Fig. 41.1
Schematic representation of the events leading to type 1 and type 2 perioperative myocardial infarction . The acute coronary syndrome (type 1 myocardial infarction) will occur when an unstable plaque undergoes spontaneous rupture or fissuring. The consequence is an acute coronary thrombosis with myocardial ischemia and finally infarction. Central in the process of plaque instability is the intraplaque inflammation, but external factors occurring in the perioperative period may trigger the event. These factors include tachycardia and hypertension, the release of stress hormones, and the increase in procoagulant factors. Myocardial oxygen supply–demand imbalance (type 2 myocardial infarction) will occur whenever myocardial oxygen demand exceeds oxygen supply. Tachycardia is the most common cause of such an imbalance. Perioperative hypotension (hypovolemia or systemic vasodilation) or hypertension (elevated stress hormones, vasoconstriction), anemia, hypoxemia, and hypercarbia may also trigger or aggravate myocardial ischemia and aggravate pre-existing diastolic and systolic dysfunction
Acute Coronary Syndrome (Type 1 Myocardial Infarction)
This will occur when an unstable plaque undergoes spontaneous rupture, fissuring, or erosion. The consequence is an acute coronary thrombosis with myocardial ischemia and finally infarction. Central in the process of plaque instability is the intraplaque inflammation, but external factors occurring in the perioperative period may trigger the event. These factors include tachycardia and hypertension, the release of stress hormones, and the increase in procoagulant factors [20–23].
Myocardial Oxygen Supply–Demand Imbalance (Type 2 Myocardial Infarction)
Tachycardia is the most common cause of an imbalance in the myocardial oxygen supply–demand ratio [24, 25]. Perioperative hypotension (hypovolemia or systemic vasodilation) or hypertension (elevated stress hormones, vasoconstriction), anemia, hypoxemia, and hypercarbia may also trigger or aggravate myocardial ischemia and aggravate pre-existing diastolic and systolic dysfunction [17].
Timing of Perioperative Myocardial Ischemic Events
Perioperative myocardial ischemia predominantly occurs in the postoperative period [26]. Postoperative ischemia and infarction often start shortly after the end of surgery, at a moment when sympathetic discharge and procoagulant activity increase [24, 27]. Le Manach et al. distinguished two types of PMI according to time of appearance and rate of increase in troponin I [28]. Early PMI occurs in the early postoperative period (<24 h) and is not preceded by subinfarction myocardial damage, whereas delayed PMI occurs later (>24 h) and is preceded by a period of myocardial damage in which biomarkers are increased. They postulated that the mechanism underlying the early PMI pattern is that of the vulnerable plaque, whereas the biomarker release pattern of delayed PMI is more consistent with a prolonged myocardial oxygen demand–supply imbalance. In addition to these two patterns, some patients showed a prolonged period of subinfarction troponin I release, indicative for prolonged subclinical myocardial damage but without the development of PMI [28, 29].
Combining all available data on perioperative ischemia and infarction in patients undergoing noncardiac surgery, the following time sequence has been proposed [15]. First, ischemia starts in most patients (~67 %) immediately after the end of surgery and during emergence from anesthesia. Such ischemia is most often silent and can only be detected by continuous electrocardiographic monitoring. In most instances ischemia is preceded by an increase in heart rate. Because the ischemic ST changes usually revert back to baseline in almost all cases (even in patients who later develop increased levels of biomarkers), this episode may be easily missed in the absence of continuous monitoring. Patients with a prolonged period of myocardial ischemia may subsequently develop an increase in biomarkers, signifying myocardial damage. An increased value for cardiac troponin is defined as a measurement exceeding the 99th percentile of a normal reference population [19].
Only about 50 % of PMI patients will show symptoms of myocardial infarction, while the other 50 % of PMIs are silent and will remain unnoticed in the absence of electrocardiographic ST segment or biomarker analysis. The increase in troponin starts mostly within 8–24 h after the end of surgery [15]. When the increase in troponin occurs shortly after the prolonged period of ST segment depression, without sudden conversion to ST segment elevation, stress-induced ischemia and not plaque rupture is the most likely cause of PMI. The peak incidence of mortality in these patients is in the first 1–3 postoperative days. In patients in whom plaque rupture or coronary thrombosis is the cause of fatal PMI (~50 %), the timing of death is evenly distributed in the postoperative period, with no special correlation to the end of surgery [15].
