Chapter 6
Pharmacokinetics
Properties that Influence Pharmacokinetic Activity
The smaller the molecular size of an agent, the better it crosses the lipid barriers and membranes of tissues. When a drug is administered, it must be absorbed across biologic membranes that have very small openings or pores. Generally, molecules with molecular weights greater than 100 to 200 do not cross the cell membranes. Transport across the membranes can occur passively or actively. Passive transport does not require energy and involves transfer of a drug from an area of high concentration to an area of lower concentration. Active transport mechanisms are generally faster and require energy. This transport system uses carriers that form complexes with drug molecules on the membrane surface and can involve movement of the drug molecule against a concentration gradient from an area of low concentration to an area of high concentration.1 Figure 6-1 depicts the movement of a drug across cell membranes.
Drug Transporters
Many cell membranes possess specialized transport mechanisms that control entry and exit molecules, such as sugars, amino acids, neurotransmitters, and metal ions. They are broadly divided into solute carrier (SLC) transporters and adenosine triphosphate (ATP)–binding cassette (ABC) transporters. The SLCs control passive movement of solutes down their electrochemical gradient. The ABCs are active pumps requiring energy derived from adenosine triphosphate. Over 300 human genes are believed to code these transporters, most of which act on endogenous substrates; however, some drugs are also transported. Other SLCs are coupled to ATP-dependent ion pumps and transport can occur against an electrochemical gradient. It may involve exchange of one molecule for another called antiport or transport of two molecules together in the same direction referred to as symport. The main sites where SLCs, including organic cation transporters (OCTs) and organic anion transporters (OATs), are important are the blood-brain barrier, gastrointestinal tract, renal tubule, biliary tract, and placenta. Drug transporters are recognized as key players in the pharmacokinetic processes. Transporter proteins have a unique gatekeeper function in controlling drug access to metabolizing enzymes and excretory pathways. This can affect bioavailability, clearance, volume of distribution, and half-life for orally dosed drugs. A classification model referred to as the Biopharmaceutics Drug Disposition Classification System (BDDCS) categorizes drug transporter effects.2,3
Degree of Ionization and Lipid Solubility
Most drugs are salts of either weak acids or weak bases. When introduced into the body, they behave as a chemical in solution. As acids or bases they exist in solutions in both ionized and nonionized forms. The charged (ionized) form is water soluble, and the uncharged (nonionized) form is lipophilic. Because the nonionized molecules are lipid soluble, they can diffuse across cell membranes such as the blood-brain, gastric, and placental barriers to reach the effect site. On the other hand, the ionized molecules are usually unable to penetrate lipid cell membranes easily because of their low lipid solubility. This results from the electric charges exerted by the ionized drug molecules. These charged drugs are repelled by those sections of the cell membranes with similar charges, preventing their diffusion across the membrane.1 The higher the degree of ionization, the less access the drug has across tissues such as the gastrointestinal tract, the blood-brain and placental barriers, and liver hepatocytes. This is important in that ionized drugs are not absorbed well when taken orally and may not be metabolized by the liver to a significant extent. Instead, they commonly are excreted via the renal system.4
The second equation applies to acidic drugs:
From these equations an estimate of the degree of drug absorption can be developed.4,5 Acids are usually defined as proton donors, whereas bases are made up of molecules that can accept a proton. When the pH is equal to the pKa, the two species exist in equal amounts; for example, because phenobarbital has a pKa of 7.4 and blood has a pH of 7.4, in the bloodstream the drug is present in equal proportions of charged and uncharged forms.
It is of importance to note that relatively modest changes in the pH of the environment, when it is close to the pKa, are more significant in changing the ratio of charged to uncharged forms than the same change in pH at some value far removed from the pKa. For example, phenobarbital, with a pKa of 7.4, is for the most part nondissociated and therefore is nonionized at a pH of 1.4. This results from the fact that the pH is well below the pKa, and when phenobarbital is in a relatively strongly acidic environment with an abundance of protons, it does not give up its protons readily. If a drug is a weak acid and if the pH of the fluid environment is below the pKa, most of the drug’s protons are associated with the drug molecule, and the predominant species is uncharged and therefore lipid soluble (Figure 6-2). Conversely, if the pH is below the pKa for a drug that is a weak base, an abundance of protons exists, and most of the drug tends to ionize as the proton is donated by the drug molecule, which results in a species that is highly charged and therefore lipid insoluble4 (Figure 6-3). The effects of pKa and pH on ionization are summarized in Box 6-1.6
Ion Trapping
Ion trapping has several anesthesia-related applications. Influences on oral absorption of drugs, maternal-fetal transfer, and central nervous system toxicity of local anesthetics are commonly cited. The degree of ionization for a specific agent can vary across a membrane that separates fluids with different pH values. For example, morphine sulfate, a base with a pKa of 7.9 when present in the blood (pH 7.4), exists in appreciable amounts in both ionized and nonionized forms. The uncharged drug fraction moves freely across tissue membranes, and the charged fraction does not. As the drug enters the stomach, a very acidic environment with a pH of 1.9, morphine accepts protons and becomes ionized, and ion trapping occurs4 (Figure 6-4 illustrates this phenomenon using diazepam as an example). The drug, however, will be absorbed later, as stomach contents move farther down the gastrointestinal tract to the more basic and favorable environment of the small intestine.
