Chapter 40 Overview of Breathing Failure
Physiology of Breathing
Diaphragm (Structure and Function)
The diaphragm arises from the embryologic pleuroperitoneal fold. Myoblasts migrate from cervical somites to the pleuroperitoneal fold where they arrange themselves into a sheet on a mesenchymal substrate that separates the peritoneum from the abdomen. Once fully formed, the diaphragm originates from bilateral tendinous crura attached to the spinal column and inserts as a costal tendon attached to the chest wall between the sixth and twelfth ribs. The dome of the diaphragm remains largely tendinous. This structure, a circular attachment to the thoracic wall, vertical muscle orientation adjacent to the thorax, and attachment to a flattened central tendinous dome (Figure 40-1), works like a piston during breathing to enlarge the thorax and displace abdominal contents downward.
Approximately 50% of the diaphragm consists of type I fast twitch muscle fibers, which have high endurance and are resistant to fatigue. The remainder of the diaphragm is made up of type IIA and type IIB fibers, which have different properties.1 Type IIA fibers are important in achieving high levels of minute ventilation quickly, have good endurance, and can contract rapidly, but are not able to sustain long-term power output. Type IIB fibers cannot sustain their force of contraction because they possess lower oxidative capacity and are more susceptible to fatigue. The greater the force of contraction required, the more motor units of the diaphragm are recruited. There appears to be little difference between the activity of costal muscles and crural muscles, either during normal breathing or in response to hypoxia and hypercapnia.
When a patient lies supine, the diaphragm rests against the inner surface of the rib cage. When the diaphragm contracts and its muscle fibers shorten, the whole diaphragm moves down, lowering pleural pressure and increasing intra-abdominal pressure. The increase in intra-abdominal pressure generated by descent of the diaphragm acts as a caval pump to enhance cardiac filling.2 Because of its alignment against the lower ribs (zone of apposition), descent of the diaphragm also expands the caudal portion of the rib cage.
The muscle of the diaphragm extends from the costal insertion onto the dome of the diaphragm. When the diaphragm is “high,” it is loaded for greater force of contraction. When it is “low” or “flat,” it is unloaded and disadvantaged.
The diaphragm is the major inspiratory muscle of the neonate. It increases in thickness with age (as its muscle mass increases). It also becomes appositional to a longer segment of chest wall with growth, enhancing its effectiveness as an inspiratory piston.3 In the neonate, the muscular diaphragm has a greater angle from the vertical than that of the adult, which reduces its effectiveness as an air pump (Figure 40-2). This angle approaches zero with growth, increasing the diaphragm’s effectiveness with advancing age. The importance of this angle as an impediment to diaphragmatic effectiveness becomes exaggerated at total lung capacity, with air trapping, and when the abdomen is distended, all of which flatten and unload the diaphragmatic muscle (Figure 40-3).
Integrated Control of Breathing
The diaphragm and intercostal muscles work in unison, but also have individualized functions in breathing. When their functions are separately impaired, as in quadriplegia or diaphragm paralysis, abnormalities of gas exchange occur. DiMarco et al4 showed that ventilation to basal lung regions is generally preserved when intercostal function alone is impaired, whereas, with isolated diaphragmatic paralysis, ventilation of more cephalad regions of the lung is relatively preserved. Costal and crural portions of the diaphragm are both responsive to hypercarbia and hypoxia.5
Neural Automatic Control of Breathing
The generation of respiratory patterns resides in the brainstem, in a respiratory complex consisting of the dorsal and ventral medullary and pontine respiratory groups.6 These three neural groups coordinate and control inspiration and expiration. They modulate input from chemoreceptors, mechanoreceptors, and lung stretch receptors. In this manner, they integrate blood gas stimuli, chest wall tension, and lung stretch signals to generate and modulate an oscillatory pattern of breathing.7
The ventral medullary group functions in sleep, anesthetized, and awake states, but how organization of activity differs in these distinct states is not known. Blood pressure is tied into excitatory or depressant respiratory activity. This is thought to occur in the rostral ventromedullary area. It is also suggested that this area mediates responses to carbon dioxide (through both tidal volume and rate control), and responses to resistive breathing.8 Lesions in the ventral medulla have been implicated in the etiology of sudden infant death syndrome and apneic episodes.9
Chemoregulation in the Physiology of Breathing
The aortic arch and the bifurcation of the common carotid artery house chemoreceptors that respond to both hypercapnia and hypoxemia. Changes in carbon dioxide are recognized by both central and peripheral chemoreceptors. The brainstem may distinguish acute from chronic hypercapnia by sensing the acid base status of the cerebrospinal fluid. Sustained hypoxia evokes a cascade of ventilatory, neurochemical, and metabolic responses. Responses in immature animals are characterized by earlier and more marked depression of ventilation than is found in fully mature animals. Ventilation during hypoxia incorporates a number of compensatory mechanisms (stimulation or depression) from multiple systems. The time course of these responses is developmentally regulated. When hypercapnia is combined with hypoxia, the ventilatory responses are exaggerated.
Breathing Failure
Respiratory muscle fatigue develops during exhaustive exercise. Prolonged malnutrition has also been shown to affect the diaphragm’s muscle structure and to impair its ability to generate force. On the other hand, it has been shown that respiratory muscle training can lessen the development of respiratory muscle fatigue.10 Training of the diaphragm can increase capillary density, myoglobin content, mitochondrial enzyme concentration, and the concentration of glycogen, but persistent mechanical ventilation (particularly during deep sedation or paralysis) decreases muscle strength by allowing disuse muscle atrophy.