Pathophysiology of Ischemia–Reperfusion Injury
Prolonged and unresolved interruption of blood supply to the myocardium without reperfusion ultimately causes myocyte cell death. Early restoration of blood flow to the ischemic myocardium is therefore necessary to prevent myocardial cell death to occur. However, reperfusion itself may lead to additional tissue injury beyond that generated by the ischemic event (Fig. 41.2). This phenomenon is called reperfusion injury, and it may manifest as arrhythmias, reversible contractile dysfunction (myocardial stunning), endothelial dysfunction, and ultimately irreversible reperfusion injury with myocardial cell death. This lethal reperfusion injury may result from two mechanisms which are necrosis and apoptosis.
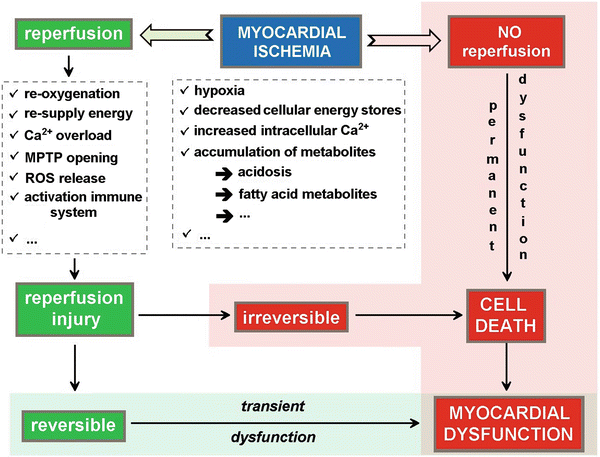
Fig. 41.2
Schematic representation of the different factors contributing to myocardial dysfunction and/or damage with ischemia–reperfusion injury. During ischemia, the hypoxic conditions result in a decrease and depletion of the energy stores and an accumulation of metabolites resulting in tissue acidosis. If no reperfusion occurs, the myocardial cell will ultimately die resulting in permanent myocardial dysfunction. Even if reperfusion is restored, there will be a period of transient myocardial dysfunction as a consequence of the ischemia–reperfusion injury. Upon reperfusion, the tissue reoxygenation triggers a burst of reactive oxygen species release and mitochondrial permeability transition pore (MPTP) opening (see Fig. 41.3), leading to disturbance of the electrochemical gradients over the mitochondrial membrane and swelling of the mitochondrial intermembrane space
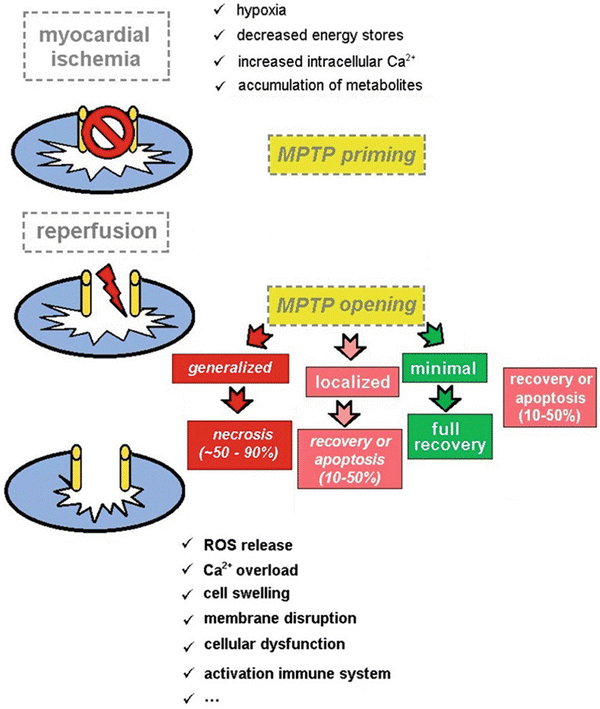
Fig. 41.3
Schematic representation of the presumed role of the mitochondrial permeability transition pore (MPTP) in the pathogenesis of ischemia–reperfusion injury. In normal conditions the MPTP is in a closed state. Also during the period of ischemia, the MPTP remains closed keeping mitochondrial integrity intact at this level. Upon reperfusion, the tissue reoxygenation triggers a burst of reactive oxygen species release, and the MPTP opens, leading to disturbance of the electrochemical gradients over the mitochondrial membrane and swelling of the mitochondrial intermembrane space. As a consequence, the supramolecular complex, containing the proton pump, ATP synthase, adenine nucleotide transporter, and mitochondrial creatine kinase, will disrupt, and normal mitochondrial function is lost. If the MPTP opening is minimal, full functional recovery of the cell may occur. When MPTP opening occurs in 10–50 % of the mitochondria, either recovery or apoptosis may occur depending on the extent of damage. However, if MPTP opening occurs in more than 50 %, necrosis of cell will occur leading to irreversible damage of the myocardium
The pathogenesis of reperfusion injury still is not fully elucidated, but several mechanisms have been shown to be involved [30, 31]. The major consistent metabolic abnormality that has been observed in the stunned myocardium is a reduction of the adenosine triphosphate concentration in the cells [32]. Because this resolves with a time course that is roughly parallel to that of the functional recovery [33], emphasis was initially placed on a potential role of high energy phosphates stores in the development of myocardial stunning. However, several lines of evidence have made it quite clear that adenosine triphosphate depletion has no major pathogenetic role in the development of reperfusion injury [34–36].