A similar scenario occurs when agents are transferred between a mother and a fetus, where the placenta is the membrane separating fluids with varying pH values—that of the fetus is more acidic than that of the mother. Again, the lipid-soluble fraction of basic agents such as lidocaine (pKa 7.9) crosses to the placenta easily. However, once there, because of the lower pH of the fetus, the drug becomes more ionized and cannot easily cross the lipid bilayer of placenta, resulting in accumulation of drug in the fetus. Finally, ion trapping may occur in a local anesthetic overdose situation in which high concentrations of a basic local anesthetic agent have entered the central nervous system and caused toxicity. If the patient experiences respiratory arrest and hypoxia, the resulting acidosis may trap the drug in the brain, resulting in prolonged and possibly more intense toxicity. Ion trapping also plays a role in the use of bicarbonate solutions and carbonated local anesthetics. A discussion of the effects of ion trapping on local anesthetics can be found in Chapter 10, Box 10-3.7,8
Protein Binding
Potential clinical changes are conceptualized by the following phenomena. Some drugs are bound extensively to proteins in the plasma because of their innate affinity for circulating and tissue proteins. The drug-protein molecule is too large to diffuse through blood vessel membranes and is therefore trapped within the circulatory system. Albumin is quantitatively the most abundant plasma protein, and although it is capable of binding basic, neutral, or acidic drugs, it favors acidic compounds. Two other proteins, α1-acid glycoprotein (AAG) and β-globulin, favor binding to basic drugs.9 Lipoproteins bind cyclosporine, and transcortin binds corticosteroids. Protein binding influences how a drug is distributed, because protein-bound drug is not free to act on receptors. High protein binding prevents the drug from leaving the blood to enter into tissue, which results in high plasma concentrations. The degree of protein binding for a drug is proportional to its lipid solubility such that the more lipid soluble an agent, the more highly protein bound it tends to be (Box 6-2).10
The number of potential binding sites on plasma proteins for drugs is finite; therefore the kinetics for binding behaves like any saturable process, in that protein binding can be overcome by adding more agent.4 The bond between drug and protein is usually weak, and they can dissociate when the plasma concentration of the drug declines or a second drug that binds to the same protein is introduced. For example, when a drug has been in chronic use and is at steady state, an equilibrium will be reached between free and protein-bound drug. If a new drug with a high affinity for the same protein sites is introduced, the new drug competes with the chronic drug for binding sites. This leads to displacement of the first drug with an increase in free fraction of that agent. It is important to note that the displaced free drug does become available for biotransformation and elimination, so unless these clearance mechanisms are at capacity, a rise in free fraction will lead to a small change in free plasma concentrations.
Protein binding is expressed in terms of the percentage of total drug bound. Drugs with protein binding greater than 90%, such as warfarin, phenytoin, propranolol, propofol, fentanyl and its analogs, and diazepam, are conceptualized to have an unexpected intensification of their effect if they are displaced from plasma proteins.11 Drugs that exhibit less than 90% binding have so little change in free active fractions that they are not a concern. The anticoagulant warfarin is commonly used as an example. Because warfarin is approximately 98% bound to plasma proteins, a reduction in bound fraction to 96% causes an increase in the free active fraction of the drug. Furthermore, when hypoalbuminemia exists, decreased albumin levels result in the availability of a greater amount of free drug. These theoretic concerns are rarely clinically relevant, as explained subsequently.
