Pearls
- •
Acute respiratory distress syndrome (ARDS) is a restrictive lung disease with clinical manifestations of hypoxemia, low respiratory system compliance, and new pulmonary infiltrates. Pathologically, ARDS is characterized by diffuse alveolar damage, pulmonary edema, loss of end-expiratory lung volume, and presence of alveolar neutrophils.
- •
Direct insults leading to ARDS include pneumonia, gastric content aspiration, lung contusion, hydrocarbon ingestion, smoke inhalation, and mechanical ventilation. Indirect insults include sepsis, severe trauma and burn, blood transfusions, pancreatitis, major surgery, and ischemia-reperfusion injury.
- •
Injury to the alveolar epithelium and/or endothelium results in loss of alveolar epithelial-endothelial barrier function, with resultant accumulation of proteinaceous fluid in the alveolar space and surfactant inactivation.
- •
Cellular and soluble mediators of inflammation, surfactant inactivation and loss, coagulation dysfunction, alveolar fluid clearance, and apoptosis are pathobiological mechanisms of lung injury.
- •
Ventilator management for children with ARDS should embrace limiting both tidal volume and inspiratory pressure, allowing for permissive hypercarbia with sufficient levels of positive end-expiratory pressure to prevent alveolar derecruitment and limit high concentrations of inspired fraction of oxygen.
- •
There is not clear evidence to support the routine use of pulmonary or nonpulmonary ancillary therapies for children with ARDS, including surfactant and inhaled nitric oxide, but data supporting prone positioning is increasing.
Acute respiratory distress syndrome (ARDS) is a restrictive lung disease with severe hypoxemia and often hypercarbia. Diffuse disruption of the alveolar epithelial-endothelial barrier resulting in noncardiogenic pulmonary edema is the main pathologic finding. Lung-protective ventilation strategies that use lower tidal volume (Vt, lower inspiratory driving pressure, and higher positive end-expiratory pressure (PEEP) have been supported in the adult literature. Differences in practice patterns, comorbidities, and outcomes specific to pediatric patients led to a pediatric definition of ARDS in 2015.
In 1821, Laënnec published the “Treatise on Disease of the Chest” in which he described the gross pathology of the heart and lungs in an entity he termed “idiopathic anasarca of the lungs”—pulmonary edema without heart failure. This was perhaps the first scientific description of the same condition that has become a significant focus of contemporary medical research. This entity was described by the name of the inciting injury (e.g., shock lung, posttraumatic lung, DaNang lung) for many years. Finally, in 1967, Ashbaugh and colleagues published a case series of patients with severe hypoxemic respiratory failure, poor lung compliance, and diffuse alveolar infiltrates on chest radiograph. Although there was an 11-year-old among the 12 patients in the case series, they coined the term “acute respiratory distress syndrome in adults,” which was later revised to “adult respiratory distress syndrome.” , Acknowledgment that this syndrome also occurred in children did not occur until the 1994 American-European Consensus Conference (AECC), where it was renamed the acute respiratory distress syndrome (ARDS). ,
Clinical features: Pathophysiology
ARDS is a restrictive lung disease with reduced respiratory system compliance due to pulmonary edema, atelectasis, surfactant dysfunction, and chest wall restriction (e.g., chest wall edema, ascites, peritonitis). Hypoxemia results from pulmonary edema, loss of functional residual capacity (FRC), and the heterogeneous intrapulmonary shunt [(ventilation/perfusion (V/Q) = 0) and V/Q ratios <1] that occurs when FRC falls below closing capacity (CC). Regions with increased physiologic dead space (ventilation with reduced to no perfusion, or V/Q ratios >1) are also common in ARDS from mechanisms related to endothelial injury and coagulation, impaired cardiac output or pulmonary perfusion, and regional overdistension.
Chest imaging often shows evidence of patchy, asymmetric to diffuse opacities. In patients for whom the syndrome does not resolve, hypoxemia and low lung compliance persist, and alveolar dead space may worsen. In these patients, chest imaging may show linear opacities, formation of bullae, and pneumothoraces.
Definition
ARDS is an acute, inflammatory, diffuse, yet heterogeneous form of lung injury. However, like other clinical syndromes, ARDS lacks a definitive gold standard for clinical diagnosis. The AECC defined ARDS as acute, noncardiogenic pulmonary edema with bilateral pulmonary infiltrates on chest radiograph and an arterial oxygen partial pressure to inspired oxygen fraction (Pao 2 /Fio 2 ) of 200 or less. For nearly 2 decades, pediatric practitioners used the AECC definition for clinical care, research, and prognostication, despite its limitations. The Berlin definition in 2012 provided several modifications to oxygenation, minimum PEEP, timing of acute onset, chest radiograph, and pulmonary capillary wedge pressure criteria. , However, validation of the Berlin definition in pediatrics was limited by differences in the epidemiology and management of children with ARDS compared with adults. ,
The Pediatric Acute Lung Injury Consensus Conference (PALICC) published a pediatric definition of ARDS, termed pediatric acute respiratory distress syndrome (PARDS; Fig. 48.1 ) in 2015. , , The major differences between the Berlin and PALICC definitions are:
- 1.
The PALICC definition does not require bilateral infiltrates on chest radiograph but still requires evidence of pulmonary parenchymal disease.
- 2.
The PALICC definition allows substitution of peripheral capillary oxygen saturation (Spo 2 ) when Pao 2 is not available to assess hypoxemia severity, as long as Spo 2 is 97% or less.
- 3.
The PALICC definition introduces the use of the oxygenation index (OI) or oxygenation-saturation index (OSI) to stratify severity groups instead of Pao 2 /Fio 2 in those requiring invasive mechanical ventilation.