More recently the focus has turned to a potential role of reactive oxygen species and the disruption of the normal intracellular calcium homeostasis as major mechanisms involved in the pathogenesis of reperfusion injury. The oxygen paradox hypothesis is based on the observation that oxygen—while it is essential for tissue survival—may also be harmful during reperfusion of the ischemic myocardium [30, 31]. Indeed, upon reperfusion, molecular oxygen undergoes a sequential reduction with formation of reactive oxygen species, which interact with cell membrane lipids and essential proteins. This results in myocardial cell damage, initially with depressed myocardial function but ultimately leading to irreversible tissue damage. Although the role of reactive oxygen species in the pathogenesis of myocardial stunning has been established, the mechanisms by which oxygen-derived free radicals produce contractile dysfunction at the cellular level are still not fully understood. Since calcium is the major ion involved in the force generation by the myocardium, it seems likely that a change in extracellular calcium influx, intracellular calcium release, or reuptake by the sarcoplasmic reticulum or an alteration in myofilament sensitivity to calcium is also involved in the pathogenesis of depressed function with myocardial stunning [37].
In the last years, numerous reports provided solid evidence on the key role of the mitochondrial permeability transition pore (MPTP) in myocardial injury caused by ischemia and reperfusion. Following an acute episode of sustained myocardial ischemia, the opening of the MPTP in the first few minutes of reperfusion has been shown to mediate cell death. Opening of the MPTP renders the inner mitochondrial membrane nonselectively permeable resulting in a collapse of the mitochondrial membrane potential, thereby uncoupling oxidative phosphorylation , leading to adenosine triphosphate depletion and cell death. Another important effect of MPTP opening is mitochondrial matrix swelling and rupture of the outer mitochondrial membrane resulting in the deposition of proapoptotic factors into the cytosol, thereby initiating cell death [38–42] (Fig. 41.3).
Perioperative Cardioprotective Strategies
It is obvious that prevention of ischemic events is the primary prerequisite of a cardioprotective strategy. This is basically obtained by keeping the myocardial oxygen demand–supply ratio in balance. Whenever myocardial ischemia occurs, treatment should not only be directed toward a prompt restoration of blood flow to the ischemic area but should also include measures to prevent or minimize the extent of reperfusion injury.
Modulation of the Myocardial Oxygen Demand–Supply Ratio
Modulation of the myocardial oxygen demand–supply ratio will primarily aim at optimizing oxygen supply to the myocardium while keeping myocardial oxygen demand to a minimum. The latter will be obtained by avoiding hemodynamic instability and prevent tachycardia and hyper- or hypotension .
Most anesthetic techniques reduce sympathetic tone, which leads to a vasodilation with the potential development of a deceased blood pressure. Therefore, anesthesiological management must ensure proper maintenance of organ flow and perfusion pressure [43]. There is no universal target blood pressure value to define intraoperative arterial hypotension, but percentage decreases >20 % of mean arterial pressure, or mean arterial pressure values <60 mmHg for cumulative durations of >30 min are associated with an increased risk of postoperative complications such as myocardial infarction, stroke, and death [44–46].
