Key Words
Analgesia, Biased Agonism, Delta-, Dependence, Hyperalgesia, Kappa- Receptor, Metabolism, Mu-, Pharmacodynamics, Pharmacokinetics, Tolerance
Key Points
- ▪
Opioids are a vital part of providing the analgesic component of anesthesia and often form the foundation for postoperative pain management.
- ▪
Opioids suppress pain by targeting multiple sites throughout the nervous system including action in brain, spinal cord, and peripheral nervous systems.
- ▪
An increased understanding of the molecular pharmacology of opioid receptors and opioid-induced cellular responses allows utilization of innovative techniques for analgesia.
- ▪
Opioids also affect multiple organ systems, including the respiratory and cardiovascular systems, and can cause a variety of adverse effects. Proper dosing and monitoring may allow these adverse effects to be minimized.
- ▪
Pharmacokinetic and pharmacodynamic properties of opioids are affected by a variety of factors, such as age, body weight, organ failure, shock, and drug interactions. To administer opioids, these factors should be taken into consideration.
- ▪
Although new opioid delivery systems such as transdermal patches provide certain clinical advantages, they can also pose additional risks such as respiratory depression.
- ▪
Opioid analgesics serve a critical role in acute pain management but their role in the long-term treatment of chronic noncancer pain has been called into question due to increased risks of overdose and addiction.
Introduction
The remarkable beneficial effects of opioids, as well as their toxic side effects and addictive potential, have been known for centuries. The term opioid refers broadly to all compounds related to opium. The word opium originates from opos , the Greek word for juice, the drug being derived from the juice of the opium poppy, Papaver somniferum . In contrast, the term opiate refers to natural products derived from the opium poppy and includes morphine, codeine, and thebaine.
The first undisputed reference to opium is found in the writings of Theophrastus in the third century B.C. During the Middle Ages, many of the uses of opium were appreciated. Opium contains more than 20 distinct alkaloids. In 1806, Sertürner reported the isolation of a pure substance in opium that he named morphine, after Morpheus, the Greek god of dreams. By the middle of the nineteenth century, the use of pure alkaloids rather than crude opium preparations began to spread throughout the medical world. Since then, there have been ongoing efforts to develop semisynthetic and synthetic opioid analgesics without the adverse side effects. Unfortunately, many of the synthetic opioids share side effects of natural opioids. The search for new opioid agonists led to the synthesis of opioid antagonists and compounds with mixed agonist/antagonist properties, which further expanded therapeutic options and provided important tools for exploring mechanisms of opioid actions. Although new methods of opioid administration, including patient-controlled analgesia (PCA) and computer-based infusion techniques, have been developed, opioids continue to act on common binding sites throughout the nervous system.
Pharmacology of Opioids
Classification of Opioid Compounds
Opioids can be classified as naturally occurring, semisynthetic, and synthetic ( Box 24.1 ). The naturally occurring opioids can be divided into two chemical classes, phenanthrenes (morphine and codeine) and benzylisoquinolines (papaverine). The semisynthetic opioids are morphine derivatives in which one of several changes has been made. Synthetic opioids are classified into four groups, the morphinan derivatives (levorphanol), the diphenyl or methadone derivatives (methadone, d -propoxyphene), the benzomorphans (phenazocine, pentazocine), and the phenylpiperidine derivatives (meperidine, fentanyl, sufentanil, alfentanil, and remifentanil). Structures of opioid compounds are shown in Fig. 24.1 and Table 24.1 .
Naturally occurring
Morphine
Codeine
Papaverine
Thebaine
Semisynthetic
Heroin
Dihydromorphone, morphinone
Thebaine derivatives (e.g., etorphine, buprenorphine)
Synthetic
Morphinan derivatives (e.g., levorphanol, butorphanol)
Diphenylpropylamine derivatives (e.g., methadone)
Benzomorphan derivatives (e.g., pentazocine)
Phenylpiperidine derivatives (e.g., meperidine, fentanyl, sufentanil, alfentanil, remifentanil)

Opioids can be classified as agonists, partial agonists, mixed agonist-antagonist, and antagonists on the basis of their interaction with the opioid receptors.
Basic Studies on Opioid Receptors
In 1973, three independent teams of investigators described the presence of opioid binding sites in the nervous system from the radioligand binding assays. From pharmacologic experiments, three types of opioid receptors were postulated. They were named μ for morphine type, κ for the ketocyclazocine type, and σ for the SKF10047 ( N -allylnormetazocine) type. In addition, a high-affinity receptor for enkephalins was found in the mouse vas deferens, and was named the δ-receptor. Furthermore, an ε-receptor was proposed as the binding site for β-endorphin in the rat vas deferens. Pharmacologic actions of opioids and the involved receptors have been analyzed ( Table 24.2 ).
Actions of | |||
---|---|---|---|
Receptor | Agonists | Antagonists | |
Analgesia | |||
Supraspinal | μ, δ, κ | Analgesic | No effect |
Spinal | μ, δ, κ | Analgesic | No effect |
Respiratory function | μ | Decrease | No effect |
Gastrointestinal tract | μ, κ | Decrease transit | No effect |
Psychotomimesis | κ | Increase | No effect |
Feeding | μ, δ, κ | Increase feeding | Decrease feeding |
Sedation | μ, κ | Increase | No effect |
Diuresis | κ | Increase | |
Hormone Secretion | |||
Prolactin | μ | Increase release | Decrease release |
Growth hormone | μ and/or δ | Increase release | Decrease release |
Neurotransmitter Release | |||
Acetylcholine | μ | Inhibit | |
Dopamine | δ | Inhibit |
Since the early 1990s, molecular biologic studies have elucidated the molecular structures and signal transduction mechanisms of the opioid receptors. Four different complementary DNAs (cDNAs) have been isolated as members of the opioid receptor family. Investigators demonstrated that three of them correspond to the pharmacologically defined μ-, δ-, and κ-opioid receptors. The fourth receptor did not bind with opioid ligands with high affinities. Later, a novel peptide, nociceptin/orphanin FQ, was identified as an endogenous agonist of the fourth member of the opioid receptor family. The μ-, δ-, and κ-opioid receptors and the nociceptin receptor share approximately 50% amino acid sequence homology with each other. Characteristics of three opioid receptors and the nociceptin/orphanin FQ receptor are listed in Table 24.3 . Hydropathy analysis of the primary structures of the opioid receptors predicted that the opioid receptors possess seven transmembrane domains ( Fig. 24.2 ). This is a characteristic structural feature of G-protein-coupled receptors. Crystallographic analysis revealed that the μ-, δ-, and κ-opioid receptors and the nociceptin receptor actually possess seven transmembrane domains and the agonist binds deeply within a large pocket ( Fig. 24.3 ). Furthermore, analysis of the crystal structure of the murine opioid receptor revealed agonist-induced conformational changes in detail, providing the basis for the development of new ligands.
μ | δ | κ | Nociceptin | |
---|---|---|---|---|
Tissue bioassay | Guinea pig ileum | Mouse vas deferens | Rabbit vas deferens | — |
Endogenous ligand | β-Endorphin, Endomorphin | Leu-enkephalin, Met-enkephalin | Dynorphin | Nociceptin |
Agonist | Morphine, Fentanyl, DAMGO | DPDPE, Deltorphin | Buprenorphine, Pentazocine, U50488H | — |
Antagonist | Naloxone, Naltrexone | Naloxone, Naltrindole | Naloxone, NorBNI | — |
Coupled G protein | G i/o | G i/o | G i/o | G i/o |
Adenylate cyclase | Inhibition | Inhibition | Inhibition | Inhibition |
Voltage-gated Ca 2+ channels | Inhibition | Inhibition | Inhibition | Inhibition |
Inward rectifier K + channels | Activation | Activation | Activation | Activation |


The σ (sigma) receptor, which was previously classified as a member of the opioid receptor, was shown to be an endoplasmic reticulum-resident protein, and has been implicated in many diseases, ranging from cocaine or alcohol addiction to the most recently reported familial adult or juvenile amyotrophic lateral sclerosis. The amino acid sequence of the σ-1 receptor does not resemble that of any other mammalian proteins.
Further pharmacologic classification of the μ-receptor as the μ 1 , μ 2 , and μ 3 subtypes has been proposed, but the molecular identity of these receptor subtypes still remains to be clarified. Several possible molecular models of opioid receptor subtypes exist, including alternate splicing of a common gene product, receptor dimerization, and interaction of a common gene product with other receptor or signaling molecules. Multiple μ-opioid receptor species can be produced from a gene product of the μ-opioid receptor gene by alternate splicing ( Fig. 24.4 ). Analysis of the alternate splicing products reveals differences in ligand binding and G-protein activation. However, their physiologic, pharmacologic, or clinical implications remain to be elucidated. Interestingly, it was recently reported that the analgesic action of 3-iodobenzoyl-6β-naltrexamide, a potent analgesic against thermal, inflammatory, and neuropathic pain, is mediated by its interaction with mMOR-1G, a truncated splice variant possessing only six transmembrane segments, in mice.

G-protein-coupled receptors can form dimers, homodimers (same receptor), and heterodimers (different receptor types). The existence of these homodimers and heterodimers has been shown in cultured cells and in vivo. Among opioid receptors (μ, δ, κ, and nociception receptors), various combinations of the molecules have been shown to form dimers, and the dimer formation has been shown to affect the ligand binding properties and signal transduction mechanisms. Physiologic and clinical implications of dimer formation of opioid receptors will be further clarified. For example, it is interesting that a bivalent ligand containing μ-agonist and δ-antagonist pharmacophores effectively bridges μ-δ opioid receptor heterodimers and exhibits enhanced efficacy and a reduced tendency for tolerance in mice. However, in the peripheral nervous system, μ and δ receptors are apparently expressed in different sensory neuronal subpopulations.