PALICC criteria do not define an upper age limit, although children with perinatal-related lung injuries are excluded. In addition, the PALICC definition of PARDS identifies an at-risk group and criteria to identify PARDS in patients with chronic lung disease, cyanotic heart disease, or left ventricular dysfunction. ,
Epidemiology
Using the AECC definition, the incidence of ARDS in US, European, Australian, and New Zealand children was estimated at 2.0 to 12.8 per 100,000 person-years. Observational studies in the 1990s and 2000s found that ARDS occurred in 3% to 6% of pediatric intensive care unit (PICU) patients, and between 5% to 8% of mechanically ventilated PICU patients. ARDS mortality in children appears to be lower than in adults (18%–27% vs. 27%–45%, respectively), although there are some populations in which adult and pediatric ARDS mortality appears similar (35%). , ,
Parvathaneni et al. compared the PALICC, AECC, and Berlin definitions among children admitted to a single multidisciplinary PICU in the United States and found that the PALICC criteria nearly doubled the number of patients diagnosed with PARDS largely because of the pulse oximetry–based criteria. The Pediatric Respiratory Distress Incidence and Epidemiology (PARDIE) study prospectively evaluated PALICC criteria in 145 international ICUs, representing 27 countries and 708 patients. PARDIE found that PARDS occurs in approximately 3% of PICU patients, or 6% of those treated with mechanical ventilation. Mortality is similar (approximately 15%) for those who are diagnosed with PARDS when on noninvasive ventilation (NIV) or those with mild or moderate PARDS at onset, with significantly higher mortality (>30%) for those with severe PARDS at first diagnosis. However, a delayed measure of PARDS severity (6 hours after PARDS onset) appears to better stratify mortality risk than initial PARDS severity. The PALICC definition identified approximately 40% more children as having ARDS and diagnosed ARDS a median 12.8 hours sooner than the Berlin definition within the first 3 days. The use of OI or OSI measurements by the PALICC definition seems to stratify mortality better than the Berlin Pao 2 /Fio 2 (P/F) ratio–based severity groups.
Overall mortality rates have decreased substantially among critically ill children. However, there is a paucity of data regarding long-term outcomes of children who survive PARDS. Adult data suggest decreased lung function and neurocognitive deficits that affect quality of life. , More research is needed to better describe the long-term morbidities and effect of ARDS on children.
Pathobiology
The primary pathologic description of ARDS is a diffuse injury to the alveolar epithelial-endothelial barrier (diffuse alveolar damage) resulting in noncardiogenic pulmonary edema. Infiltration of activated neutrophils into the alveolar space is a pathologic hallmark of ARDS. Disruption of alveolar fluid clearance and surfactant, dysregulated inflammation, apoptosis, and coagulopathy are additional pathobiological mechanisms associated with worse lung injury ( Fig. 48.2 ). The etiologies of ARDS can be divided into direct (alveolar epithelial) and indirect (endothelial) injuries ( Box 48.1 ). The three conceptual phases of ARDS (exudative, fibrosing, and resolution) overlap temporally but provide clinical and research frameworks of pathobiological relevance. As definitions of ARDS in adults and children continue to evolve, knowledge of pathobiological mechanisms will allow improvements in specificity of subtypes of the syndrome.

Exudative phase
The exudative phase is characterized by disruption of the alveolar epithelial-endothelial barrier by direct or indirect injuries to alveolar epithelial cells, pulmonary capillary endothelial cells, or both. The alveolar unit consists of alveolar epithelial and pulmonary capillary endothelial cells sharing a common basement membrane, making it efficient for gas exchange but susceptible to injury.
Direct injury
Early pathologic descriptions of diffuse alveolar damage (DAD) suggested a predominance of alveolar epithelial cell injury. Infectious pathogens and aspiration are the most common causes of direct alveolar epithelial injuries. , , Pathogens may cause alveolar cell necrosis, apoptosis, or pyroptosis. Alveolar epithelial cell necrosis and pyroptosis cause uncontrolled release of damage-associated molecular patterns (DAMPs) and proinflammatory cytokines.
Indirect injury
Indirect lung injury refers to injury to, or activation of, the pulmonary vascular endothelium. Sepsis is the most common cause of indirect lung injury. , Mechanical, chemical, and cellular injuries to the pulmonary endothelium cause alveolar barrier dysfunction, activate inflammatory and coagulation cascades, change pulmonary vascular resistance, and may lead to multiorgan dysfunction. ,
Several biomarkers of endothelial activation and injury have been associated with outcomes in children and adults with ARDS. , Angiopoietin 1 (Ang-1) and Angiopoietin 2 (Ang-2) are important endothelial growth factors that function in opposition. , The ratio of Ang-2/Ang-1 measured in plasma has been associated with mortality in adults with ARDS; high dead space fractions and plasma Ang-2 have been associated with mortality in adults and children with ARDS. The endothelial surface layer (ESL) is a glycocalyx composed of an apical extracellular matrix of glycoproteins, proteoglycans, and glycosaminoglycans (GAGs) creating an organized carpet-like layer between the endothelial cell membrane and the capillary lumen. , The glycocalyx contributes to fluid and molecular permeability of the endothelial barrier, transduces vascular shear stress, and regulates leukocyte-endothelial adhesion and platelet activation. , Injury to the pulmonary endothelial glycocalyx is likely to be an important mechanism of increased alveolar permeability, and restoration of the glycocalyx has been associated with resolution of pulmonary edema.
Alveolar fluid clearance
The integrity of the alveolar epithelial-endothelial barrier requires maintenance of a thin layer of alveolar wall liquid (AWL) coating the alveolar epithelium. Injuries to the alveolar epithelial-endothelial barrier result in increased permeability and disruption of alveolar fluid clearance (AFC), leading to pulmonary edema. The AWL is necessary for dispersion of surfactant; it is dependent on regulated flow of water, proteins, and small solutes across postcapillary venules into the alveolar airspace. Excess alveolar fluid is removed by sodium-dependent transport by type II alveolar epithelial cells.