Spinal or epidural anesthesia also induces sympathetic blockade. The benefit of neuraxial anesthesia versus general anesthesia is highly debated in the literature with proponents of a beneficial effect of neuraxial techniques and opponents who claim a lack of effect on criteria such as mortality or severe morbidity such as myocardial and pulmonary complications. Given the ongoing debate, the ESC/ESA guidelines gave a grade IIb recommendation, meaning that neuraxial anesthesia and analgesia may be considered for the management of patients with cardiovascular risk factors or diseases, provided no contraindications are present for the application of these techniques [43].
Several pharmacological strategies have been proposed to help stabilizing the perioperative hemodynamic conditions, in order to preserve the myocardial oxygen supply–demand ratio (Table 41.1).
Table 41.1
Pharmacological cardioprotective agents
✓ Anesthetic agents |
✓ Hemodynamically active agents |
➔ β-Blockers |
➔ Nitrates |
➔ Angiotensin-converting enzyme inhibitors |
➔ Angiotensin receptor blockers |
➔ Calcium channel blockers |
➔ α2-Receptor agonists |
✓ Others |
➔ Statins |
➔ Aspirin |
β-Blockers
Despite the lack of unequivocal evidence [47], international guidelines [48, 49] have put an important emphasis on the place of β-blockers in the prevention of perioperative cardiac complications . The generally accepted protective effect of perioperative β-blockade was seriously questioned by the results of the POISE study [50]. Perioperative treatment with metoprolol did indeed decrease the incidence of perioperative myocardial infarction compared to placebo, but this was at the expense of an increased mortality mainly related to a higher incidence of stroke in the metoprolol-treated patients . This increased incidence of stroke seemed to be related to the occurrence of intraoperative hypotension and bradycardia. Moreover, the concerns raised regarding the reliability of the Dutch Echocardiographic Cardiac Risk Evaluation Applying Stress Echocardiography (DECREASE) studies [51] further prompted skepticism regarding the appropriateness of β-blocking therapy in the perioperative period [52–55].
Clearly, the issue of perioperative β-blocking therapy is more complex than initially assumed [56]. Reasons for the heterogeneity of results among different studies on perioperative β-blocking therapy include influences of bias, length of the titration period, metabolism of the different β-blocking compounds, ratio of β1 to β2 selectivity, and others (Table 41.2). First, conclusions with regard to the beneficial effects of perioperative β-blocking therapy seem to have been skewed by a number of trials with a high risk of bias. Indeed, the results of the low-bias-risk studies clearly show that the beneficial effect on perioperative myocardial infarction rate is associated with an increased mortality and an increased rate of perioperative stroke, an observation which is blunted by the inclusion of high-bias-risk studies [47]. Second, the risk of adverse events with perioperative β-blocking therapy seems to be related to the length of the titration period. In the POISE study , the β-blocking therapy was initiated just before surgery at a fixed dose without appropriate titrating of the dose in function of the hemodynamic effects [50]. Timing of initiation of perioperative β-blocking therapy seems to play a pivotal role in the risk of stroke. In patients in whom β-blocking therapy is initiated within hours before surgery, an increased risk of hypotension and bradycardia is to be expected because of insufficient careful titration of the drugs with an increased risk of stroke as result. Conversely, in studies where β-blockers were started at least a week before surgery , allowing for more careful titration, the risk of developing perioperative stroke was not increased [57–59]. Thirdly, metabolism of β-blockers may be a factor to take into account when analyzing results of studies on perioperative β-blocking therapy. β-Blockers differ in their dependency on cytochrome P450 for metabolism. Many β-blockers (metoprolol, propranolol, carvedilol, labetalol, timolol) are metabolized by the P450 CYP2D6 isoenzyme. Metoprolol is the most dependent on this pathway with 70–80 % of its metabolism by CYP2D6 [60]. The CYP2D6 gene is highly polymorphic, and based on the number of copies of functional CYP2D6 alleles, patients can be classified in function of the degree of metabolism from poor to extensive metabolizers. Poor metabolizers have an up to fivefold higher risk for development of adverse effects (bradycardia and hypotension) during metoprolol treatment than normal metabolizers [61–63]. Fourth, even among β-blockers that are β1 cardioselective , variations exist in the ratio of β1 to β2 receptor affinity. Among the β-blockers used perioperatively, the β1 to β2 affinity ratios range from 13.5 for bisoprolol to 4.7 for atenolol and 2.3 for metoprolol [64]. Incidence of adverse events seems to be lower with perioperative β-blockers with a high β1 to β2 ratio [56, 65–67]. Finally, genetic polymorphisms at the level of β-receptors may determine cardiovascular outcome [68, 69] though they have not yet been shown to differentially affect specific β-blockers [56]. More important is the interaction of perioperative β-blocking therapy with the occurrence of intraoperative anemia. The beneficial effect of perioperative β-blocking therapy may revert to a deleterious effect on perioperative mortality in the presence of acute anemia with intraoperative bleeding [70, 71]. Finally, the protective effects of perioperative β-blockade seem to depend on the risk profile of the patient and the type of surgery involved. β-Blocking therapy was associated with lower mortality only in nonvascular surgery, and protection seemed more pronounced in the presence of a higher revised cardiac risk index score [72]. Similarly, among patients with ischemic heart disease undergoing noncardiac surgery, perioperative protective effects were only observed in patients with heart failure or recent myocardial infarction [73].