Taken together, the overwhelming evidence of opioid receptor diversity in structure, ligand binding, and distribution supports the concept they serve both essential and complex signaling roles throughout the nervous system.
Genetic Variations Which Influence Opioid Effects
Several single nucleotide polymorphisms (SNPs) have been identified in the human μ-opioid receptor gene ( Fig. 24.5 ). The A118G mutation, the most common of these SNPs, leads to a change in the gene product in the human μ-opioid receptors, which is an A-to-G substitution in exon 1 that results in an amino acid exchange at position 40 from asparagine to aspartate (N40D). Patients with cancer who are homozygous for the A118G variant required higher doses of oral morphine for long-term treatment of their pain. A118G mutation of the human μ-opioid receptor gene reduces analgesic responses to morphine-6-glucuronide (M6G), but it does not significantly affect the respiratory depression induced by M6G. Furthermore, morphine consumption with intravenous PCA after total abdominal hysterectomy is significantly greater in women homozygous for the A118G variant than in other patients. In a meta-analysis involving 18 studies and more than 4600 patients, carriers of A118G were observed to exhibit higher opioid analgesic requirements. Another meta-analysis showed significant association between A118G polymorphism and susceptibility to opioid dependence or addiction in Asian populations.

In vitro experiments show that the variant receptors with A118G mutation are associated with higher binding affinity to and potency of β-endorphin, but lower potency of morphine. Studies in mouse models with analogous substitution of human A118G showed reduced analgesic response to morphine in some regions of the mouse brain with the GG genotype when compared with the AA genotype. A study also showed that 118A messenger RNA was 1.5- to 2.5-fold more abundant than the 118G messenger RNA in heterozygous brain autopsy tissues.
Overall, these findings suggest that the 118G allele may result in changes in abundance and/or function of the μ-opioid receptor leading to differences in antinociceptive potency of opioid analgesics. This in turn could misdirect healthcare providers in providing the most effective and safe opioid-based analgesic plan.
The C17T mutation of the human μ-opioid receptor, located in exon 1, causes an amino acid exchange from alanine to valine at receptor protein position 6 of the extracellular receptor terminal. This mutation has been reported to occur in a higher overall proportion of opioid-dependent persons, but the effects of the C17T polymorphism on analgesic responses are unknown. A recent study using cultured cells reported that adenylate inhibition via the C17T polymorphism was decreased for many opioids, including the clinically significant drugs morphine, buprenorphine, and fentanyl, as well as endogenous opioids, suggesting that this polymorphism may affect individual responses to opioid therapy, while the possible disruption of the endogenous opioid system may contribute to susceptibility to substance abuse.
It is known that genetic variation of genes other than opioid receptor genes can affect sensitivity to opioids. It has been demonstrated that the Val158Met polymorphism of the human catechol- O -methyltransferase (COMT) gene has contributed to differences in opioid consumption in patients treated for chronic cancer pain, and is associated with variability in opioid consumption in postoperative nephrectomy patients. COMT metabolizes biogenic amines including catecholamines such as dopamine, epinephrine, and norepinephrine, thereby acting as a key modulator of dopaminergic and adrenergic neurotransmission, and can affect the pharmacologic effects of opioids. Some studies have explored the relationship between gene-gene interactions and opioid responses. Kolesnikov and associates have demonstrated that the heterozygous patients with μ-opioid receptor A118G and COMT G1974A mutation consumed significantly less morphine compared with homozygous patients of A118. As investigation into the clinical impact of genetic variability continues, it is revealing that even greater complexity exists between gene-gene interactions and postoperative morphine consumption.
Endogenous Opioid Peptides
Enkephalin, β-endorphin, and dynorphin were identified as endogenous agonists for the δ-, μ-, and κ-opioid receptors, respectively. Following purification of these peptides from mammalian tissues, cDNAs for the precursors of these peptides were cloned. cDNA cloning and amino acid determination of preproopiomelanocortin demonstrated that the cleavage of this precursor protein produces not only β-endorphin but also several other neuropeptides including methionine-enkephalin, adrenocorticotropic hormone (ACTH), and α-melanocyte stimulating hormone. Amino acid sequence of preproenkephalin indicates that four methionine enkephalins and one leucine-enkephalin are cleaved from this precursor. Furthermore, the primary structure of preprodynorphin, the precursor of dynorphin, gives rise to dynorphin, leumorphin, and neoendorphin—ligands for the κ-opioid receptor.
Orphanin FQ or nociceptin, a novel endogenous opioid peptide with a significant sequence homology to dynorphin, was isolated in 1995 and so named because it lowered pain threshold under certain conditions in contrast to other endogenous opioid peptides. Pharmacologic and physiologic studies have demonstrated that orphanin FQ/nociceptin has behavioral and pain modulatory properties distinct from those of the three classic opioid peptides. However, studies of the effect of orphanin FQ/nociceptin on pain sensitivity have produced conflicting results, which may suggest that the effects of orphanin FQ/nociceptin on pain sensitivity depend on the underlying behavioral state of the animal. Prepronociceptin, the precursor of orphanin FQ/nociceptin, was cloned, and its amino acid sequence suggested the existence of prepronociceptin-derived neuropeptides other than orphanin FQ/nociception.
The search for endogenous ligand binding with the μ receptor with high affinity and high selectivity led to the discovery of a class of novel endogenous opioids termed endomorphin-1 and endomorphin-2. These peptides are tetrapeptides with the sequence Tyr-Pro-Trp-Phe and Tyr-Pro-Phe-Phe, respectively. An endomorphin gene has yet to be cloned, and much remains to be learned about the anatomic distribution, mode of interaction with the opioid receptors, function in vivo, and potential existence of other related peptides that are highly selective for each of the opioid receptors. It was recently demonstrated that both endomorphin-1 and endomorphin-2 given centrally and peripherally produced potent antiallodynic activities in a mouse model of neuropathic pain, and downregulation of endomorphin-2 correlates with mechanical allodynia in a rat bone cancer pain model.
Intracellular Signal Transduction Mechanism of Opioid Receptors
The opioid receptors belong to the G-protein-coupled receptor family. It has been demonstrated that activation of the opioid receptors leads to activation of the pertussis toxin-sensitive G proteins (G i or G o or both). Expression of the cloned opioid receptors in cultured cells by transfection of the cloned cDNAs has facilitated analysis of the intracellular signal transduction mechanisms activated by the opioid receptors ( Fig. 24.6 ). Adenylate cyclase is inhibited by opioid receptor activation, with a resulting reduction of the cellular cyclic adenosine monophosphate (AMP) content. Electrophysiologically, the voltage-gated Ca 2+ channel is inhibited and the inwardly rectifying potassium (K + ) channels are activated by the opioid receptors. As a result, neuronal excitability is reduced by activation of the opioid receptors. However, the role of adenylate cyclase in opioid receptor activation is complex. For example, long-term tolerance to opioids has been thought to be associated with superactivation of adenylyl cyclase activity, which is a counterregulatory response to the decrease in cyclic AMP levels seen after acute opioid administration. That this effect is prevented by pretreatment of cells with pertussis toxin demonstrates involvement of G proteins (G i or G o or both).

Beyond adenylate cyclase, other regulatory components participate in the coupling of opioid receptor binding and a cellular response. It was shown that extracellular signal-related kinase, a class of mitogen-activated protein kinases, is activated by the opioid receptors. Opioid-induced activation of extracellular signal-related kinase can lead to increase in arachidonate release and expression of immediate early genes, c-fos and junB.
Chronic exposure of the opioid receptors to agonists induces cellular adaptation mechanisms, which may be involved in opioid tolerance, dependence, and withdrawal symptoms. Several investigators have shown that short-term desensitization probably involves phosphorylation of the opioid receptors through protein kinase C. A number of other kinases also have been implicated, including protein kinase A and β-adrenergic receptor kinase (BARK), a member of the G-protein-coupled receptor kinase (GRK). BARKs selectively phosphorylate agonist-bound receptors and thereby promote interactions with β-arrestins, which interfere with G-protein coupling and promote receptor internalization. β-Arrestin 2 functions as a scaffolding protein that interacts with signal transducers, and the recruitment of β-arrestin 2 induced by opioid receptor activation is involved in the regulation of activity of c-Src, Akt, and mitogen-activated protein kinases ( Fig. 24.7 ). The c-Src inhibitor, dasatinib, attenuated and reversed morphine-induced tolerance in mice, suggesting that c-Src recruited by β-arrestin 2 is involved in the morphine-induced tolerance. Acute morphine-induced analgesia was enhanced in mice lacking β-arrestin 2, suggesting that this protein contributes to regulation of responsivity to opioids in vivo. Therefore, β-arrestin modification by associated kinases serves a critical role in the coupling between agonist binding to opioid receptors and their ability to develop and sustain an analgesic response.

Like other G-protein-coupled receptors, the opioid receptors can undergo rapid agonist-mediated internalization by a classic endocytic pathway. These processes may be induced differentially as a function of the class of the ligand. For example, certain agonists, such as etorphine and enkephalins, cause rapid internalization of the μ receptor, whereas morphine, which decreases adenylyl cyclase activity equally well, does not cause μ receptor internalization in all cell types such as HEK293 cells. Although these findings may suggest that different ligands induce different conformational changes in the receptor that lead to divergent intracellular events, they also have revealed that rapid morphine–dependent μ receptor endocytosis is still possible in subpopulations of central nervous system (CNS) neurons such as striatal neurons. Taken together, they may help provide an explanation for differences in the efficacy and abuse potential of various opioids.