The rate of AFC has been associated with mortality in adult patients with ARDS. Catecholamines, glucocorticoids, mineralocorticoids, thyroid hormone, growth factors (including keratinocyte growth factor [KGF]), and activation of the tumor necrosis factor receptor superfamily, affect AFC and have been tested as therapeutic targets, but none has improved outcomes in adults with ARDS. , β-Adrenergic agonists upregulate AFC in human lungs, but two randomized placebo-controlled trials of treatment with intravenous salbutamol did not show a reduction in ventilator-free days or mortality in adults with ARDS. Animal studies of KGF therapy for acute lung injury suggested that preinjury but not postinjury treatment is protective. However, a double-blind, randomized, placebo-controlled trial of KGF for adults with ARDS did not show improvement in oxygenation, ventilator-free days were fewer, and 28-day mortality was increased. ,
Leukocytes and inflammation
Infiltration of activated neutrophils into the alveolar airspace is a pathologic hallmark of ARDS, but transformed alveolar and recruited macrophages, epithelial cells, and T cells also mediate host innate immune inflammatory responses to pathogens and lung injury. , , Macrophages reside in the quiescent alveolar airspace and are the sentinels against pathogens. Alveolar macrophages express pathogen-associated molecular pattern (PAMP) and DAMP receptors, allowing them to detect the presence of pathogens and tissue injury and provide early warning signals. The alveolar epithelium, endothelium, and activated leukocytes contribute to local and systemic defense responses that are crucial to surviving direct injury to the lungs, but they also contribute to the pathophysiology of reduced lung compliance and V/Q mismatch.
Severe systemic inflammation (high levels of circulating cytokines and chemokines) activates the alveolar endothelium and circulating leukocytes, resulting in indirect lung injury. In addition to alveolar fluid accumulation from loss of barrier function, the cell surface adhesion molecules expressed on activated alveolar endothelial cells regulate neutrophil rolling, binding, activation, and migration into the alveolar space by both para- and transcellular mechanisms. , ,
Surfactant
Surfactant creates variable surface tension at the air-liquid interface of the alveoli and contributes to innate immunity. , Surfactant proteins B (SP-B) and C (SP-C) are hydrophobic and lower the surface tension of the AWL. The surfactant proteins A (SP-A) and D (SP-D) are collectins and contribute to innate immune responses to microbial pathogens. All four of the surfactant proteins have immunomodulatory effects and affect pulmonary fibrosis and lung remodeling. ,
Adults with ARDS have low levels of SP-A, SP-B, and SP-D in bronchoalveolar lavage (BAL) fluid. Increased serum levels of these proteins in children and adults are associated with severity of lung injury. BAL fluid of patients with ARDS have lower levels and changes in the overall composition of phospholipids. Reactive oxygen species from high concentrations of supplemental oxygen and activated neutrophils in the alveolar airspace may cause surfactant dysfunction. , Finally, patients with genetic polymorphisms that result in lower levels of SP-B have a higher risk of developing ARDS or have more severe lung injury when they become ill. Similar findings are seen with SP-A and SP-D with regard to development of ARDS for adults with pneumonia.
Coagulation
Endothelial function, inflammation, and coagulation are inextricably linked. , The intact glycocalyx is essential for normal intravascular anticoagulant function, and disruption of the glycocalyx has been associated with platelet activation and disseminated intravascular coagulation in sepsis. , Activation and/or injury to the endothelium results in expression of tissue factor (TF) and von Willebrand factor (vWF) antigen. vWF has been associated with mortality in pediatric and adult ARDS patients. , Plasminogen activator inhibitor type-1 (PAI-1) has also been associated with severity of illness and mortality in adult and pediatric patients with ARDS. , , Coagulopathy and fibrinolysis are not isolated to the vascular space in the lungs of patients with ARDS, as activated protein C, thrombomodulin, and TF activity have all been found in the alveolar compartment and are associated with alveolar epithelial cell function. , ,
Apoptosis
Apoptosis is a controlled, energy-dependent mechanism of programmed cell death that occurs by ligand-triggered extrinsic or stress-induced intrinsic pathways. Increased alveolar epithelial cell apoptosis is associated with severity of lung injury and mortality in adults with ARDS. , Studies suggest that multiple factors coregulate innate immunity, AFC, and apoptosis. Nuclear factor kappa-B (NFκB)-dependent innate immune signaling via Toll-like receptors also activates apoptotic pathways. , Fas ligand, transforming growth factor-β (TGF-β), and lipopolysaccharide mediate inflammation, AFC, and apoptosis of epithelial and endothelial cells in the lungs. ,
Apoptosis is important for normal postnatal lung development. Intersections between postnatal lung morphogenesis, inflammation, AFC, and apoptosis may affect the outcome of children with lung injury. , Fas has been shown to mediate apoptosis in normal lung development, and Fas/FasL may protect against hyperoxic lung injury in newborn mice. , Fibroblast growth factor 10 (FGF-10) regulates postnatal lung morphogenesis and appears to reduce DNA damage and apoptosis of alveolar epithelial cells treated with cyclic mechanical stretch. However, other animal studies suggest that mechanical ventilation during infancy may increase apoptosis in the lungs of newborns, thereby disrupting normal postnatal lung development. Age-dependent mechanisms of apoptosis in the lungs of patients with ARDS remain an opportunity for future research.
Fibrosis and repair phase
The acute proinflammatory response is essential to recover from direct lung injury, but prolonged inflammation in the lungs can be pathologic and lead to pulmonary fibrosis. , A balanced pro- and antiinflammatory response that results in appropriate resolution of inflammation once the inciting injury has resolved requires soluble mediators, cellular immunity, and, likely, stem cells. Unfortunately, experimental models to investigate pulmonary fibrosis and repair have been more challenging than those modeling the acute phase of ARDS.
Coordinated activity of type II alveolar epithelial cells, macrophages, neutrophils, T cells, dendritic cells, mesenchymal stem cells, and fibroblasts are all necessary for normal repair of the injured lung. Restoration of the endothelium and glycocalyx are also necessary to clear alveolar edema and restore the normal AWL. Additionally, neutrophil apoptosis is important for resolution of lung inflammation. , Although cell-cell interactions play important roles in the resolution of inflammation, soluble mediators (interleukin-10 [IL-10], granzyme B, and lipid mediators) are also required. ,
Multipotent mesenchymal stem (stromal) cells (MSCs) may be important to the resolution of lung inflammation and repair. MSCs appear to modulate inflammation, augment tissue repair, enhance pathogen clearance, and reduce severity of injury, pulmonary dysfunction, and death in experimental models of acute lung injury. , Many of these effects occur without engraftment; rather, they occur by paracrine effects. A fixed pool of MSCs in postnatal lungs that is depleted with age may be a mechanism for age-dependent differences in outcomes of patients with ARDS.
