the same group indicated that following repair of tetralogy of Fallot, dobutamine did enhance cardiac output when it was combined with atrial pacing to increase heart rate. Isoproterenol without pacing provided a higher cardiac output than either dobutamine alone or dobutamine in combination with pacing. Specific indications for dobutamine in the pediatric age group include low-output CHF and a normal to moderately decreased blood pressure (see Table 31.2 ). Typical examples include viral myocarditis, anthracycline-associated cardiomyopathy, cyclophosphamide, hemochromatosis (related to hypertransfusion therapy), or myocardial infarction (Kawasaki disease).
Dobutamine is not a first-line agent to treat low-output states that are caused by intracardiac shunt or abnormal cardiac chamber structure. Although dobutamine may be used following corrective or palliative cardiovascular surgery in the child in the context of demonstrated or suspected myocardial dysfunction, milrinone is now the preferred agent for providing inotropy and afterload reduction, despite insufficient evidence of superior effectiveness in a recent meta-analysis. Dobutamine may be of value as adjunctive therapy in treating myocardial dysfunction that complicates ARDS, septic shock, or a hypoxic-ischemic event, but epinephrine is generally preferred.
Preparation and administration
Dobutamine is available as a premixed solution in a variety of concentrations. Therapeutic dosing ranges between 2.5 and 20.0 μg/kg per minute. However, epinephrine is now generally employed in children requiring doses that exceed 10 μg/kg per minute.
Adverse effects
Adverse cardiovascular effects include hypertension, tachycardia, and ectopic heart beats. Headache, nausea, vomiting, paresthesia, and dyspnea may also occur. Dobutamine also may decrease serum potassium concentrations.
Dobutamine usually increases myocardial oxygen demand. In subjects with myocardial dysfunction, coronary blood flow and oxygen supply improve with the increase in demand. However, if dobutamine is used when myocardial contractility is normal, oxygen balance will be adversely affected. Tachycardia greatly increases myocardial oxygen consumption and should prompt a reduction in the dosage or use of an alternate agent.
Although dobutamine is less likely than other catecholamines to induce serious atrial and ventricular dysrhythmias, these may occur, particularly in the context of myocarditis, electrolyte imbalance, or high infusion rates. Dobutamine and other inotropes should be administered cautiously to patients with dynamic left ventricular outflow obstruction, as in hypertrophic obstructive cardiomyopathy. Prolonged infusion of dobutamine may inhibit platelet aggregation and, in some adult patients, petechial bleeding has been attributed to dobutamine.
Interactions
The concomitant use of a β-adrenergic antagonist such as propranolol may antagonize the cardiovascular actions of dobutamine. Halogenated anesthetic agents, such as halothane, may potentiate the adverse cardiovascular effects of dobutamine. Dobutamine may increase the insulin requirement in diabetic patients.
Summary
Dobutamine is a positive inotropic agent that may be used to treat poor myocardial contractility. For septic shock and other acute hemodynamic disturbances, dobutamine is an adjunct when the primary problem is complicated by poor myocardial function (see Table 31.2 ). In this context, concomitant use of a vasopressor such as norepinephrine may be appropriate.
Vasopressin
Basic pharmacology
Vasopressin is a highly conserved hormone, and vasopressin-like peptides are present in numerous species. Its main function is to preserve fluid balance in the organism. In humans, it is released in response to two main stimuli: increases in plasma osmolality and decreases in effective circulating volume or blood pressure. Although vasopressin has long been used for the treatment of diabetes insipidus, its name derives from its vasopressor effect. Vasopressin also acts as a neurotransmitter in the CNS, has a role regulating adrenocorticotropin hormone release, and is involved in thermoregulation, platelet aggregation, and smooth muscle contraction in the uterus and gastrointestinal tract. ,
Clinical pharmacology
The response patterns differ for the two stimuli for vasopressin release. An increase in plasma osmolality above 280 mOsm/kg leads to a dramatic increase in the release of vasopressin from the posterior pituitary. The hormone exerts its effect by increasing water reabsorption in the renal collecting duct. The dose-response curve is so steep that when osmolality is 290 mOsm/kg, vasopressin levels exceed those that produce maximal urinary concentration. In contrast, the threshold for release in response to hypovolemia or hypotension is much higher, with decreases of greater than 20% of the circulating volume required. However, once the threshold is reached, plasma levels rise twentyfold to thirtyfold (far exceeding levels seen with hyperosmolality). Vasopressin exerts its hemodynamic effects via the V 1a -receptor, which is coupled to G q . In the peripheral vasculature, intracellular calcium is increased, enhancing contraction and restoring systemic vascular tone. Vasopressin also inhibits potassium channels, further increasing intracellular calcium. , Baroreceptors in the left atrium, left ventricle, and pulmonary veins sense changes in volume while baroreceptors in the carotid sinus and aorta sense changes in arterial pressure. Decreased pressure leads to a reduced rate of firing and release of the tonic inhibition of vasopressin release.