Biased Agonism
Chemically distinct ligands binding to the same G-protein–coupled receptor can stabilize the receptor in multiple active conformations. In this way, differential activation of G-protein–coupled cell signaling pathways may produce divergent physiologic outcomes, a phenomenon known as biased agonism. Biased agonism can be exploited to design drugs that selectively activate desired signaling pathways, while minimizing other signaling pathways via the same receptor subtype and associated side effects.
Morphine binds to the μ-opioid receptor protein to form an active complex with signaling proteins, including G i/o and β-arrestin. The G i/o signaling pathway is thought to mediate analgesic action of morphine, whereas β-arrestin signaling results in unwanted side effects including euphoria, which can lead to addiction, respiratory depression, and gastrointestinal effects. Consistently, it was shown that the analgesic properties of morphine were enhanced but morphine-induced respiratory depression and constipation were attenuated in β-arrestin 2 knockout mice. Emerging research in this evolving field includes a report by Manglik and associates, who computationally screened 3 million molecules, resulting in the compound PZM21, which showed high G i/o -biased signaling and suppressed pain in mice without constipation, respiratory depression, hyperlocomotion, and addiction–related behaviors. The clinical importance of these findings awaits further confirmation given other reports showing little difference in adverse effect profiles between morphine and PZM21 in mice. However, TRV130, a “G protein-biased” μ-opioid ligand with G-protein–coupling efficacy similar to that of morphine, but markedly reduced receptor phosphorylation, recruitment of β-arrestin 2, and internalization, was tested in a randomized, double-blind, placebo-controlled crossover study in healthy volunteers. TRV130 produced greater analgesia than morphine, but less respiratory depression and less severe nausea ( Fig. 24.8 ). As additional biased-agonist candidates of the μ-opioid receptor emerge, so does the hope for a new generation of clinically effective analgesics with a reduced profile of harm.

Mechanism of Opioid Analgesia
Brain
Pain control by opioids needs to be considered in the context of brain circuits modulating analgesia and the functions of the various types of receptors in these circuits. It is well established that the analgesic effects of opioids arise from their ability to inhibit directly the ascending transmission of nociceptive information from the spinal cord dorsal horn and to activate pain control circuits that descend from the midbrain, through the rostral ventromedial medulla (RVM), to the spinal cord dorsal horn. Petrovic and colleagues used an experimental pain model and positron emission tomography (PET) to study mechanisms of action of the short-acting μ-opioid agonist remifentanil and found drug-induced activation of the rostral anterior cingulate cortex, insula, orbitofrontal cortex, and brainstem areas. The activated brainstem regions overlapped with brain areas that have been implicated in pain modulation, such as the periaqueductal gray (PAG). Interestingly, placebo analgesia acts similarly on the activity of these brain areas, presumably via endogenous opioid release.
Immunohistochemical studies and in situ hybridization analysis have demonstrated that opioid receptors are expressed in various areas in the CNS. These areas include the amygdala, the mesencephalic reticular formation, the PAG, and the RVM. However, the role of the opioid receptors in all of these areas has not been completely clarified.
Microinjection of morphine into the PAG or direct electrical stimulation of this area produces analgesia that can be blocked by naloxone. Opioid actions at the PAG influence RVM, which in turn modulates nociceptive transmission in the dorsal horn of the spinal cord through the action of the descending inhibition pathway. Thus, opioids produce analgesia by direct actions on the spinal cord, as well as by neurally mediated action in the region separated from the site of opioid administration. Interestingly, a study by Dogrul and Seyrek reported that spinal serotonin 5-HT7 receptors play an important role on the antinociceptive effects of systemic morphine.
It is well known that the actions of opioids in bulbospinal pathways are critical to their analgesic efficacy. It is clear that opioid actions in the forebrain contribute to analgesia, because decerebration prevents analgesia when rats are tested for pain sensitivity using the formalin test, and microinjection of opioids into several forebrain regions are analgesic in this test. Analgesia induced by systemic administration of morphine in both the tail-flick and formalin tests was disrupted either by lesioning or reversibly inactivating the central nucleus of the amygdala, thereby demonstrating that opioid actions in the forebrain contribute to analgesia after tissue damage, as well as after acute phasic nociception.
The distribution of opioid receptors in descending pain control circuits indicates substantial overlap between μ and κ receptors. Interactions between the κ receptor and the μ receptor may be important for modulating nociceptive transmission from higher nociceptive centers, as well as in the spinal cord dorsal horn. The μ receptor produces analgesia within descending pain control circuits, at least in part, by the removal of γ-aminobutyric acidergic inhibition of RVM-projecting neurons in the PAG and spinally projecting neurons in the RVM. The actions of μ-receptor agonists are invariably analgesic, whereas those of κ-receptor agonists can be either analgesic or antianalgesic. The pain-modulating effects of the κ-receptor agonists in the brainstem appear to oppose those of μ-receptor agonists.
Spinal Cord
Analgesic actions of systemic morphine are in part mediated by a net inhibitory effect from the PAG and RVM on nociceptive processing in the spinal dorsal horn. It was demonstrated that morphine increased 5-HT release in the spinal dorsal horn by activation of serotonergic neurons in the RVM, and intrathecal pretreatment with ondansetron attenuated the analgesic effect of morphine in normal rats, suggesting the involvement of the 5-HT 3 serotonergic receptor in morphine analgesia.
Local spinal mechanisms, in addition to descending inhibition, underlie the analgesic action of opioids. In the spinal cord, opioids act at synapses either presynaptically or postsynaptically. Opioid receptors are abundantly expressed in the substantia gelatinosa, where glutamate and substance P release from the primary sensory neuron is inhibited by opioids. Histamine receptors are known to participate in spinal cord nociceptive transmission, and previous studies have suggested that histaminergic receptors are also involved in the analgesic effects of morphine. An H 1 antagonist and H 3 agonist were found to potentiate the analgesic and antiedematogenic effects of morphine, suggesting that histaminergic and opioid spinal systems may be explored for means of improving analgesia, as well as peripheral antiinflammatory effects.
Opioid-receptor ligand binding has been identified in both presynaptic and postsynaptic sites in the dorsal horn of the spinal cord. It is well known that opioids decrease the pain-evoked release of tachykinins from primary afferent nociceptors. However, the degree that opioids can suppress tachykinin signaling in response to noxious stimulation remains controversial. These results suggest that although opioid administration may reduce tachykinin release from primary afferent nociceptors, the reduction may not be a major modulator on the actions of tachykinins on postsynaptic pain-transmitting neurons.
Peripheral Mechanism
Opioids may also produce analgesia through the peripheral mechanism. Immune cells infiltrating the inflammation site may release endogenous opioid-like substances, which act on the opioid receptors located on the primary sensory neuron. Interestingly, in cannabinoid receptor type 1 and type 2 knockout mice, the antinociceptive effect of morphine injected into the paw in the inflammatory phase of the formalin test was decreased by 87% and 76%, respectively. This finding may suggest the possibility that the release of endogenous cannabinoids in structures along the pain pathway is involved in opioid analgesia by the peripheral mechanism.
Acupuncture
Acupuncture and electroacupuncture have been shown to block pain by activating a variety of bioactive chemicals through peripheral, spinal, and supraspinal mechanisms. Mechanistically, endogenous opioids and opioid receptors have been shown to be involved in acupuncture- and electroacupuncture-induced analgesia in various pain models. Studies in carrageenan-induced inflammatory rat pain models show that an intraplantar injection of naloxone or selective antagonists against μ-, δ-, or κ-opioid receptors 1 hour before electroacupuncture treatment at acupoint Zusanli (ST36) dose-dependently blocked electroacupuncture-produced inhibition of mechanical hyperalgesia assessed through paw-pressure threshold. In a capsaicin-induced inflammatory hind paw pain model, stimulation by 2 Hz of four-train pulses with 100 Hz of intratrain frequency at Houxi (SI3) and San-yangluo (TE8) on the forelimb significantly raised the mechanical pain threshold of the injected paw. This analgesic effect was blocked by intrathecal μ- or δ-opioid receptor antagonists but not by a κ-opioid receptor antagonist. Precise mechanisms for acupuncture and electroacupuncture, including the involvement of physiologic systems other than the opioid receptors, remain to be elucidated.
Mechanism of Mood Alterations and Rewarding Properties
The mechanisms by which opioids produce euphoria, tranquility, and other alterations of mood (including rewarding properties) remain an area of active investigation, especially given the expanding scope of opioid diversion and misuse. Behavioral and pharmacologic evidence points to the role of dopaminergic pathways, particularly involving the nucleus accumbens (NAcc), in drug-induced reward. Functional magnetic resonance imaging studies demonstrated that a small intravenous dose (4 mg) of morphine induces positive signal changes in reward structures, including the NAcc, sublenticular extended amygdala, orbitofrontal cortex, and hippocampus, and decreases signal in cortical areas similar to the action of sedative-hypnotics such as propofol and midazolam. These observations are consistent with results of pharmacologic studies.
The shell of the NAcc is the site that may be involved directly in the emotional and motivational aspects of drug-induced reward. All three opioid receptor types are present on the NAcc and are thought to mediate, at least in part, the motivational effects of opioid drugs. Selective μ- and δ-receptor agonists are rewarding when defined by place preference and intracranial self-administration paradigms. Conversely, selective κ-receptor agonists produce aversive effects. Positive motivational effects of opioids are partially mediated by dopamine release at the level of the NAcc.