Ventilator management
Adult and animal data support ventilator strategies in ARDS that limit tidal stretch of the alveoli (avoiding volutrauma), limit inspiratory (driving) pressure (barotrauma), provide adequate PEEP to maintain end-expiratory lung volume to limit cyclic opening and closing of the alveoli (avoiding atelectrauma), and allow permissive hypoxemia and hypercapnia. Theoretical constructs of ventilator management in ARDS advocate ventilation in a “safe zone” characterized by location on the deflation limb of the quasi-static pressure volume curve, in the zone of optimal compliance ( Fig. 48.3 ). Adequate PEEP during conventional ventilation or mean airway pressure (MAP) during high-frequency ventilation may prevent atelectrauma by limiting cyclic opening and closing of alveoli. Limiting tidal stretch (by limiting tidal volume and/or peak inspiratory pressure) prevents overdistention, particularly of relatively spared alveoli with shorter inspiratory time constants. Despite these theoretical constructs, there is limited high-level evidence regarding the optimal methods or targets to achieve these strategies in children.

Considerations for ventilator mode
There is limited evidence to support that one mode of ventilation is superior to another for PARDS. However, volume control (constant flow pattern), used in the ARDS Network ARMA trial in adults, is infrequently used in pediatrics in favor of a decelerating flow pattern of pressure control or volume-targeted pressure control. This factor, among others, may limit the direct applicability of adult ARDS data to pediatrics. However, regardless of the mode of ventilation, the principles of lung-protective ventilation are applicable and should be used for children with ARDS.
Tidal volume
The benefits of targeted V t ventilation were established in the landmark ARDS Network ARMA trial. Mortality was reduced by 22% in the group receiving V t of 6 mL/kg ideal body weight (IBW) compared with patients receiving V t of 12 mL/kg IBW. Unlike the adult population, the pediatric data are inconclusive without a randomized control trial for V t . Observational pediatric studies have previously demonstrated lower mortality for patients treated with higher V t = 6 to 10 mL/kg. This is likely due to V t commonly being a dependent versus the set variable in pediatric mechanical ventilation. Higher V t for a set pressure represents better respiratory system compliance, implying that the patient is less ill. Location of measurement of V t (proximal airway vs. at the ventilator without compensation for ventilator tubing compliance), IBW versus actual body weight, and correction for leakage around endotracheal tubes are practice differences between adult and pediatric ICU providers that complicate extrapolation of adult V t data to children. Nevertheless, current recommendations advocate use of V t in or below the physiologic range (5–8 mL/kg) IBW, with lower V t targets in children with more compromised respiratory system compliance.
Peak or plateau pressure
There is sparse evidence to support a limit for peak or plateau pressure in pediatrics. These terms are commonly interchanged when pressure control ventilation is used. There may be few differences between peak and plateau pressure for children with ARDS managed with the decelerating flow pattern of pressure control ventilation if flow fully decelerates (dependent on inspiratory time and time constant of the lung) unless they concurrently have significant lower airway disease. Measuring plateau pressure requires a period of no flow during inspiration (an inspiratory hold). This maneuver may not be implemented routinely or consistently in pediatric critical care, and “plateau” pressure is often mislabeled. However, recent recommendations are to limit plateau pressure to 28 cm H 2 O for children with normal chest wall compliance, allowing slightly higher pressures when chest wall compliance is reduced. Ultimately, the shear stress induced by positive pressure ventilation is a function of transpulmonary pressure (alveolar pressure minus pleural pressure), potentially affording the use of higher inspiratory pressure when pleural pressure is also increased (e.g., obesity, anasarca). V t often gets the most attention, but the ARDS Network lung-protective 6 mL/kg strategy recommends decreasing V t below 6 mL/kg if needed to limit plateau pressure to 30 cm H 2 O. High-driving pressure (difference between plateau pressure and PEEP), independent of both plateau pressure and V t , is associated with higher mortality in adults with ARDS.
Positive end-expiratory pressure
PEEP should be set to prevent lung collapse and recruitment-derecruitment injury during tidal ventilation. Unfortunately, given the heterogeneity of lung disease in PARDS, it is difficult to use this principle to guide specific PEEP recommendations for individual patients. The optimal method to set PEEP for an individual patient remains a research question, and existing data do not support a single PEEP level (high or low) that improves outcome for adults or children with ARDS. Adult studies that protocolize PEEP/Fio 2 for oxygenation targets support higher levels of PEEP. , Recent adult data suggest that transpulmonary pressure-guided PEEP via esophageal pressure measurements rendered no mortality benefit compared with empirical high PEEP/Fio 2 . Adult studies of recruitment maneuvers combined with increased PEEP were the rationale for a multicenter, phase II randomized controlled trial in adults that did not improve ventilator-free days. A novel approach has been used to determine the ratio of the compliance of recruited lung versus “baby lung” during expiration, which may be a functional test of determining responders to higher PEEP.
Pediatric practice appears highly variable with respect to PEEP application, and PEEP is uncommonly increased to levels frequently used in adults with ARDS. , In a study of pediatric patients with PARDS, 27% were managed with lower PEEP relative to the amount recommended by the ARDS Network PEEP/Fio 2 protocol, and lower PEEP was independently associated with increased mortality among these patients.
Pediatric evidence is lacking, but moderately elevated levels of PEEP (10–15 cm H 2 O) titrated to oxygenation and hemodynamic responses are recommended for children with severe PARDS, with potential for higher levels of PEEP in the most severe patients. Importantly, plateau pressure should still be limited, and markers of oxygen delivery, respiratory system compliance, and hemodynamics should be closely monitored as PEEP is increased.