Table 41.2
Causes of heterogeneity with regard to protective effects of perioperative β-blocking therapy
✓ Low-bias versus high-bias-risk trials |
✓ Length of the titration period |
✓ Metabolism of β-blockers |
✓ β1 to β2 receptor selectivity |
✓ Perioperative anemia |
✓ Patient risk factors and type of surgery |
Currently, the guidelines give a class Ib recommendation to continue β-blocking therapy perioperatively in those patients who already receive this medication. There is indeed evidence of an increased mortality when preoperative β-blocking therapy is withdrawn [74–76]. In patients without clinical risk factors or undergoing low risk surgery, initiation of β-blocking therapy is not recommended [43]. Things are less obvious for intermediate risk patients. If the decision is made to initiate β-blocking therapy, the drug should be slowly uptitrated starting at a low dose in order to achieve a resting heart rate between 60 and 70 beats per minute. β1-Selective blockers without intrinsic sympathomimetic activity are favored, and evidence indicates that atenolol and bisoprolol are superior to metoprolol [65, 66]. Because of the need of slow uptitration to appropriate heart rate and blood pressure targets, initiation of treatment should be at least 1 week up to 30 days before surgery.
Other Vasoactive Drugs
Although nitrates are well known to reverse myocardial ischemia, the effect of perioperative intravenous nitroglycerine on perioperative ischemia is debated, and no effect has been demonstrated on the incidence of myocardial infarction or cardiac death. In addition, perioperative administration of the drug may impair the hemodynamic stability of the patient because of hypotension and tachycardia [43].
Perioperative use of angiotensin-converting enzyme inhibitors and angiotensin receptor blockers carries a risk of severe hypotension under anesthesia. Hypotension is less common when the drugs are discontinued the day before surgery, the reason why some consider withdrawal 24 h before surgery [43].
The beneficial effects of calcium channel blockers on the myocardial oxygen balance make them theoretically suitable for perioperative risk reduction strategies. However, the relevance of the available evidence is limited by the small size of the trials, the lack of risk stratification, and the absence of systematic reporting of adverse effects and complications. A meta-analysis of 11 randomized trials (1007 patients) showed a reduction in episodes of myocardial ischemia and supraventricular tachycardia, but decrease in mortality and myocardial infarction only reached statistical significance when both endpoints were combined [77]. Another study in elective aortic aneurysm surgery showed that dihydropyridine use was independently associated with an increased incidence of perioperative mortality [78]. Therefore, it is recommended to avoid the use of short-acting dihydropyridines, in particular, nifedipine [43].
α 2 -Receptor agonists reduce postganglionic noradrenaline output and may therefore be helpful in preventing perioperative hemodynamic instability. Perioperative administration of mivazerol did not reduce the incidence of death or myocardial infarction in the entire study population but seemed to have a protective effect in the subgroup of vascular surgery patients [79]. In a small study in 190 patients, perioperative administration of clonidine was shown to be associated with a reduction of perioperative cardiac morbidity and postoperative death in patients at risk for coronary artery disease undergoing noncardiac surgery [80]. However, more recently, the results of the POISE-2 trial clearly indicated that clonidine did not reduce the incidence of perioperative myocardial infarction or death but instead was associated with an increased incidence of important hypotension and nonfatal cardiac arrest [81]. Although some early data suggest that dexmedetomidine may be helpful in hemodynamic management of vascular surgery patients [82], to date, no firm evidence has demonstrated a perioperative cardioprotective action of the drug. A systematic review and meta-analysis of 20 studies including a total of 840 patients found no statistically significant evidence for improved cardiac outcomes but an increased evidence for perioperative hypotension and bradycardia [83]. Therefore, it was suggested that at this moment insufficient data are available to recommend the use of α2-receptor agonists in perioperative cardioprotective strategies [43].