The locus ceruleus contains both noradrenergic neurons and high concentrations of opioid receptors and is postulated to play a critical role in feelings of alarm, panic, fear, and anxiety. Neural activity in the locus ceruleus is inhibited by both exogenous opioids and endogenous opioid peptides.
Analysis of Knockout Mice
The physiologic roles of the opioid receptors and endogenous opioid peptides have been investigated mainly by pharmacologic and physiologic methods. However, it has been difficult to analyze functional roles of these proteins. With analysis of knockout mice, in which a specific gene is inactivated by molecular biologic methods, additional insight into the physiologic role of the respective opioid receptors and/or endogenous opioid peptide precursors can be determined.
In the μ-receptor knockout mice, analgesia, the reward effect, and the withdrawal effect of morphine were lost. Morphine-induced respiratory depression was not observed in the μ-receptor knockout mice. Therefore, the μ-receptor is a mandatory component of the opioid system for morphine action. In the μ-receptor knockout mice, ketamine-induced respiratory depression and antinociception are diminished, a finding suggesting that ketamine interacts with the μ receptor to lead to these phenomena. Furthermore, minimum alveolar concentration (MAC) of sevoflurane was significantly higher in the μ-receptor knockout mice than wild-type mice, an observation suggesting the involvement of the μ receptor in the anesthetic potency of sevoflurane. The δ-receptor knockout mice displayed a markedly reduced analgesic effect of opioids selective for δ-receptors at the spinal cord level. However, at the supraspinal level, analgesia could be induced by δ-receptor agonists in δ-receptor knockout mice, a finding suggesting the existence of a second δ-like analgesic system. Disruption of the κ receptor abolished the analgesic, hypolocomotor, and aversive actions of the κ-receptor agonists and induced hyperreactivity in the abdominal constriction test; this finding indicates that the κ receptor is involved in the perception of visceral chemical pain. Using gene knockout mice, a pharmacologic study demonstrated that the antinociceptive effect of N 2 O may not be mediated by μ-receptor ; rather N 2 O exerts its antinociceptive action and reduces MAC of volatile anesthetics in mice through complex mechanisms including activation of the κ receptor and the descending inhibitory pathway in the spinal cord, whereas its hypnotic potency is not dependent on κ-receptor activation.
In the mice lacking β-endorphin, morphine induced normal analgesia, but naloxone-reversible stress-induced analgesia could not be observed. The preproenkephalin knockout mice were more anxious than wild-type mice, and males displayed increased offensive aggressiveness. The mutant mice showed marked difference from controls in supraspinal, but not in spinal, responses to painful stimuli.
Thus, the functional roles of individual components of the opioid system continue to be elucidated by analysis of knockout mice. The μ-opioid receptor continues to be recognized as a predominant signaling receptor for both the affective and antinociceptive action of opioid agonism.
Actions of Opioids on Targets Other than Opioid Receptors
Molecular pharmacologic analyses have shown that opioids can interact with molecules other than the opioid receptors. In cardiac myocytes, morphine can inhibit voltage-dependent sodium (Na + ) current in a naloxone-insensitive manner, a finding suggesting the existence of a signal transduction mechanism that is not dependent on the opioid receptors. Buprenorphine, a partial μ-opioid receptor agonist, also has a local anesthetic property and blocks voltage-gated Na + channels through the local anesthetic binding site. Meperidine is an agonist of both μ and κ receptors. In addition, meperidine can block voltage-dependent Na + channels in amphibian peripheral nerves, as well as in the Xenopus oocyte expression system. Furthermore, meperidine exerts agonist activity at the α 2B -adrenoreceptor subtype. Yamakura and associates have shown that high concentrations of opioids, including meperidine, morphine, fentanyl, codeine, and naloxone, directly inhibit the N -methyl- d -aspartate (NMDA) receptor expressed in Xenopus oocytes. Methadone is clinically used as a racemic mixture of the l and d isomers. The opioid-like activity of the racemate seems to be almost entirely the result of l -methadone, whereas d -methadone acts as an NMDA antagonist. The commercially available remifentanil solution (Ultiva), which contains glycine, directly activates the NMDA receptor expressed in Xenopus oocytes. Furthermore, an electrophysiologic study in rat spinal cord showed that remifentanil hydrochloride does not directly activate NMDA receptors, the NMDA current recorded after application of Ultiva is related to the presence of glycine, and the glycine-induced NMDA current is potentiated by application of remifentanil hydrochloride through a pathway involving the μ-opioid receptor. The serotonin type 3A (5-HT 3A ) receptor, which is directly and indirectly linked to gastrointestinal motility, visceral pain, nausea, and vomiting, is competitively inhibited by morphine and hydromorphone, as well as naloxone; however, fentanyl-like opioids did not significantly affect activity of the 5-HT 3A receptor. The mechanism for tramadol analgesia is complex and appears to be composed of two actions that include reuptake inhibition of the noradrenergic serotonergic system and activation of the μ-opioid receptor. Tramadol’s analgesic effect is only partially reversed by the μ-opioid receptor antagonist, naloxone. Furthermore, tramadol also acts as an agonist of transient receptor potential vanilloid-1 (TRPV1) heterologously expressed in cultured cells. Tramadol may activate TRPV1 in sensory neurons, followed by local release of vasoactive neuropeptides and marked desensitization of the afferent fibers. Inhibition of the nicotinic acetylcholine receptor by tramadol has also been reported. It remains to be clarified which of these “off-target” actions of opioids have physiologic or clinical implications.
Physiologic Role of Nociceptin/Orphanin FQ
Nociceptin or orphanin FQ is a 17-amino-acid peptide, the sequence of which resembles those of opioid peptides. Nociceptin or orphanin FQ precursor mRNA and peptide are present throughout the descending pain control circuits. In the spinal cord, nociceptin or orphanin FQ-receptor mRNA expression is stronger in the ventral horn than in the dorsal horn, but higher levels of ligand binding occur in the dorsal horn. Targeted disruption of the nociceptin or orphanin FQ receptor in mice had little effect on basal pain sensitivity in several measures, whereas targeted disruption of the N nociceptin or orphanin FQ precursor consistently elevated basal responses in the tail-flick test, findings suggesting an important role for nociceptin or orphanin FQ in regulating basal pain sensitivity. Intrathecal injections of nociceptin or orphanin FQ have been shown to be analgesic ; however, supraspinal administration has produced hyperalgesia, antiopioid effects, or a biphasic hyperalgesic-analgesic response. Nociceptin or orphanin FQ inhibits both pain-facilitating and analgesia-facilitating neurons in the RVM. The effects of nociceptin or orphanin FQ on pain responses appear to depend on the preexisting state of pain in the animal. Involvement of nociceptin or orphanin FQ in physiologic functions such as regulation of feeding, body weight homeostasis, and stress response, and in psychiatric disorders such as depression, anxiety, and drug or alcohol dependence has also been reported.
Systemic administration of nonpeptide nociceptin or orphanin FQ receptor agonists revealed that such compounds were effective analgesics in animal pain models. It was reported that a nociceptin or orphanin FQ receptor agonist, Ro64-6198, has antinociceptive and antiallodynic potency and efficacy without side effects of opioids such as itching, respiratory depression, and addiction in monkeys. As nociceptin or orphanin FQ and opioid receptor agonists modulate pain by different targets, combining both mechanisms may constitute a novel approach for the development of innovative analgesics. Cebranopadol, a newly synthesized compound possessing nociceptin or orphanin FQ and opioid receptor agonist activity, exhibits highly potent and efficacious antinociceptive and antihyperalgesic effects in several rat models of acute and chronic pain (tail-flick, rheumatoid arthritis, bone cancer, spinal nerve ligation, diabetic neuropathy). In a phase 1 clinical trial, it was shown that cebranopadol produced respiratory depression with a ceiling effect, in contrast to apnea produced by full μ-opioid receptor agonists.
Expression of opioid receptors on peripheral blood mononuclear cells is controversial. Williams and associates reported that human peripheral blood mononuclear cells express the nociceptin receptor, but not μ-, δ-, or κ-opioid receptors. Nociceptin, produced by the peripheral blood mononuclear cells, is involved in the control of immune functions.
Neurophysiologic Effects of Opioids
Characteristics of The Analgesic Action of Opioids
In human beings, morphine-like drugs produce analgesia, drowsiness, changes in mood, and mental clouding. A significant feature of opioid analgesia is that it is not typically associated with loss of consciousness. When morphine in the same dose is given to a normal, pain-free individual, the experience may be unpleasant. The relief of pain by morphine-like opioids is relatively selective, in that other sensory modalities are not affected. Patients frequently report that the pain is still present, but that they feel more comfortable. It is also important to distinguish between pain caused by stimulation of nociceptive receptors and transmitted over intact neural pathways (nociceptive pain) and pain that is caused by damage to neural structures, often involving neural supersensitivity (neuropathic pain). Although nociceptive pain usually is responsive to opioid analgesics, neuropathic pain may respond poorly to opioid analgesics and require higher doses of drug.