Nonconventional ventilation strategies
Various forms of nonconventional ventilation modes are considered for children with PARDS, particularly those with severe disease. The most common nonconventional mode is high-frequency oscillatory ventilation (HFOV). Although many practitioners use HFOV in PARDS, there are no data supporting improved outcomes; however, there are data suggesting improved gas exchange. As such, HFOV is frequently used as a rescue modality. The concept of HFOV is to ventilate with V t approximating the anatomic dead space, leading many to believe that it is an optimal form of lung-protective ventilation that should be considered earlier in the course of ARDS. However, animal studies suggest that treatment with high amplitude combined with low frequency can deliver high convective V t and cause ventilator-associated lung injury. Recent adult and observational pediatric data do not support use of HFOV early in ARDS. Current recommendations are to consider HFOV for patients with moderate to severe ARDS when adequate gas exchange cannot be maintained without exceeding suggested limits of plateau pressure (i.e., when lung protection cannot be maintained with conventional ventilation). However, optimal strategies to guide management of the different ventilator parameters on HFOV are an active area of investigation, with an ongoing pediatric interventional clinical trial investigating the use of HFOV on the outcomes of children with ARDS.
Alternative modes of nonconventional ventilation, such as airway pressure release ventilation (APRV) and high frequency jet ventilation (HFJV), may have some theoretical benefit for patients with moderate to severe ARDS, but there are no controlled data demonstrating their superiority to conventional modes of ventilation or HFOV for children with PARDS.
Ventilator-associated lung injury
Mechanical ventilation is the lifesaving support for patients with ARDS. The earliest observations of ARDS suggested that adding PEEP improved survival, and limiting V t remains the single most significant improvement to the care of patients with ARDS. , However, the converse is also true—mechanical ventilation may worsen lung injury. Ventilator-associated lung injury (VALI) describes the potential contribution of mechanical ventilation to patients with existing lung injury; ventilator-induced lung injury (VILI) is used to describe injury directly caused by mechanical forces. Many carefully designed laboratory studies have established multiple mechanisms by which the mechanical forces imposed on the respiratory system cause injuries in the lungs, but, because all patients with ARDS have a known clinical insult, VALI will be used throughout the rest of this chapter. VALI may occur by shear stretch (volutrauma), repeated opening and closing of atelectatic lung (atelectrauma), production of proinflammatory cytokines via mechanotransduction (biotrauma), and oxidative stress. , Barotrauma is a term that continues to be used to describe lung injury resulting in air leaks (pneumothorax, pneumomediastinum, and so on) as well as lung injury associated with high airway pressure. However, barotrauma is likely somewhat of a misnomer, as an elegant study by Dreyfuss and Saumon showed that pressure, in the absence of high absolute lung volume, did not cause lung injury.
The apparent discrepancy between volutrauma and barotrauma in the ARDS patient with significant portions of derecruited lung and high airway pressures can be reconciled by recognizing that, in heterogeneous lung disease, the energy of each V t is delivered to only the fraction of lung that inflates. , Amato’s work, showing that driving pressure was the variable that most accurately stratified risk, is consistent with the concept that mechanical power is the sum of the forces needed to recruit atelectatic and stretch inflated regions, divided by the time in which those forces are applied. , , This can be thought of as two related concepts: stress and strain. The stress of the lung is characterized by the driving pressure or, more precisely, the transpulmonary driving pressure. This should be limited. The strain is characterized by the V t applied/FRC. Hence, patients with more atelectasis and lower FRC will be more subject to strain-related injury with higher V t ventilation than those with more normal levels of FRC. This reinforces the importance of reducing the V t (and driving pressure) for those with the most severe disease, where FRC is most reduced.
PEEP and prone positioning have the potential to recruit atelectatic regions and thereby reduce the fraction of tidal stretch delivered to individual lung regions by increasing the total amount of inflated lung (i.e., improving FRC and potentially reducing strain). , This is the most likely physiologic rationale for studies that have shown improved outcomes in adult and pediatric patients with ARDS treated with higher PEEP. , However, if additional PEEP does not recruit atelectatic regions, then the remaining lung regions will rest at higher levels of inflation at end expiration and the fractional tidal stretch will be increased, thus, worsening volutrauma. In a study of children with ARDS, airway pressures were inadequate measures of stress and strain. Although HFOV has the potential to improve lung recruitment with elevated MAPs and avoid volutrauma by delivering ultra-low, subphysiologic dead space V t , it may also cause VALI. The HFOV frequency is inversely related to convective V t . Considering that the inspiratory time in which that V t is delivered is very short, there is potential for disproportionate forces to be applied to inflated lung. , , ,
There are many benefits to having patients breathe spontaneously while being treated with mechanical ventilation, but high amounts of power, causing worse direct injury to the lung, may be difficult to discern during spontaneous breathing. In order to reduce the power delivered to the lung, there was early recognition that hypercapnia should be tolerated. Although data suggesting that hypercapnia may even be therapeutic, patient dyspnea from the attendant respiratory acidosis remained a barrier to effective implementation of “lung-protective ventilation.” , Significant negative inspiratory forces generated at a high rate by the dyspneic patient with lung injury are likely to contribute to VALI—this situation is often called P-SILI for patient self-induced (or inflicted) lung injury. ,
Pulmonary ancillary therapies
Exogenous surfactant
The efficacy of exogenous surfactant for PARDS may depend on the concentration of individual surfactant proteins and other phospholipids. , Recent investigations have used exogenous surfactant preparations rich in either SP-B or SP-C given their direct properties on alveolar surface tension.
Preclinical or adult data
Lung injury studies in animals suggest that exogenous surfactant improves oxygenation, pulmonary compliance, alveolar edema, total lung water, and alveolar protein concentrations; plus, it may have an effect on lung inflammation.