Apart from pharmacological strategies aiming at a stabilization of the patient’s perioperative hemodynamic status, prevention of perioperative cardiac complications also includes avoiding the occurrence of thromboembolic events . Several drugs are available that—at least theoretically—may be of help in stabilizing the coronary plaques and/or prevent the occurrence of thromboembolic events.
3-Hydroxy-3-methylglutaryl coenzyme A reductase inhibitors or statins are widely prescribed in patients with or at risk for ischemic heart disease. They also induce coronary plaque stabilization through pleiotropic effects, thereby preventing perioperative plaque fissuring or rupture and subsequent myocardial infarction. Several studies have shown a beneficial effect of perioperative statin therapy on mortality and cardiovascular complications [84–89]. Even more, statin withdrawal more than 4 days is associated with a threefold increased risk of postoperative myocardial ischemia [90]. According to the current guidelines, patients with peripheral artery disease should receive statins. In patients not previously treated, statins should be initiated preferably at least 2 weeks before the procedure for maximal plaque-stabilizing effects and continued for at least 1 month after surgery. In patients undergoing nonvascular surgery, there is no evidence to support preoperative statin treatment if there is no other indication [43].
Aspirin is an antiplatelet drug prescribed to patients with established cardiovascular disease (secondary prevention) or with certain cardiovascular disease risk factors (primary prevention) to reduce major adverse thrombotic events such as myocardial infarction and stroke. Whereas the use of aspirin for secondary prevention of thrombotic events is based on high-quality evidence [91–95], the evidence supporting the use of aspirin for primary prevention is less robust [96, 97]. Data to guide the use of aspirin during the perioperative period are limited, and as a consequence, controversy exists on this issue [98, 99]. A large meta-analysis, including 41 studies in 49,590 patients, which compared periprocedural withdrawal versus bleeding risks of aspirin, observed that the risk of bleeding complications with aspirin was increased by 1.5, but that this did not result in a higher severity of bleeding complications. In subjects at risk of proven ischemic heart disease, aspirin nonadherence/withdrawal was associated with a threefold higher risk of major adverse cardiac events [100]. The POISE-2 trial, including 10,010 patients, found no difference in the composite endpoint of death or nonfatal myocardial infarction between placebo- and aspirin-treated patients. However, major bleeding occurred more often in the aspirin group [101]. On the basis of these results, it was concluded that the risk of continuing perioperative aspirin may not outweigh the risk of cessation [101]. These conclusions, however, have been challenged [102, 103], and the current guidelines recommend that the use of low-dose aspirin in patients undergoing noncardiac surgery should be based on an individual decision that depends on the perioperative bleeding risk weighed against the risk of thrombotic complications [43].
Modulation of Ischemia–Reperfusion Injury
During the past decades it has become increasingly obvious that important cellular damage may occur upon reperfusion after a period of myocardial ischemia . Interestingly, the extent of ischemia–reperfusion injury can be modulated by an intrinsic defense mechanism which is conditioning of the organ (Fig. 41.4). In 1986, Murry et al. observed that in an in vivo dog model, four 5 min occlusions of the circumflex coronary artery, each separated by 5 min of reperfusion, followed by a sustained 40 min occlusion were associated with a 25 % lower infarct size of that seen in a control group that received a single 40 min occlusion [104]. This phenomenon has been termed ischemic preconditioning . This study initiated extensive research —both experimentally and clinically—with regard to underlying mechanisms and potential clinical relevance.