In acute illness, breathing fails if respiratory muscle demand for blood flow, metabolic substrate, and oxygen delivery outstrips supply, just as it does in exhaustive exercise.11 The point at which this occurs is influenced by many factors, including the energy cost of breathing, duration of contraction per breath, velocity of contraction, operational length of muscle fibers, energy supply, efficiency of muscles, and state of muscle training.12 Respiration can also fail if control of breathing is impaired. In any event, breathing failure, if untreated, may cause respiratory arrest and death.
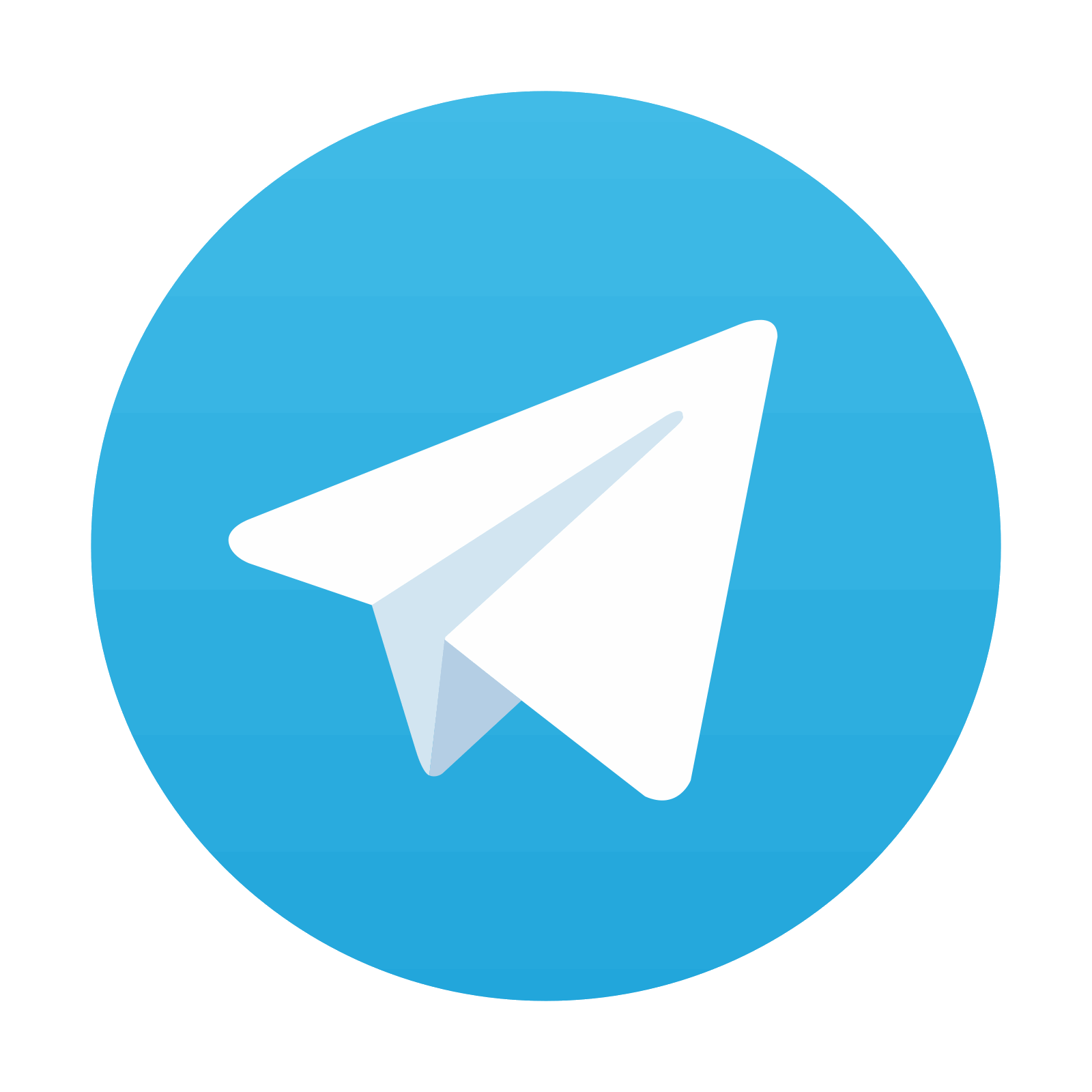
Stay updated, free articles. Join our Telegram channel
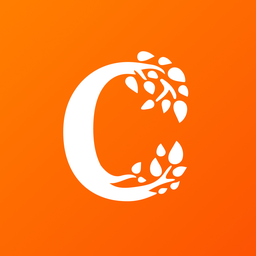
Full access? Get Clinical Tree
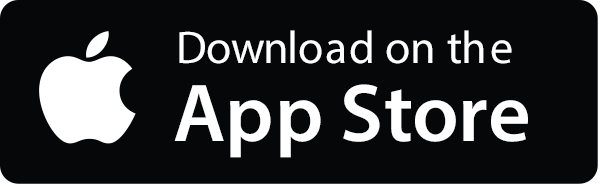
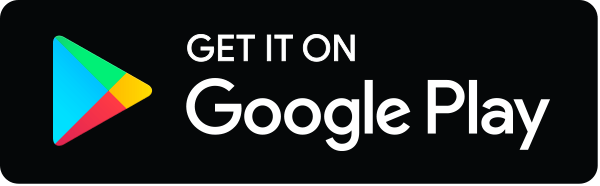