Vasopressin is a potent vasoconstrictor when present in the plasma at high concentrations. At the lower concentrations associated with the vasopressin response to hyperosmolality, it actually induces vasodilation in the pulmonary, renal, and cerebral circulation via the V 2 -receptor or oxytocin-mediated nitric oxide release. It does not elevate blood pressure because an associated decrease in heart rate offsets the increase in SVR. For this reason, vasopressin was not originally considered to be a clinically useful agent to treat hypotension. Landry and colleagues measured plasma vasopressin levels in patients with shock who were receiving catecholamine support. Surprisingly, plasma levels of vasopressin were not elevated in patients with septic shock as compared with those with cardiogenic shock, whose plasma levels were nearly 10 times greater. Vasopressin infusion in patients with septic shock who were receiving catecholamines produced an increase in SVR and mean arterial pressure and a decrease in cardiac index. Plasma vasopressin levels are inappropriately low in patients with vasodilatory septic shock, possibly due to impaired baroreflex-mediated secretion. The authors hypothesized that this phenomenon contributes to the hypotension of vasodilatory septic shock.
It appears that in the early stages of septic shock, vasopressin levels are higher than normal but decrease to relatively low levels as shock persists. This pattern has also been demonstrated in a model of hemorrhagic shock in which neurohypophysis stores of vasopressin were depleted. In three patients with septic shock and low levels of vasopressin, the high-intensity signal from the posterior pituitary on T1-weighted magnetic resonance imaging was lost, suggesting depletion of vasopressin. Hence, vasopressin deficiency may occur early in vasodilatory shock and contribute to its pathogenesis.
Pharmacokinetics
Vasopressin circulates as a free peptide and does not exhibit any protein binding. It is degraded rapidly in the kidneys and liver, with 5% to 15% of an intravenous dose eliminated unchanged in the urine. The normal elimination half-life is 10 to 20 minutes. Renal failure or hepatic insufficiency can prolong the elimination half-life. ,
Clinical role
The original report by Landry and colleagues generated intense investigation into the clinical applications of vasopressin in the setting of vasodilatory shock. The same group prospectively evaluated vasopressin in patients with vasodilatory shock after placement of a left ventricular assist device. At a dose of 0.1 U/min, vasopressin increased mean arterial pressure and SVR but not cardiac index. Among patients with a high level of endogenous vasopressin, the increase in blood pressure tended to be less. A rapid response to vasopressin was noted in all patients, allowing for the dose to be decreased to as low as 0.01 U/min. This group also published experience with vasopressin in children after cardiac surgery. , Vasopressin was used to treat 11 children with hypotension on epinephrine infusions following cardiac surgery. At vasopressin doses ranging from 0.0003 to 0.002 U/kg per minute, blood pressure increased within 1 hour and the epinephrine infusion could be decreased in 5 of 8 patients. Two patients who had echocardiographic evidence of poor function died. The remaining nine patients with vasodilatory shock survived and were discharged from the ICU. The authors cautioned against the use of vasopressin in patients with cardiogenic shock in view of the potential effect on cardiac index. Vasopressin levels were measured in three patients; two had an absolute deficiency and one had a relative deficiency. In adults, vasopressin deficiency (relative or absolute) was associated with shock following cardiopulmonary bypass. Hemodynamic function improved with vasopressin, and the need for other vasopressors decreased. In a prospective, randomized study, the combination of vasopressin at a dose of 0.06 U/min and norepinephrine was compared with norepinephrine alone in patients with catecholamine-resistant vasodilatory shock. The patients in the vasopressin-norepinephrine arm had a lower heart rate and higher blood pressure, SVR, and cardiac index. They also had reduced requirements for norepinephrine and a lower rate of new-onset dysrhythmias. Gastric perfusion also was better preserved in the vasopressin group.
In summary, in studies of patients with vasodilatory shock, vasopressin has been shown to improve blood pressure, increase SVR, lessen the need for catecholamines, improve markers of myocardial ischemia, and improve urine output. , Published experience in pediatric patients with septic shock or following cardiac surgery is still limited. Liedel and colleagues published their experience with 5 patients, ranging in age from 2 weeks (a 23-week premature infant) to 14 years. In 4 patients, blood pressure increased and catecholamine support could be decreased. In three patients, urine output improved. In a multicenter, randomized trial involving 69 pediatric patients with vasodilatory shock, vasopressin (0.0005–0.002 U/kg per minute) or placebo was added to open-label vasoactive agents. There was no difference in the primary end point of time to vasoactive-free hemodynamic stability or in any of the secondary outcomes, which included mortality, ventilator-free days, length of critical care unit stay, and adverse events. Ten deaths occurred in the vasopressin group and five in the placebo group (no statistical significance). It was concluded that low-dose vasopressin did not demonstrate any added benefit. Vasopressin also has been used in children undergoing evaluation for brain death. At a dose of 0.04 U/kg per hour, blood pressure increased and α-agonist support was decreased. No deleterious effect on organ function was noted.