Heterogeneous patient populations and study designs make interpreting adult data difficult. Exogenous surfactant appears to transiently improve oxygenation without affecting mortality, but some studies suggest harm related to adverse events such as hypoxemia (7%–50%), hypotension (9%–35%), transient airway obstruction, or rare events such as bradycardia, leukopenia, air leak syndrome and pneumonia. , Post-hoc analyses suggesting that treatment with recombinant protein-C surfactant benefited the subgroup of adults with ARDS due to direct injury were not confirmed in subsequent randomized trials. ,
Pediatric data
Early pediatric studies of exogenous surfactant for ARDS showed improvements in oxygenation and suggested reduced duration of ventilation and reduced mortality. Unfortunately, subsequent multicenter, randomized, placebo-controlled, blinded trials of calfactant did not show improved mortality or ventilator-free days. ,
A phase II placebo-controlled, double-blind, randomized controlled trial of a synthetic surfactant preparation (lucinactant) that mimics the actions of SP-B was performed in 165 nonpremature children with persistent hypoxemia. The most common diagnoses were bronchiolitis and pneumonia, and only 37 children met criteria for ARDS. Lucinactant was shown to be safe and improved oxygenation, but without an effect on duration of mechanical ventilation or mortality.
In summary, there is no evidence to suggest that exogenous surfactant reduces mortality for children or adults with ARDS. Exogenous surfactant can be safely administered in children, and oxygenation may transiently improve. The effects of surfactant seem to be confounded by the dosing regimen, drug delivery, drug concentration, surfactant formulation, degree of lung recruitment, lung disease severity, etiology of ARDS, comorbidities, and a multitude of other factors. Future research is warranted to identify whether subgroups of patients benefit from exogenous surfactant.
Nitric oxide
Physiologic rationale
Nitric oxide (NO) is normally synthesized in the vascular endothelium and causes vasodilation by relaxing smooth muscle via intracellular cyclic guanosine monophosphate (cGMP). Nitric oxide also affects inflammation by altering endothelial interactions with leucocytes and platelets. Since inhaled NO (iNO) is theoretically delivered only to ventilated lung units, its major effect is improved V/Q by increasing blood flow to ventilated units, thereby reducing physiologic dead space and improving oxygenation. , iNO may also be important for lowering pulmonary vascular resistance and supporting the right ventricle in patients with ARDS and pre-existing pulmonary hypertension.
Preclinical and adult studies
In addition to effects on pulmonary vascular resistance, animal data suggest that iNO may both aggravate and ameliorate lung injury. Some lung injury models show that iNO reduces neutrophil migration and oxidative burst, decreases lung inflammation, decreases platelet aggregation, and promotes lung repair. iNO may also improve pulmonary bacterial clearance through mechanisms related to endothelial permeability. , Other models suggest that iNO has no salutary effect or may potentiate lung injury through proinflammatory mechanisms.
Meta-analyses of iNO for adults with ARDS suggest that iNO does not reduce mortality. , Most studies demonstrate transient improvements in oxygenation within the first 24 hours, but there may be an increased risk of renal impairment (relative risk [RR], 1.59; 95% confidence interval [CI], 1.17–2.16) among adults treated with iNO as compared with controls. Despite these challenges, iNO continues to be used as a rescue therapy.
Pediatric
There have been a number of uncontrolled trials and small randomized controlled trials demonstrating improvements in oxygenation but not mortality or duration of ventilation with iNO administration for children with ARDS or acute hypoxemic respiratory failure. , , , A third small randomized controlled trial on 32 children with ARDS examined the potential synergistic effects of prone positioning and iNO, demonstrating more sustained improvements in oxygenation with the combination of prone positioning and iNO over the first 24 hours of ventilation. A recent multicenter trial has shown that iNO reduced the length of mechanical ventilation, mostly through lower use of ECMO. Unlike in adult studies, there does not appear to be evidence of significant toxicity with iNO administration in pediatric ARDS.
Pediatric and adult evidence suggest that iNO is probably best reserved for situations in which refractory hypoxemia needs to be temporarily ameliorated (e.g., as a bridge to extracorporeal support) or for patients with a true clinical indication, such as documented pulmonary hypertension with right heart dysfunction.
Nonpulmonary therapies
Prone positioning
Physiologic rationale
The best described and cited rationale for placing patients with ARDS in the prone position is to improve regional V/Q matching due to more uniform distribution of aerated lung, particularly in dorsal lung regions, thereby reducing physiologic dead space and improving oxygenation. , The effects of prone positioning on gas exchange are conventionally thought to be due to gravitational effects on the distribution of atelectasis and edema as well as the effects of the weight of the heart and position of the dorsal diaphragm on the expansion of dorsal lung units. , Interestingly, prone positioning also appears to improve the cephalocaudal distribution of ventilation, because the heart, mediastinal structures, and abdominal contents rest on the sternum. The prone position reduces regional differences in pleural pressure near the diaphragm that are normally present when upright or supine.
Prone positioning may also reduce inequalities in regional time constants and promote more effective alveolar ventilation to “slower” compartments. , These effects ultimately may influence lung strain and reduce the development of VALI. , When used in conjunction with high PEEP strategies currently recommended for ARDS, prone positioning may also increase alveolar recruitment and prevent cyclic recruitment/derecruitment and atelectrauma. Minimizing derecruitment may be a physiologic explanation of why additional recruitment strategies such as HFOV sustain temporary improvements obtained during prone positioning.
Patients with more compliant chest walls may have more benefit from prone positioning due to the reduction in chest wall compliance caused by limiting expansion of the sternum. This reduction in chest wall compliance, in addition to decreasing compression of the lungs by the heart and decreasing the vertical pleural pressure gradient in the lungs, theoretically allows preferential distribution of ventilation to the dorsal lung. However, there is contradictory evidence for this mechanism and the overall effect of chest wall properties on the oxygenation response to prone positioning of ARDS patients. It is also important to note that none of these studies was performed in children.