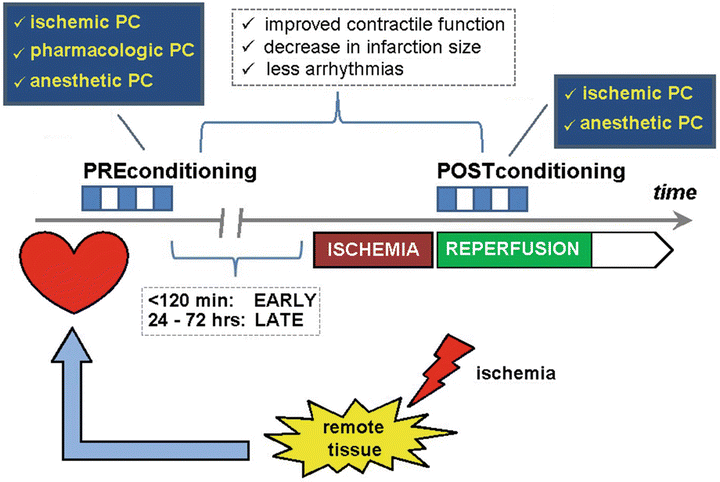
Fig. 41.4
Schematic representation of the principle of organ protection by the “conditioning” mechanism. Both preconditioning (PC) and postconditioning (PoC) are intrinsic defense mechanisms that help to attenuate the extent of ischemia–reperfusion injury. At the level of the heart, this will manifest as better preservation of contractile function, a decreased myocardial infarction size, and less occurrence of arrhythmias after the ischemic event. The phenomenon was first described as ischemic preconditioning after the observation that a number of short bursts of ischemia alternated with short periods of reperfusion resulted in protection against the consequences of a prolonged period of myocardial ischemia. Several protocols with varying time of repetitive ischemia and reperfusion have been proposed. Essential for the preconditioning is the washout period, i.e., the period between the last ischemic preconditioning trigger and the insult of prolonged myocardial ischemia. Depending on the length of the time interval between preconditioning and the actual ischemic insult, a distinction is made between “early” (<120 min) and “late” (24–72 h) preconditioning. Postconditioning involves the same principle, but now the ischemic trigger is applied after the event of myocardial ischemia. Since the concept of ischemic conditioning involves an additional ischemic burden to an already jeopardized, intensive research has been performed to possibilities of pharmacological modulation of the mechanistic pathways involved in the phenomenon of preconditioning. Several compounds have been tested such as adenosine receptor agonists, K ATP channel openers, protein kinase C agonists, etc. However, all the compounds tested showed important side effects prohibiting their use for “pharmacological” preconditioning in clinical practice. Only cyclosporine A (an inhibitor of MPTP opening) is capable of providing pharmacological protection against the extent of myocardial ischemia–reperfusion injury. Interestingly, volatile anesthetic agents have also been shown to confer direct (independent of the modulation of the myocardial oxygen balance) cardioprotective effects via a pre- and a postconditioning pathway. Especially in coronary surgery patients, application of this strategy seems to protect the heart with less myocardial damage and better functional recovery after ischemia. A similar cardioprotective effect can be obtained by an ischemic trigger in a tissue distant from the heart. This strategy is termed “remote ischemic preconditioning.” Typically, this ischemic trigger is applied on the skeletal muscle either of the upper or lower limb
It was only in 2003 that Zhao et al. introduced the concept of ischemic postconditioning . In an open chest dog model, three cycles of 30 s reocclusion were applied at the start of reperfusion after a 60 min occlusion of the left anterior descending artery [105]. Compared to a control group without reocclusion, a similar reduction in infarct size was observed as with an ischemic preconditioning protocol. This protective mechanism has since then been widely studied in order to determine both the pathways involved and its potential clinical applications.
This concept of endogenous cardioprotection was further refined by the intriguing observation that similar degrees of cardioprotection could be achieved by applying brief episodes of nonlethal ischemia and reperfusion to an organ or tissue remote from the heart. Skeletal muscle has been investigated as the most appropriate remote site for generation of such cardioprotection . In experimental studies, the application of an ischemic stimulus to skeletal muscle both before (remote ischemic preconditioning) and immediately after (remote ischemic postconditioning) myocardial ischemia has been shown to induce cardioprotective effects [106, 107]. More than direct ischemic conditioning, the concept of remote ischemic conditioning may constitute an attractive protective strategy to reduce the extent of ischemia–reperfusion injury [108], though the conclusions of these studies might be influenced by methodological issues [109].