Prior to the 2015 guidelines, vasopressin was added to the Advanced Cardiac Life Support protocol for ventricular fibrillation in adults. However, there is insufficient evidence to make a recommendation either for or against its use in children who sustain a cardiac arrest. , Mann and colleagues published their experience with vasopressin during cardiopulmonary resuscitation in pediatric patients in a retrospective case series. In six events involving four patients, vasopressin was given after conventional therapy had failed to achieve return of spontaneous circulation (ROSC). In all six events, pulseless electrical activity was the initial rhythm, while at the time vasopressin was given, events were asystole, pulseless ventricular tachycardia, and ventricular fibrillation. In four cases, ROSC was achieved for more than 60 minutes and one patient survived to discharge in a condition close to her neurologic baseline. A review of the American Heart Association National Registry of Cardiopulmonary Resuscitation in children suggested a lower rate of ROSC and longer arrest duration in patients who received vasopressin during in-hospital resuscitation. The authors emphasize that this result should be interpreted with caution, however, because vasopressin was only administered in 64 (5%) of the 1293 cases reviewed, and all of these patients had longer arrest times and were also pretreated with epinephrine.
Dosing and administration
No standards for pediatric dosing currently exist. The American Heart Association guidelines for pediatric advanced life support suggest a bolus dose of 0.5 U/kg. Standard dosing for vasopressin in vasodilatory shock has not been determined, but the dose used in a recent pediatric study ranged from 0.0005 to 0.002 U/kg per minute. The current guidelines for the management of severe sepsis and septic shock in adults suggest that vasopressin (0.03 U/min) may be added to norepinephrine to raise mean arterial pressure to target or to decrease norepinephrine dose but that it should not be used as the initial vasopressor.
Adverse effects
Few adverse events have been reported with the use of vasopressin in the setting of vasodilatory shock. Elevation of liver enzymes and total bilirubin, with a decrease in platelet count, have been noted, and one series in adults noted 6 cardiac arrests among 50 patients receiving vasopressin for hemodynamic support. , All six patients had “severe refractory shock” and five were receiving a vasopressin dose greater than 0.05 U/min. In 30% of patients receiving vasopressin, ischemic skin lesions of the distal limbs, trunk, or tongue were noted. Preexisting peripheral arterial occlusive disease and the presence of septic shock were identified as risk factors. Extravasation of vasopressin from a peripheral intravenous catheter was associated with skin necrosis. Treatment with vasopressin at doses used to augment blood pressures and improve hemodynamics may cause hyponatremia that, in most cases, resolves with discontinuation of the drug. Yet, in a case series involving 10 neonates with severe persistent pulmonary hypertension of the newborn who were treated with vasopressin, no significant decrease in serum sodium was observed. The effect on sodium level may be a function of patient selection, dose, and duration of treatment.
Interactions
The antidiuretic effect of vasopressin may be antagonized with the concomitant administration of epinephrine, heparin, lithium, or demeclocycline. The tricyclic agents chlorpropamide, carbamazepine, clofibrate, phenformin, and fludrocortisone may exert additive antidiuretic effects when used in combination with vasopressin. Concomitant use of vasopressin with a ganglionic blocking agent can enhance the vasopressor effect of vasopressin.
Summary
Vasopressin has been added to the pediatric intensive care unit (PICU) practitioner’s armamentarium for the treatment of decreased SVR. Its use may elevate blood pressure and urine output and, although it is not recommended as routine, first-line treatment, a low-dose infusion may be considered as rescue therapy in patients with catecholamine and steroid refractory vasodilatory shock. Its major advantage is in the lack of dependence on adrenergic receptors, which are known to be downregulated in septic shock. Studies to date in adults and children have not shown a benefit in reducing mortality or in decreasing intensive care mortality or ICU length of stay. Vasopressin should not be used in settings in which impaired myocardial function is the principal problem. Thus far, there is insufficient evidence to make a recommendation for or against the routine use of vasopressin in children during cardiopulmonary resuscitation from cardiac arrest.
Bipyridines
Inamrinone (formerly known as amrinone), milrinone, enoximone, and piroximone are nonsympathomimetic inotropic agents. The structure of milrinone is shown in Fig. 31.10 . Inamrinone, the earliest formulation to be introduced, was associated with an increased risk of thrombocytopenia in both adults and children , and potentially fatal hypotension. Thus, it is no longer available in the United States. Neither of these complications has been observed with milrinone, the current bipyridine of choice.
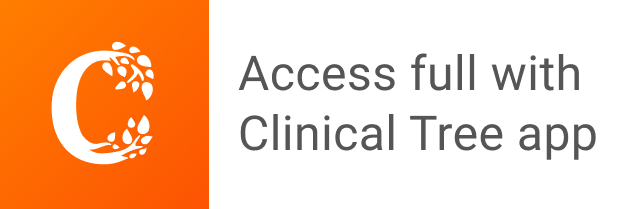