The analgesic effects, as well as side effects, of opioids vary greatly among individuals. A pharmacogenomic twin study has shown that the interindividual variations in opioid effects are likely the result of genetic and environmental factors. Animal and human studies indicate the existence of sex-related differences in opioid-mediated behavior. Sarton and associates examined the influence of morphine on experimentally induced pain in healthy volunteers, and demonstrated the gender differences in morphine analgesia, with greater morphine potency but slower speed of onset and offset in women. In contrast, in a study examining interindividual variability in alfentanil analgesic sensitivity in experimental pain models in humans, no gender differences were detected. In a study examining the effects of genetic factors on pain sensitivity, it was shown that males had higher pain thresholds in both thermal skin pain and muscle pressure pain than females. The mechanism of the gender difference in pain sensitivity and opioid effects remains to be clarified.
The pharmacokinetic and pharmacodynamic characteristics of opioids often vary throughout the day, as demonstrated for oral morphine in chronic pain. The duration of intrathecal sufentanil analgesia exhibited a temporal pattern, with 30% variation throughout the day in women in the first stage of labor. Scavone and colleagues reported that the time of day of administration does not seem to influence duration of labor analgesia with spinal-epidural administration of fentanyl or with systemic hydromorphone administration. The potential impact of chronobiology on clinical practice is not clear, and research on the influence of circadian rhythms on the actions of opioids is warranted.
Some controversy remains regarding analgesia produced by peripheral actions of opioids. One review concluded from meta-analysis that intraarticularly administered morphine has a definite but mild analgesic effect. It may be dose dependent, and a systemic effect cannot be completely excluded. The addition of opioids in brachial plexus block improves the success rate and postoperative analgesia. In contrast, the addition of sufentanil may not prolong the duration of brachial plexus block.
Despite variations in effect ascribed to the type of pain stimuli, genetics, gender, time of administration, or site of action (central versus peripheral), opioids remain one of the most potent classes of analgesic drugs.
Effects of Opioids on Consciousness
Cortical acetylcholine originates in the basal forebrain and is essential for maintaining normal cognition and arousal. Injection of morphine to the substantia innominata or intravenous morphine administration significantly decreased acetylcholine release within the prefrontal cortex in the rat, and this effect may be the neurochemical basis of opioid-induced change in consciousness. Although unconsciousness in humans can be produced with high doses of opioids alone, opioid-based anesthesia can be unpredictable and inconsistent. Therefore, opioids are not suitable as sole intravenous induction agents. The anesthetic potential of opioids was tested by measurement of MAC. Fentanyl can reduce the MAC of isoflurane at skin incision in patients by at least 80%. The relationship between the plasma fentanyl concentration and reduction in MAC is not linear, and fentanyl has a sub-MAC ceiling effect on reduction of the MAC of isoflurane. The MAC of sevoflurane was also dose dependently reduced by fentanyl, with 3 ng/mL resulting in a 61% reduction in MAC. A ceiling effect was observed for MAC; 6 ng/mL fentanyl provided only an additional 13% reduction in the MAC of sevoflurane. Even potency ratios have been established for the “reduction of volatile anesthetic MAC” for most opioids such as sufentanil, fentanyl, remifentanil, and alfentanil. However, the MAC-reducing capabilities of the opioids are not complete; that is, these are not complete anesthetics. The opioids must be combined with other anesthetics to produce a “complete anesthetic.” Esmolol, a short-acting β 1 -receptor antagonist, significantly decreased the MAC of isoflurane in the presence of alfentanil, although it did not significantly affect this parameter in the absence of alfentanil. The mechanism of the interaction is unknown. Epidural fentanyl infusion reduces the awakening concentration of isoflurane more than does intravenous fentanyl infusion despite the lower plasma concentration, possibly by modulating the afferent nociceptive inputs in the spinal cord.
The MAC that prevents movement in response to laryngoscopy and tracheal intubation in 50% of patients (MAC-TI) is higher than the MAC that prevents movement in response to surgical incision (MAC). The MAC-TI of sevoflurane was 3.55% and was reduced markedly to 2.07%, 1.45%, and 1.37% by the addition of fentanyl 1, 2, and 4 μg/kg, respectively, with no significant difference in the reduction between 2 and 4 μg/kg, thus showing a ceiling effect. The MAC that prevents sympathetic responses to surgical incision in 50% of patients (MAC-BAR) decreased with increasing concentrations of fentanyl in plasma, and the initial steep reduction followed by a ceiling effect.
The bispectral index (BIS) is often used to determine the level of consciousness under anesthesia. In the presence of fentanyl, alfentanil, remifentanil, or sufentanil, loss of consciousness occurred at a lower effect-site concentration of propofol and at a higher BIS value as compared with propofol alone. Furthermore, Wang and associates reported that infusion of remifentanil (0.1-0.4 μg/kg/min) did not significantly change the median effective concentration (EC 50 ) of propofol necessary to lower the BIS value to 50 or less. These results suggest that the hypnotic effect of propofol is enhanced by analgesic concentrations of opioids without changes in the BIS value. Conversely, infusion of remifentanil (effect-site target concentrations of 0.25, 2.5, and 10 ng/mL), combined with propofol infusion adjusted to a BIS of approximately 60, dose dependently decreased the BIS, thus suggesting a sedative or hypnotic effect of remifentanil. Response surface analysis showed considerable synergy between opioids and hypnotics for sedation and suppression of responses to various noxious stimuli. In contrast to the effect of opioids administered during anesthesia on the BIS, the impact of chronic opioid use on BIS is unknown. It was recently reported that the end-tidal concentration of sevoflurane necessary to maintain the BIS under 50 was 0.84% for chronic opioid users who received a stable dose of oral morphine of at least 60 mg/day according to the morphine equivalent daily dose for at least 4 weeks, which was lower than for opioid-naïve patients (1.18%).
Opioids administered as a mainstay of postsurgical pain management can inhibit sleep on the first night after surgery. However, the effects of opioids on sleep and circadian rhythm are not clearly understood. A human study demonstrated that an overnight constant infusion of remifentanil inhibited rapid eye movement sleep without suppressing the nocturnal melatonin surge, findings that may suggest a minimal effect of opioids on the circadian pacemaker.
Taken together, perioperative opioids have consistently been shown to dose dependently reduce the MAC and are well known to synergize with hypnotics to produce sedation; however, their ability to effect the BIS may vary depending on the context of opioid administration.
Hallucination
Opioid-induced hallucination is an uncommon yet significant adverse effect of opioid treatment, frequently attributed to underlying psychiatric disease or personality disorder rather than a direct neurobiologic effect of opioids. Hallucinations attributed to opioids have been typically described as auditory, visual, or rarely, tactile hallucinations. Although many reports have cited morphine as the causative agent, there is no evidence that across a population, one particular class of opioid is associated with a lower incidence of hallucinations. Moreover, there are reports of fentanyl, methadone, tramadol, hydromorphone, buprenorphine, pentazocine, and/or oxycodone–associated hallucinations and/or changes in mental status. Nevertheless, accumulation of morphine metabolites, particularly morphine-3-glucoronide, has been linked to the development of neurologic phenomena. Many hypotheses have been advanced to explain the etiology of opioid-induced hallucinations. One common feature of these hypotheses involves opioid-induced dopamine dysregulation. Overactivation of the dopaminergic pathways is thought to result in auditory and visual hallucinations. The simplest treatment for opioid-induced hallucination is discontinuing opioid therapy if practical. There are reports describing the successful use of naloxone and κ-selective opioid antagonists in the treatment of hallucinations associated with schizophrenia, although such κ-selective agents are known to be associated with hallucinations in subjects or patients without a diagnosis of mental illness.
Electroencephalography
Increasing concentrations of inhaled anesthetics produce a continuum of electroencephalogram (EEG) changes and eventually result in burst suppression and a flat EEG. In contrast, a ceiling effect is reached with opioids. Once this ceiling has been obtained, increasing opioid dosage does not further affect the EEG.
Although the potency and rate of equilibrium between plasma and brain are different among opioids, the effects of fentanyl, alfentanil, sufentanil, and remifentanil are consistent ( Fig. 24.9 ). Small doses of fentanyl (2-5 μg/kg) produce minimal EEG changes, whereas higher doses (30-70 μg/kg) result in high-voltage slow (δ) waves suggesting a state consistent with anesthesia. Although transient isolated (usually frontotemporal) sharp wave activity can be observed after large doses of fentanyl and other opioids, it is not generalized. In a study examining the effect of morphine (3-10 mg) on EEG in patients (14.8 ± 2.8 years) who stayed overnight for pain relief after otologic surgery, it was demonstrated that morphine reduced high-frequency β 1 (13.5-20 Hz) and β 2 (20-30 Hz) EEG powers and decreased coherence between frontal and occipital β 2 EEG activities compared to wakefulness and non–rapid eye movement sleep, indicating that morphine induced a deep sedative state ( Fig. 24.10 ). Khodayari-Rostamabad and associates studied the effect of remifentanil administration on resting EEG functional connectivity and its relationship to cognitive function and analgesia in healthy volunteers. Remifentanil administration was associated with significant changes in functional cortical connectivity, which seem to disrupt the complex cortical network subserving normal brain function and can have the potential to be a biomarker for the sedative effects of opioids.


As an effect-site measure, EEG can be employed to assess onset of drug action and drug potency ratios. The spectral edge and serum concentration are closely parallel for remifentanil, whereas a significant time lag is found for recovery of the spectral edge for fentanyl and sufentanil ( Fig. 24.11 ). In healthy volunteers, approximate entropy derived from a parietal montage showed significant correlation with remifentanil concentration, and it was shown to be appropriate for the assessment of the remifentanil effect on the EEG. Potency ratios based on EEG studies are similar to those obtained from studies determining the plasma drug levels of each opioid necessary to reduce the MAC of isoflurane by 50%. Overall, opioids produce dose-dependent changes in the EEG that can mimic those of volatile anesthetics, however opioids show a ceiling effect at higher doses.