Other potential mechanisms for the beneficial effects of prone positioning include improved airway secretion clearance and lowering pulmonary artery pressure via attenuation of hypoxic pulmonary vasoconstriction. However, due to compromised preload, this effect may not directly translate into improvements in cardiac index. , ,
Adult data
Adult data demonstrate that the gas exchange response to prone positioning is not universal and benefits only a subset of patients. , , Moreover, the immediate improvement in oxygenation is inconsistently associated with a survival advantage in adults with ARDS. However, prone positioning has been associated with improved survival in adults for whom dead space ventilation improved. ,
There have been numerous randomized controlled trials of prone positioning for ARDS in adults. , , Meta-analyses highlight heterogeneity in these trials due to duration of prone positioning, use of concurrent lung-protective ventilation, V t , hypoxemia severity, use of HFOV, and duration of respiratory failure prior to prone positioning. , Nevertheless, these meta-analyses concluded that prone positioning affords a survival advantage for adults with ARDS, with the effect most pronounced in those who receive a longer duration of prone positioning, are managed with lung-protective ventilation strategies with regard to PEEP and V t , and who have more severe lung injury and hypoxemia. However, when pooling the adverse events, those in the prone-positioning group had a higher incidence of pressure ulcers, airway problems, and endotracheal tube obstruction.
The PROSEVA trial in adults is a major driver of the conclusions with regard to survival advantage in patients positioned prone. Prone positioning was maintained for a minimum of 16 consecutive hours, and ventilator management was standardized with a lung protective ventilation protocol. Mortality was 32.8% in the supine group, and 16% in the prone group at 28 days ( P < .001). Unlike other studies, there were no increased adverse events in the prone group as compared with controls. The study suggests that prone positioning may benefit adults with severe ARDS when done by experienced providers, long consecutive duration of prone positioning, and meticulous lung protective ventilation.
Pediatric data
The results of the Proning Severe ARDS Patients (PROSEVA) trial question existing data on prone positioning in children. Multiple uncontrolled trials and one randomized controlled trial on prone positioning have not demonstrated a survival advantage in children with ARDS. The highest level of pediatric evidence comes from a multicenter randomized controlled trial from 2005, which had detailed multidisciplinary protocols and guidelines for lung-protective ventilation, sedation, extubation readiness, hemodynamic support, nutrition, and skin care. , This unblinded clinical trial was stopped early secondary to futility: there were no differences in the primary outcome of ventilator-free days or the secondary outcomes of mortality, time to recovery of lung injury, organ failure, cognitive impairment, or functional health. Most of those patients would now be considered to have moderate ARDS; at the time that study procedures began, nearly half of the children had P/F ratios greater than 150. These results may be further confounded because adult data support more benefit from prone positioning for those with severe hypoxemia. Finally, while theory supports that children may have more pronounced benefits from prone positioning due to chest wall compliance, this benefit may be limited by less substantial compression of the lungs by the heart when supine as compared with adults. Future studies may benefit from the inclusion of measurements of transpulmonary pressure gradients and chest wall versus static lung compliance changes with prone positioning. There is an active pediatric interventional clinical trial investigating the use of prone positioning on the outcomes of children with severe ARDS.
Monitoring
Pediatric patients with or at risk of PARDS should receive at least clinical monitoring of respiratory frequency, heart rate, continuous pulse oximetry, and noninvasive blood pressure. Exhaled V t should be measured at the endotracheal tube, especially in the smallest patients, or corrected for tubing compliance if measured at the ventilator. Exhaled V t should likely be normalized to IBW, although spinal deformities and contractures may complicate measurement of height for calculation of ideal body weight in some patients. Inspiratory pressure (peak pressure in pressure modes, and plateau pressure in volume modes), flow, and volume should be measured continuously. Continuous monitoring of Spo 2 (with titration of Fio 2 titrated to keep Spo 2 ≤97%), PEEP, and MAP are essential to diagnose PARDS and monitor disease progression. Continuous capnography is important to determine the adequacy of support provided and to monitor physiologic dead space, which is associated with disease severity. Arterial blood sampling may be required when the accuracy of noninvasive monitoring is insufficient, and placement of arterial catheters may be indicated for hemodynamic assessments. Standard plain-film chest radiographs are indicated for diagnosis, determining appropriate position of equipment, and diagnosis of complications (e.g., pneumothorax).
Noninvasive support
Despite the widespread increased use of noninvasive support for pediatric and adult patients with acute respiratory failure, there is a paucity of data to determine the utility of these modes of respiratory support in ARDS. Noninvasive support of patients with ARDS has theoretical benefits, including avoidance of complications of intubation, preservation of spontaneous breathing and airway clearance, and reduced need for sedation. Noninvasive respiratory support can be considered for children with mild PARDS in a setting where trained and experienced staff can closely monitor for response to therapy and rapidly identify and treat deterioration. A multicenter observational study of children with PARDS suggests that approximately half of children with PARDS who are initially treated with NIV are intubated; mortality in these patients is similar to those with moderate to severe PARDS. Although this study showed that some children with PARDS can be managed without invasive ventilation, at this time there is not a means to determine which children will require intubation. However, the severity of underlying disease and an early response to noninvasive support appear to be primary determinants of success. Presence of a second organ failure, Fio 2 greater than 0.6 or Spo 2 :Fio 2 ratio less than 190, high severity of illness scores (Pediatric Logistic Organ Dysfunction [PELOD] or Pediatric Risk of Mortality [PRISM]), or moderate to severe ARDS suggest that noninvasive support will not be sufficient.
Once a patient is started on noninvasive support for respiratory failure, the patient must be closely monitored for clinical response. Failure to respond to noninvasive respiratory support within the first few hours has been associated with need for intubation, higher complication rates at the time of intubation, and higher mortality in adults. Evidence that a patient has failed to respond to noninvasive respiratory support may include absence of reduction in respiratory rate, pH less than 7.25 after 2 hours, increased oxygen requirement (Fio 2 >80% after 1 hour), a decreased P/F ratio or an S/F ratio less than 190, an increase of PaCO 2 , or an altered level of consciousness. , However, patients with very high respiratory drive may also be at high risk for poor outcome when left on NIV, by exacerbating P-SILI. Adult data, in fact, support worse outcomes for ARDS patients who achieve higher V t when on NIV. This is a population of pediatric patients and mode of support that requires study, as it is not clear whether early identification of patients that will require invasive mechanical ventilation could be more safely managed by avoiding noninvasive support with high patient work of breathing.