No clinically relevant examples of changes in drug disposition or effects can be clearly ascribed to changes in plasma protein binding. The idea that a drug displaced from plasma proteins increases the unbound drug concentration, increases the drug effect, and perhaps produces toxicity seems a simple and obvious mechanism. Unfortunately this simple theory, which is appropriate for a test tube, does not work in the body, which is an open system capable of eliminating unbound drug.12
The clinical application of plasma protein binding is only to help interpretation of measured drug concentrations. When plasma proteins are lower than normal, total drug concentrations are lowered, but unbound concentrations are not affected.1
Absorption
An important variable in the bioavailability of a drug at its effect site is the route by which the agent is administered. The route of administration determines how much of the drug is delivered to the systemic circulation. When the entire amount of drug given is delivered, the drug is said to have 100% bioavailability. Many routes of drug administration are used; each has advantages and disadvantages (Table 6-1). The routes of drug administration are enteral (involves the gastrointestinal tract); parenteral (injected subcutaneously, intramuscularly, intravenously, intrathecally, or epidermally); pulmonary; and topical.13 Absorption mechanisms of relevance to anesthesia are discussed in the following sections.
TABLE 6-1
Route | Bioavailability (%) | Comments |
Intravenous | 100 (by definition) | Most rapid onset; allows for titration of doses; suitable for large volumes |
Intramuscular | 75 to 100 | Moderate volumes feasible; may be painful |
Subcutaneous | 75 to 100 | Smaller volumes than intramuscular; may be painful; suitable for implantation of pellets |
Oral | 5 to 100 | Most convenient and economical; first-pass effect may be significant; requires patient cooperation |
Rectal | 30 to 100 | Less first-pass effect than oral; useful in pediatric patients |
Inhalation | 5 to 100 | Common anesthetic use for inhalation drugs, steroids, bronchodilators, and occasionally resuscitative drugs; very rapid onset (parallels intravenous administration) |
Sublingual | 60 to 100 | Lack of first-pass effect; absorbed directly into systemic circulation |
Intrathecal | Low (intentionally) | Specialized application, as with local anesthetics and analgesics, chemotherapy, and antibiotic administration; circumvents blood-brain barrier |
Topical | 80 to 100 | Includes skin, cornea, buccal, vaginal, and nasal mucosa; dermal application results in slow absorption; used for lack of first-pass effect; prolonged duration of action |
Enteral Administration
The oral route is the most common and convenient method for administration of drugs. It is relatively inexpensive, does not require sterile technique, and can be carried out with little skill. However, several disadvantages exist because many conditions—such as emotions, physical activity, and food intake—change the gastrointestinal environment. Therefore orally administered drugs tend to have a lower bioavailability. The stomach has a large surface area, and the length of time a drug remains there is a significant factor in absorption. Because of the low pH in the stomach (1.5 to 2.5), drugs that are highly acidic, such as barbiturates, tend to remain nonionized and are highly absorbed. Basic drugs that remain intact in the stomach acids can pass through and are more readily absorbed in the intestine. The small intestine is highly vascular and has an alkaline environment (pH 7 to 8).13
The enteral route often results in failure of the drug to be absorbed into the systemic circulation. Alternatively, chemical alteration may occur before entry into the intestines. Presystemic elimination refers to the elimination of drug by the gastrointestinal system before the drug reaches the systemic circulation. This occurs by means of three mechanisms: the stomach acids hydrolyze the drug (e.g., penicillin); enzymes in the gastrointestinal wall deactivate the drug; or the liver biotransforms ingested drug before it reaches the effect site.4 This liver activity is called first-pass hepatic effect. Drugs absorbed from the gastrointestinal tract after oral ingestion enter the portal venous blood and pass through the liver first, with subsequent delivery to the tissue receptors. In the liver they may undergo extensive hepatic extraction and metabolism before they have a chance to enter the systemic circulation (Box 6-3). Agents such as these exhibit large differences between oral and intravenous dosages. To have the desired effect, oral dosages must be exaggerated to compensate for the initial metabolism that occurs before the drug reaches the effect site.
The role transporters play in drug absorption and distribution should be further clarified. P-glycoprotein transporter is part of a larger family of efflux transporters found in the intestine, liver cells, renal proximal tubular cells, and capillary endothelial cells comprising the blood-brain barrier. Using ATP as an energy source, they transport substances against their concentration gradients. They appear to have developed as a mechanism to protect the body from harmful substances and can influence the ability of a drug to traverse barriers.