Evoked Responses
Because opioids do not appreciably alter sensory-evoked potentials elicited at the posterior tibial or median nerve, sensory-evoked potentials can be used for spinal cord function monitoring during anesthesia using opioids. Although remifentanil produced dose-dependent reduction in auditory-evoked potentials, it was also reported that remifentanil infusion (target plasma concentrations of 1, 2, and 3 ng/mL) did not affect evoked potential amplitudes and latencies. In healthy human volunteers receiving 3 μg/kg fentanyl, amplitude and latency of motor-evoked responses to transcranial stimulation were not significantly affected. This is consistent with the report by Kawaguchi and colleagues that intraoperative myogenic motor-evoked potential monitoring is feasible during isoflurane or sevoflurane anesthesia with fentanyl.
Middle latency auditory-evoked potentials (MLAEPs) and their derivatives are increasingly used as a surrogate measure of the level of anesthesia. Changes in MLAEPs following the administration of opioids occur and may result from either a direct depressant effect of opioids on MLAEPs itself or an indirect effect reflecting the action of opioids in attenuating CNS arousal associated with noxious stimuli. Wright and associates examined the effect of remifentanil (1 or 3 μg/kg/min) on MLAEPs in tracheally intubated and nonintubated patients and showed that remifentanil has an effect on MLAEPs in attenuating the arousal associated with tracheal intubation but had no effect in the absence of a stimulus. Similarly, Schraag and associates found no significant contribution of remifentanil alone on MLAEPs, whereas increasing the concentration of remifentanil led to a significant decrease of the calculated propofol effect-site concentrations necessary for unconsciousness.
Cerebral Blood Flow and Cerebral Metabolic Rate
Opioids generally produce modest decreases in cerebral metabolic rate and intracranial pressure (ICP), although the changes are influenced by the concomitant administration of other agents and anesthetic drugs, as well as by the patients’ conditions. When vasodilation is produced by coadministered anesthetics, opioids are more likely to cause cerebral vasoconstriction. Opioids also decrease cerebral blood flow (CBF) when they are combined with nitrous oxide (N 2 O). When opioids are administered alone or when the coadministered anesthetics cause cerebral vasoconstriction, opioids usually have no influence or result in a small increase in CBF.
Endogenous opioid activity is present in the cerebral arteries, although exogenously administered opioids were found to exert little effect on pial artery diameter in several animal models. In the piglet, fentanyl, alfentanil, and sufentanil decreased arteriolar diameter in a dose-dependent naloxone-reversible manner. In human volunteers, PET demonstrated that CBF changes induced by fentanyl are regionally heterogenous.
In healthy volunteers, sufentanil (0.5 μg/kg intravenously [IV]) produces no significant effect on CBF. Alfentanil (25-50 μg/kg IV), administered to patients receiving isoflurane (0.4%-0.6%)-N 2 O anesthesia, produces minimal reductions in middle cerebral artery flow velocity. A PET study in human volunteers showed that remifentanil induced dose-dependent changes in relative regional CBF in areas involved in pain processing, such as the lateral prefrontal cortex, inferior parietal cortex, and supplementary motor area. In patients scheduled to undergo a supratentorial tumor surgical procedure and receiving N 2 O, remifentanil (1 μg/kg/min), similar to fentanyl (2 μg/kg/min), reduced CBF and did not significantly affect cerebrovascular carbon dioxide (CO 2 ) reactivity.
Opioid-induced neuroexcitation and focal seizure activity can cause regional increases in brain metabolism. Regional increases in glucose use induced by high doses of alfentanil in the rat were associated not only with epileptiform activity but also with neuropathic lesions. In humans, PET evaluation demonstrated that a 1 to 3 μg/kg/min infusion of remifentanil induces significant increase in the cerebral metabolic rate for glucose. In summary, opioids, in general, do not significantly effect measures of CBF.
Intracranial Pressure
Opioids are generally thought to affect ICP minimally under conditions of controlled ventilation. When given with background anesthesia (isoflurane-N 2 O), opioids do not cause significant increases in ICP undergoing craniotomy for supratentorial space-occupying lesions. Opioid sedation does not alter ICP in patients with head injuries. Light sedation with remifentanil (4.2 ± 1.8 μg/kg/h) does not result in higher ICP than that observed with propofol (4.3 ± 2.5 mg/kg/h) in patients undergoing stereotactic brain tumor biopsy, and cerebral perfusion pressure might be better preserved with remifentanil.
Opioids may produce increases in ICP in patients undergoing craniotomy for excision of supratentorial space-occupying lesions, especially if intracranial compliance is compromised. In a study of patients with severe head injury who have preserved and impaired autoregulation, morphine (0.2 mg/kg) and fentanyl (2 μg/kg) moderately increased ICP, a finding suggesting that mechanisms other than vasodilation could be implicated in the opioid-induced ICP elevation. Investigators also demonstrated no change in ICP in hydrocephalic children after administration of alfentanil (70 μg/kg). Whether these discrepancies of the opioid effects on ICP reflect pressure assessment methods or the effects of other drugs is not clear. If opioids do increase ICP, whether cerebrovascular dilatation is directly induced by opioids or indirectly resulted from opioid-induced decrease in blood pressure is not known.
Neuroprotection
Although certain early studies suggested potentially adverse effects of μ-opioid agonists on ischemic brain, other studies documented that certain opioid agents, such as the κ-agonists, can be neuroprotective in animal models of focal ischemia. Investigators also showed that activation of δ-opioid receptors increases survival time of mice during lethal hypoxia. An in vitro study using rat cerebellar brain slices demonstrated that pretreatment with morphine at clinically relevant concentrations induced acute neuroprotection mediated by activation of the δ 1 -opioid receptors, activation of adenosine triphosphate (ATP)-sensitive K + channels, and free radical production in mitochondria. In the rat focal ischemia model, fentanyl neither increased nor decreased brain injury compared with awake unanesthetized rats. It was demonstrated that at a supraclinical concentration remifentanil had no pronecrotic effect but exerted ex vivo antiapoptotic action on the immature mouse brain, involving the opioid and NMDA receptors, and the mitochondrial-dependent apoptotic pathway. A recent study reported that κ-opioid receptors were upregulated and played a critical role in brain ischemia and reperfusion in mice, and that κ-opioid receptor activation could potentially protect the brain and improve neurologic outcome via blood-brain barrier protection, apoptosis reduction, and inflammation inhibition. Although there is contradicting evidence for a potential neuroprotection in animal models, there is no clear evidence for neuroprotective effects in humans.
Muscle Rigidity
Opioids can increase muscle tone and may cause muscle rigidity. The incidence of rigidity noted with opioid anesthetic techniques varies greatly because of differences in dose and speed of opioid administration, the concomitant use of N 2 O, the presence or absence of muscle relaxants, and the patient’s age. Opioid-induced rigidity is characterized by increased muscle tone that sometimes progresses to severe stiffness and associated clinical challenges ( Table 24.4 ). Clinically significant opioid-induced rigidity usually begins just as, or after, a patient loses consciousness. Mild manifestations of rigidity, such as hoarseness, can occur in conscious patients. Vocal cord closure is primarily responsible for the difficult ventilation with bag and mask that follows the administration of opioids. Although opioids are generally thought to affect ICP minimally, it was demonstrated that alfentanil-induced rigidity can cause increase in ICP in rats. Delayed or postoperative rigidity may be related to second peaks that can occur in plasma opioid concentrations, similar to the recurrence of respiratory depression.
System | Problem |
---|---|
Hemodynamic | ↑CVP, ↑ PAP, ↑ PVR |
Respiratory | ↓ Compliance, ↓ FRC, ↓ ventilation |
Hypercarbia, Hypoxemia | |
Miscellaneous | ↑ Oxygen consumption, ↑Intracranial pressure, |
↑ Fentanyl plasma levels |
The precise mechanism by which opioids cause muscle rigidity is not clearly understood. Muscle rigidity is not the result of a direct action on muscle fibers because it can be decreased or prevented by pretreatment with muscle relaxants. Mechanisms of opioid-induced muscle rigidity involving the CNS have been postulated. The nucleus raphe pontis within the reticular formation and the caudate nucleus within the basal ganglia have been implicated mechanistically. Pharmacologic investigation using selective agonists and antagonists suggests that systemic opioid-induced muscle rigidity is primarily caused by the activation of central μ-receptors, whereas supraspinal δ 1 and κ 1 receptors may attenuate this effect. Some aspects of opioid-induced catatonia and rigidity (increased incidence with age, muscle movements resembling extrapyramidal side effects) are similar to Parkinson disease and suggest similarities in neurochemical mechanisms. Patients with Parkinson disease, particularly if they are inadequately treated, may experience reactions such as dystonia following opioid administration.
Pretreatment with or concomitant use of nondepolarizing muscle relaxants can decrease the incidence and severity of rigidity. Opioid-induced muscle rigidity can also be reversed with the μ-receptor antagonist naloxone. Induction doses of sodium thiopental and subanesthetic doses of diazepam and midazolam can prevent, attenuate, or successfully treat rigidity.
Neuroexcitatory Phenomena
Fentanyl causes seizure activity seen on the EEG in animals, but EEG evidence of seizure activity after fentanyl, alfentanil, or sufentanil is generally lacking in humans. Remifentanil induced generalized tonic-clonic seizure-like activity in an otherwise healthy adult. Morphine produces tonic-clonic activity after epidural and intrathecal administration. Focal neuroexcitation on the EEG (e.g., sharp and spike wave activity) occasionally occurs in humans after large doses of fentanyl, sufentanil, and alfentanil.