Extracorporeal life support
There are data from clinical trials of extracorporeal life support (ECLS) for neonates and adults with severe respiratory failure, but none in children. Data from the Extracorporeal Life Support Organization (ELSO) registry indicates that the average survival of more than 7000 children treated with ECLS for respiratory failure is 58%. ECLS should be considered for children with severe PARDS from reversible causes in centers with clearly defined ECLS teams. Clinical factors, including lower precannulation blood pH and precannulation mechanical ventilatory support greater than 2 weeks was associated with higher mortality. In a large cohort study involving 2449 children, children with severe ARDS treated with ECMO did not have better outcomes relative to children with severe ARDS who were not treated with ECMO. Improvements in diagnosis and risk stratification of PARDS will provide a means to perform better comparative effectiveness trials for ECLS with “lung rest” versus conventional lung protective ventilation.
Key references
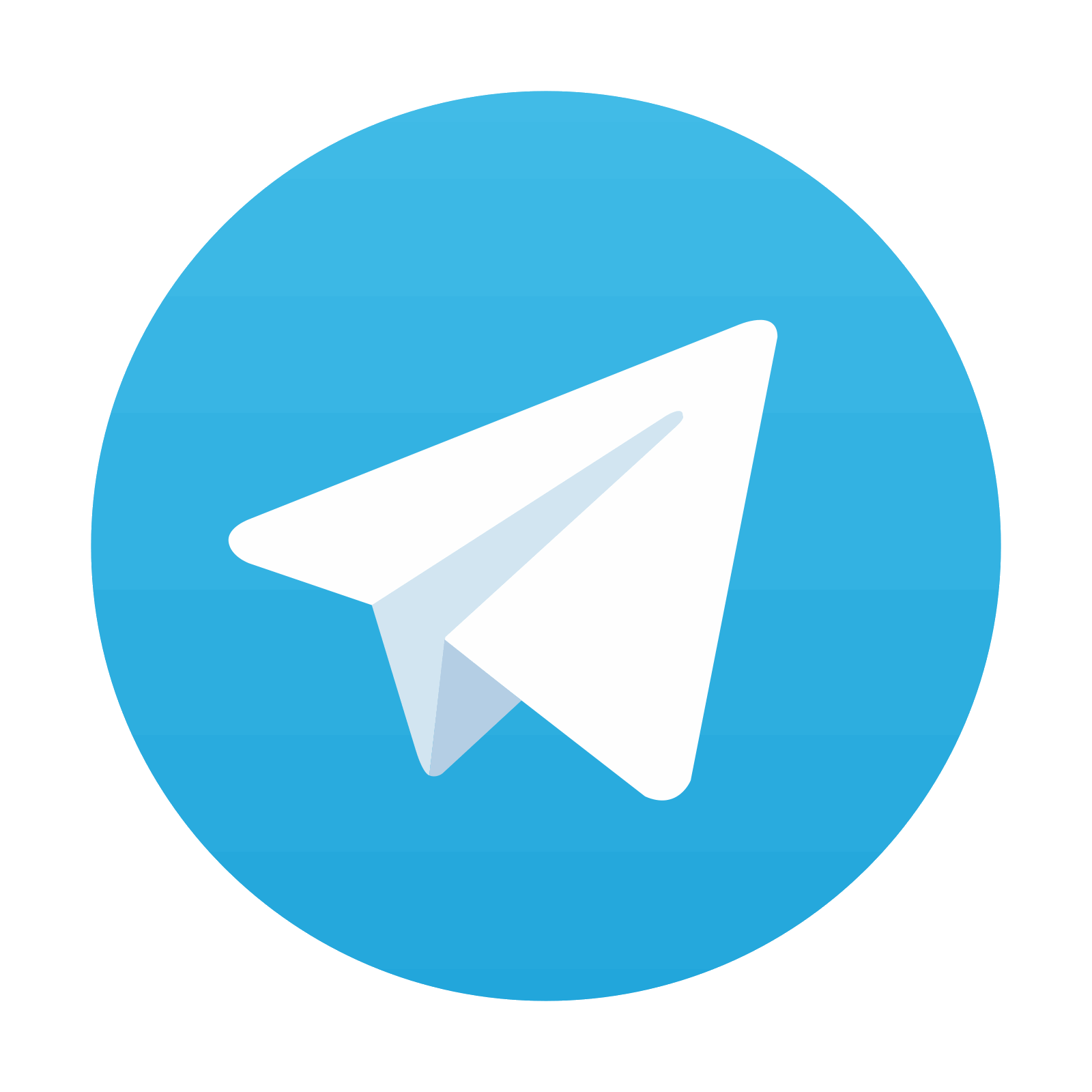
Stay updated, free articles. Join our Telegram channel
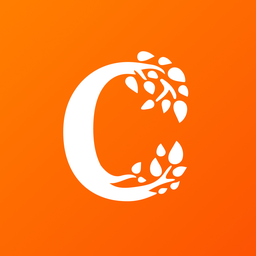
Full access? Get Clinical Tree
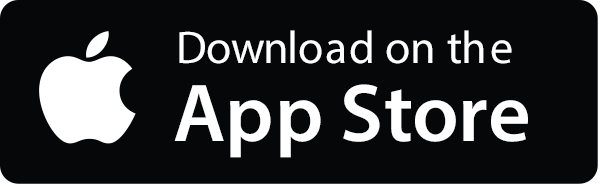
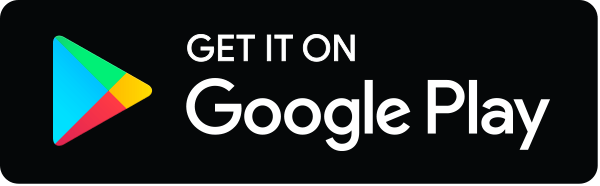
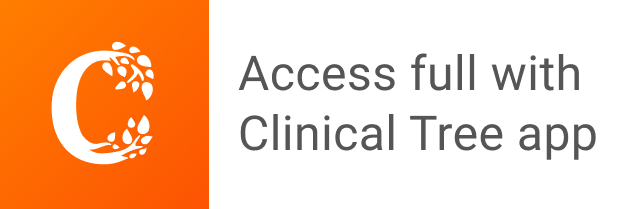