The sublingual and buccal routes of administration of drugs bypass the presystemic, portal system first-pass effect and are delivered rapidly to the superior vena cava for transport to the effect site.1 Nitroglycerin is an example of a sublingual drug that is put under the tongue and absorbed by the rich blood supply there. If ingested, the drug would be hydrolyzed by the stomach; therefore sublingual administration is the ideal route for this agent. Protein hormones that would also be digested by the stomach are instead placed between the gum and cheek (buccal administration) and enter venous drainage without undergoing hepatic, presystemic elimination.14,15
Occasionally the rectal route of drug administration is the ideal route for prevention of emesis caused by irritation of the gastrointestinal mucosa by the drug. It is also a preferred method of drug delivery for patients in whom oral ingestion poses difficulty. For example, rectal acetaminophen is administered to infants and young children undergoing general anesthesia for postoperative pain control. Drugs placed in the proximal rectum are absorbed into the portal system via the superior hemorrhoidal vein. They will therefore undergo significant first-pass effect in the liver before entering the systemic circulation, leading to unpredictable responses.1 Conversely, agents placed in the distal rectum do not undergo presystemic elimination and therefore have more predictable circulatory levels. The disadvantage historically associated with this route was the unpredictability of drug retention and absorption because of rectal contents. However, more recent studies have demonstrated that regardless of enema volume, agents are absorbed with consistent plasma concentrations within subjects.16
Parenteral Administration
Parenteral administration refers to administration by injection. The most rapid and predictable route to the systemic circulation is the parenteral route. With intramuscular injections, the drug is instilled deep into the muscle among the muscle fascicles.4 Subcutaneous agents are placed under the skin. With both intramuscular and subcutaneous injections, the systemic absorption of the drug is dependent on the capillary blood flow to the area and the lipid solubility of the agent.1 Conversely, intravenous injections allow for rapid and accurate delivery of drug into the systemic circulation. Parenteral administration is the route of choice for anesthetists, because it is an exact method of achieving the desired effect from agents delivered.
Pulmonary Administration
The lungs provide a large surface area for drugs administered by inhalation. Bronchodilators and antibiotics are administered via devices such as nebulizers, used to propel aerosols into the alveolar sacs.16 Anesthetic gases are also effectively administered through the lungs, as described in detail in Chapter 7.
Transdermal (Topical) Administration
The transdermal route is usually chosen for administration of a sustained release agent, providing the patient with a steady therapeutic plasma concentration. Drugs that are administered via this route must possess several characteristics. They usually exist in a combined form—both water soluble and lipid soluble. The water solubility is necessary so the drug can penetrate the hair follicles and sweat ducts. Once in the system, the drug must be lipid soluble to traverse the skin and exert effect at the receptors. These agents must have a molecular weight of less than 1000, dose requirements less than 10 mg in a 24-hour period, and a pH of 5 to 9.1 The area to which the drug is applied must have a relatively thin epidermis with a sufficient blood supply (Figure 6-5).
Bioavailability
Bioavailability is the extent to which a drug reaches its effect site after its introduction into the circulatory system. The rate at which systemic absorption occurs establishes a drug’s duration of action and intensity. Many factors play a role in the bioavailability of agents, including lipid solubility, solubility in aqueous and organic solvents, molecular weight, pH, pKa, and blood flow. For example, drugs given in aqueous solution are more rapidly absorbed than those given in oily solution or solid form because they mix more readily with the aqueous phase at the absorptive site.17,18 A recent study demonstrated that propofol in the form of a water-soluble prodrug is metabolized to propofol with a longer half-life, increased volume of distribution, delayed onset, and greater potency.19
The environment into which the drug is introduced also has an impact on its bioavailability. The patient’s age, sex, pathology, pH, blood flow, and temperature are all factors to consider. For example, pH plays a role when local anesthetic (a weak base) is injected into an infected wound (an acidic environment). In this instance, the local anesthetic is highly ionized (basic agent in acidic environment) and therefore cannot enter the lipid nerve membrane to reach the site of action. Some important concepts influencing absorption are listed in Box 6-4.
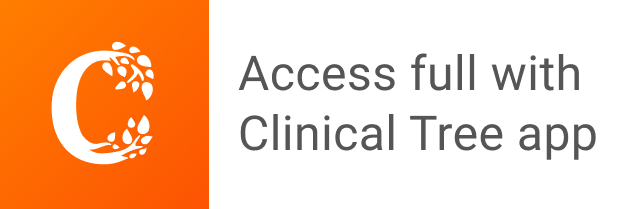