The mechanisms underlying opioid-induced neuroexcitatory phenomena are not completely clear. Excitatory opioid actions may be related to coupling to mitogen-activated protein kinase cascades. Local increases in CBF and metabolism are also of theoretic concern because prolonged seizure activity, even if focal, could lead to neuronal injury and/or cellular death. Fentanyl, alfentanil, and sufentanil in large doses also induced hypermetabolism and histopathologic alterations of the limbic system in rats. Experimental study using excised mouse hippocampus showed that the proseizure effect of morphine is mediated by selective stimulation of the μ- and κ-opioid receptors but not the activation of the δ-opioid receptor. In rats, midazolam, naloxone, and phenytoin prevented EEG seizure activity and histologically evident brain damage induced by large doses of fentanyl.
Measurement of CBF by magnetic resonance imaging in human volunteers indicated that the cingulate cortex is the most susceptible to activation by remifentanil (0.05-0.2 μg/kg/min), and susceptibility is affected by the apolipoprotein E genotype. These findings support the notion that neuroactivation of limbic areas by the perioperative use of opioids may have a role in the genesis of postoperative cognitive dysfunction.
Pupil Size
Morphine and most μ− and κ−agonists cause constriction of the pupil by an excitatory action on the parasympathetic nerve innervating the pupil. Light induces excitation of the Edinger-Westphal nucleus leading to pupillary constriction, which is inhibited by hypercarbia, hypoxia, and nociception. Opioids release the effect of inhibitory neurons on the Edinger-Westphal nucleus, resulting in papillary constriction ( Fig. 24.12 ). After intravenous administration of morphine (0.125 mg/kg) in one report, a 26% decrease in pupil diameter occurred at 1 hour, and a period of more than 6 hours was necessary for complete recovery of pupil diameter. The pupillary dilatation reflex has been successfully used to assess the analgesic component of a balanced anesthetic regimen. The pupillometer may be a valuable tool to guide morphine administration in the immediate postoperative period. A prospective randomized study evaluated the impact of intraoperative pupillometry monitoring on perioperative opioid consumption in major gynecologic surgery. It was demonstrated that the use of pupillometry to guide intraoperative analgesia reduced intraoperative remifentanil consumption and postoperative morphine requirements. Pupillary unrest is the fluctuation in pupil diameter, and pupillary unrest under ambient light (PUAL) is present even in well-rested individuals. Although the underlying mechanisms are unknown, PUAL is depressed by opioids. Administration of fentanyl to healthy volunteers diminishes PUAL, and the decrease is proportionally larger than the change in pupil diameter. The pretreatment magnitude of PUAL is correlated with the analgesic response to opioid therapy, and patients who exhibit higher levels of PUAL change after opioid administration have a more beneficial analgesic effect from opioids.

Pupillary constriction represents a well-known phenomenon following opioid administration and the use of pupillometry may help guide intraoperative opioid dosing to optimize analgesia.
Thermoregulation and Shivering
Opioid-based anesthesia probably reduces thermoregulatory thresholds to a degree similar to that of the potent inhaled anesthetics. However, meperidine is unique among opioids in its ability to effectively terminate or attenuate shivering. The antishivering effect of meperidine is primarily related to a reduction in the shivering threshold, and seems to be mediated by meperidine’s activity on the κ receptor. However, the relatively specific κ-receptor agonist, nalbuphine, did not show significant antishivering activity. Meperidine exerts agonist activity at the α 2B -adrenoreceptor subtype, a finding suggesting the involvement of this action in the antishivering action of meperidine. Alfentanil, morphine, and fentanyl are not as effective as meperidine in the treatment of postoperative shivering. However, alfentanil and nefopam, a centrally acting analgesic, additively reduce the shivering threshold in humans. Tramadol (0.5 mg/kg) suppressed postepidural anesthetic shivering in parturients as effectively as meperidine (0.5 mg/kg). A quantitative systematic review of randomized controlled trials found that meperidine 12.5 to 35 mg and tramadol 35 to 220 mg were more effective than control for parenteral pharmacologic interventions for the prevention of postoperative shivering.
Remifentanil is associated with an increased incidence of postanesthetic shivering, which is not related with intraoperative hypothermia. The higher incidence of postanesthetic shivering with higher doses of remifentanil probably reflects acute opioid tolerance and stimulation of the NMDA receptors. A small dose of ketamine, 0.5 mg/kg at induction of anesthesia followed by infusion at 0.3 mg/kg/hour, can prevent remifentanil-induced postanesthetic shivering.
Pruritus
Opioid-induced pruritis is one of several perpetual challenges associated with opioid-based analgesia. With the possible exception of morphine, histamine release, once thought to underlie this phenomenon, is not causative because non-histamine-releasing opioids also produce pruritus. Both central and peripheral nervous system mechanisms have been explored. Facial itching may not necessarily be a manifestation of direct opioid action at the level of the trigeminal nucleus, but rather, it may be a reflection of opioid-triggered neural transmission at a distant site. It is not known why the face is prone to pruritus even after spinal opioids. Interestingly, pruritus due to cholestasis is ameliorated by opioid antagonists. Intrathecal morphine-induced itching in monkeys was suggested to be mediated by the μ receptor. Activation of a μ-opioid receptor isoform MOR1D by morphine induced activation of the gastrin-releasing peptide receptor that heterodimerized with MOR1D, thus resulting in activation of phospholipase β3 and intracellular Ca 2+ increase in neurons leading to itch sensation in mice.
Naloxone reverses opioid-induced itching, but opioid antagonists are not ideal therapeutic agents against pruritus because opioid analgesia is often reversed by these agents. A subcutaneous administration (12 mg) of methylnaltrexone bromide, a peripherally acting μ-opioid antagonist, did not reduce the overall severity or incidence of pruritus in women having elective cesarean section under spinal anesthesia with intrathecal morphine 100 μg, suggesting that peripheral mechanisms do not significantly contribute to spinal opioid-induced pruritus. Ondansetron, a serotonin 5-HT 3 receptor antagonist has been proposed to treat spinal or epidural morphine-induced pruritus, and a metaanalysis has demonstrated that prophylactic use of 5-HT 3 antagonists significantly reduced the severity and the need for treatment of pruritus. Another meta-analysis showed that prophylactic intravenous administration of 8 mg ondansetron does not decrease the incidence of fentanyl- or sufentanil-induced pruritus but may decrease the need for pruritus rescue medication. The application of mixed or partial opioid agonists, such as nalbuphine and butorphanol, are growing in popularity as antipruritics because they may partially antagonize μ receptor function with intact κ actions to maintain analgesia. In fact, activation of the κ-opioid receptor inhibits pruritus evoked by subcutaneous and intrathecal morphine in animal models.
Other agents that have been investigated include pentazocine (15 mg), an agonist of the κ-opioid receptor and partial agonist of the μ-opioid receptor. Pentazocine has been found to be superior to 4 mg of ondansetron for the treatment of pruritus induced by intrathecal morphine in parturients undergoing cesarean delivery. It was recently reported that the antipruritic effect of κ-receptor agonists may not require interaction between the κ receptor and β-arrestins. Tenoxicam, a nonsteroidal antiinflammatory drug (NSAID), was reported to be effective for pruritus induced by epidural fentanyl. Other approaches include intravenous administration of droperidol (1.25 mg), propofol (20 mg), or alizapride (100 mg), which reduced the incidence of pruritus induced by the use of morphine 0.2 mg intrathecally in patients undergoing cesarean section under spinal anesthesia. Preoperative gabapentin prevents pruritus induced by intrathecal morphine in patients undergoing lower limb surgery with spinal anesthesia.
As opioid-induced pruritis continues to be a clinical challenge, current strategies have shifted away from the consequences of histamine release (morphine) and are focused on exploiting partial blockade/activation of the μ-opioid receptor, activation of the κ-receptor, and non-opioid receptor pathways.
Opioid-Induced Hyperalgesia
There is growing evidence that opioid-induced hyperalgesia (OIH) can represent a major adverse effect of opioid administration, especially when driven by potent formulations and escalating dosages. Experimentally, opioids have elicited hyperalgesia in animal models after repeated opioid administration or continuous delivery. A systematic review to evaluate the clinical implication of OIH demonstrated that high intraoperative doses of remifentanil are associated with small but significant increases in acute pain after surgery as assessed by: pain intensity at rest 24 hours after surgery, 24-hour morphine use, pain intensity on movement, and hyperalgesia measured after an operation. In a randomized, double-blind, crossover study with 24 healthy male volunteers, it was demonstrated that when compared with low-dose (1 μg/kg) fentanyl, high-dose fentanyl (10 μg/kg) increased the area of hyperalgesia from 4.5 to 6.5 hours after fentanyl administration. This result suggests that fentanyl can also produce OIH in humans.
OIH was shown to be due to spinal sensitization to glutamate and substance P. Activation of glycogen synthase kinase-3β (GSK-3β) contributes to remifentanil-induced hyperalgesia by regulating NMDA receptor plasticity in the spinal dorsal horn, and further demonstrated that GSK-3β inhibition prevented remifentanil-induced postoperative hyperalgesia via regulating α-amino-3-hydroxy-5-methyl-4-isoxazolepropionic acid receptor (AMPAR) expression and function in the spinal dorsal horn. Importantly, abrupt withdrawal of opioid administration may also contribute to induction of OIH, although its mechanism is not clear. In contrast to the high incidence of hyperalgesia induced after abrupt withdrawal of remifentanil (administered at 2.5 ng/mL for 30 minutes), no hyperalgesia was observed after gradual withdrawal by 0.6 ng/mL every 5 minutes.