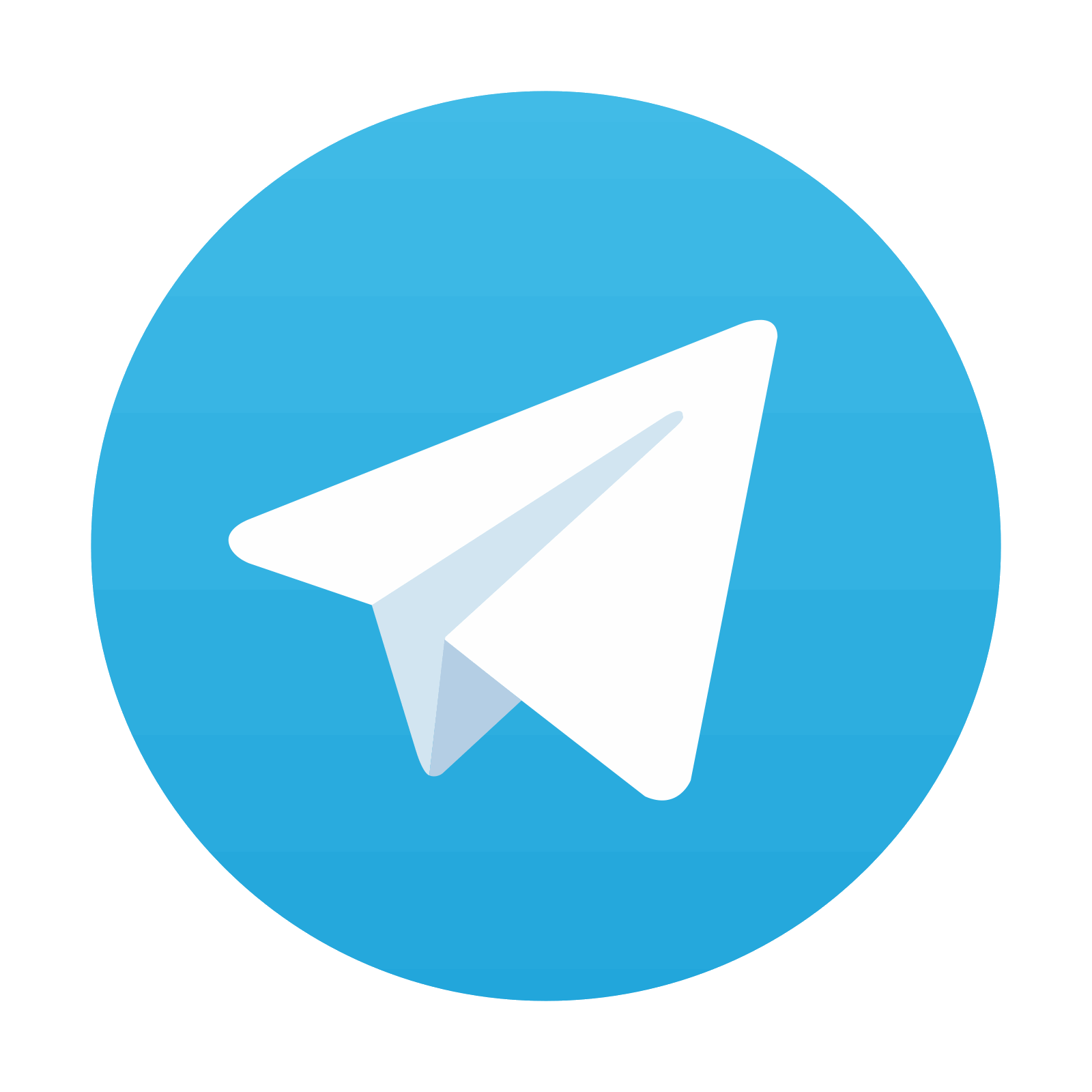
Stay updated, free articles. Join our Telegram channel
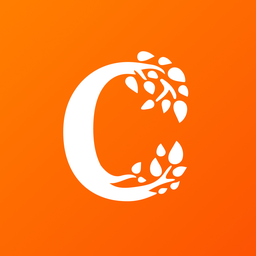
Full access? Get Clinical Tree
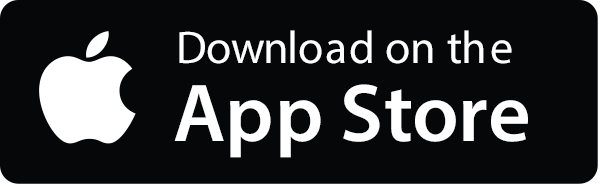
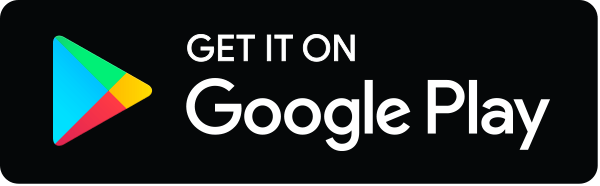