There have been reports that OIH and subsequent acute opioid tolerance can be prevented by ketamine, suggesting the involvement of the NMDA receptor. Methadone is unique in possessing both μ-opioid receptor activating and NMDA antagonist properties. Opioid-induced hyperalgesia resulted from the presence in the racemate of the l -methadone (μ-opioid agonist) and was antagonized by the presence of the d -methadone (NMDA antagonist). Low-dose buprenorphine (25 μg/h for 24 h), an opioid with NMDA antagonist activity, in patients receiving remifentanil infusion during major lung surgery prevented postoperative secondary hyperalgesia. Butorphanol (0.2 μg/kg) was also effective for prevention of postoperative hyperalgesia after laparoscopic cholecystectomy performed with remifentanil (0.3 μg/kg/min). N 2 O, an inhalation anesthetic, is an effective NMDA antagonist. Intraoperative 70% N 2 O administration significantly reduced postoperative opioid-induced hyperalgesia in patients receiving propofol (approximately 120 μg/kg/min) and remifentanil (0.3 μg/kg/min). A randomized, double-blind, prospective study showed that a relatively high dose of remifentanil (0.2 μg/kg/min) enhances periincisional hyperalgesia in patients undergoing thyroidectomy, and intraoperative magnesium sulfate (30 mg/kg at induction followed by 10 mg/kg/h) can prevent remifentanil-induced hyperalgesia.
Other strategies have focused on the upregulation of spinal cyclooxygenase-2 (COX-2) and increased prostaglandin E2 release in the spinal cord after morphine withdrawal in rats. In humans, hyperalgesia after a 30-minute intravenous infusion of remifentanil (0.1 μg/kg/min) can be prevented by administration of parecoxib, a COX-2 inhibitor, before remifentanil infusion, suggesting the involvement of COX-2 in OIH. Alternatively, it was reported that an ultra-low dose of naloxone blocked remifentanil-induced hyperalgesia but did not change opioid tolerance in rats under inhaled anesthesia, and that the MAC increase associated with hyperalgesia was also blocked by naloxone. In patients undergoing elective thyroid surgery, intraoperative use of naloxone (0.05 μg/kg/h) reduced postoperative hyperalgesia after remifentanil infusion of 4 ng/mL.
Genetic variants of the β 2 -adrenergic receptor gene may explain some part of the differences between various strains of mice to develop OIH, and the selective β 2 -adrenergic receptor antagonist butoxamine was shown to dose dependently reverse OIH. In mice, systemic or intrathecal injection of the 5-HT 3 receptor antagonist, ondansetron, was shown to prevent or reverse opioid-induced tolerance or hyperalgesia.
Interestingly, the occurrence of OIH might be affected by general anesthetics coadministered with opioids. When women undergoing breast cancer surgery were anesthetized with sevoflurane or propofol to keep BIS value at 40 to 50, postoperative hyperalgesia induced by intraoperative remifentanil infusion (effect-site target 4 ng/mL) was significant with sevoflurane anesthesia, but not apparent with propofol anesthesia.
A follow-up study on the postoperative course of cardiac surgery demonstrated that intraoperative remifentanil was predictive for chronic thoracic pain 1 year after surgery in a dose-dependent manner. It can be anticipated that hyperalgesia during the perioperative period is linked to peripheral and central pain sensitization and thereby correlates with the development of postoperative chronic pain. This suggests that hyperalgesia due to remifentanil in the early postoperative period may explain the higher incidence of chronic pain.
Overall, human data generally support the existence of OIH in a few specific settings. Clinically relevant mechanisms of OIH include involvement of the NMDA receptor with ketamine as an appealing remedy. Although all potent opioids such as fentanyl are apparently capable of inducing OIH, ultrapotent opioid agonists such as remifentanil may pose even greater risks. The conditions under which OIH is expressed should be clarified and the extent of its clinical significance remains to be elucidated.
Respiratory Effects of Opioids
The respiratory depressant actions of opioids represent their most serious adverse effect. Although some early studies indicated the involvement of both μ- and δ-opioid receptors, activation of the μ-opioid receptor in the caudal medullary raphe region, which is important for regulating pain and respiration, inhibits the ventilatory response to hypercapnia in anesthetized rats. Furthermore, administration of morphine or M6G did not produce significant respiratory depression in the μ-opioid receptor knockout mice. Polymorphism of the μ-opioid receptor at nucleotide position 118, which is known to affect M6G-induced analgesia, does not significantly change the susceptibility of respiratory depressive effect of M6G. This result suggests that analgesia and respiratory depression may be mediated by different signal transduction mechanisms activated by the μ-opioid receptor.
Effects on Airways
The antitussive actions of opioids are well known and central in origin. Opioids blunt or eliminate somatic and autonomic responses to tracheal intubation. They allow patients to tolerate endotracheal tube placement without coughing or “bucking.” Conversely, two successive doses of 1.5 μg/kg fentanyl did not effectively prevent laryngospasm in children aged 2 to 6 years old who were anesthetized with sevoflurane. Opioids can also help avoid increases in bronchomotor tone in asthma. In addition, fentanyl also has antimuscarinic, antihistaminergic, and antiserotoninergic actions and may be more effective than morphine in patients with asthma or other bronchospastic diseases.
Nevertheless, several studies have reported a depressant effect of morphine on respiratory mucus transport, which is one of the most important defenses against respiratory tract infections. However, morphine had no effect on the beating frequency of nasal cilia in vitro. Opioids can affect pharyngeal function, airway protection, and coordination of breathing and swallowing. A sedative dose of morphine (0.1 mg/kg) was associated with increased incidence of pharyngeal dysfunction and discoordinated breathing and swallowing, a combination impairing airway protection and potentially increasing the risk for pulmonary aspirations.
More potent opioids such as remifentanil (effect-site concentration of 2 ng/mL) can suppress coughing induced by extubation after propofol or sevoflurane anesthesia. In contrast, fentanyl, sufentanil, and alfentanil curiously elicit a brief cough in up to 50% of patients when the drug is injected by intravenous bolus. Fentanyl, administered through a peripheral intravenous cannula, provoked cough when it was injected rapidly, but the incidence of cough decreased significantly as the injection time was increased, as well as by the administration of 1.5 mg/kg lidocaine 1 minute before fentanyl administration. A meta-analysis showed that the lowest effective dose of lidocaine on the risk of opioid-induced cough was 0.5 mg/kg. It was also reported that preemptive use of fentanyl 25 μg, administered 1 minute before bolus injection of fentanyl (125 or 150 μg), can effectively suppress fentanyl-induced cough. Propofol, α 2 agonists (clonidine, dexmedetomidine), inhalation of β 2 agonists (terbutaline, salbutamol), and NMDA-receptor antagonists (ketamine, dextromethorphan) were also effective for suppression of fentanyl-induced cough. A prospective randomized controlled study demonstrated that a huffing maneuver, consisting of a forced expiration against open glottis, just before intravenous fentanyl administration significantly reduced the incidence and severity of fentanyl-induced coughing in the majority of the patients. Li and associates reported that nonsmoking women undergoing gynecological surgery who develop fentanyl-induced cough during induction of anesthesia have a higher incidence of postoperative nausea and vomiting (PONV).
In summary, opioids engender potent antitussive properties that can help blunt airway responsiveness to endotracheal intubation. However, depending on the class of opioid and rate of administration, they may evoke a brief cough that can be subverted through the use of preadministered agents such as lidocaine. Ultimately, caution should be exercised in the administration of opioids as they can also interfere with protective aspects of airway physiology.
Respiratory Depression
The lack of adequate pain relief can cause shallow respiration leading to postoperative respiratory dysfunction including atelectasis, and therefore opioids can serve as a foundational component of postoperative analgesia to prevent or correct respiratory impairment. However, opioids can also dose dependently depress respiration, representing the most feared adverse effect. The incidence of opioid-induced respiratory depression varies from 0.1% to 37%, depending on the route of opioid administration, the type of opioid, the definition and method of monitoring opioid-induced respiratory depression, and the prospective versus retrospective nature of the study, and is a significant cause of death and brain damage in the perioperative period.
Opioids activating the μ receptor cause dose-dependent depression of respiration, primarily through a direct action on brainstem respiratory centers. The stimulatory effect of CO 2 on ventilation is significantly reduced by opioids. Hypercapnic responses can be separated into central and peripheral components. In one report, morphine-induced changes in the central component were equal in men and women, whereas changes in the peripheral component were larger in women. In addition, the apneic threshold and pressure of end-tidal carbon dioxide (PETCO 2 ) are increased by opioids ( Fig. 24.13 ). Opioids also decrease hypoxic ventilatory drive.

Respiratory rate is usually drastically decreased in opioid overdose, although hypoxic CNS insult can counter this effect. The prolonged expiratory time in the respiratory cycle induced by opioids frequently results in greater reductions in respiratory rate than in tidal volume. Monitoring of breath intervals can sensitively detect fentanyl-induced respiratory depression and can be used as a measure of dynamic opioid effect. High doses of opioids usually eliminate spontaneous respirations without necessarily producing unconsciousness. Patients receiving high doses of opioids may still be responsive to verbal command and often breathe when they are directed to do so.
Peak onset of respiratory depression after an analgesic dose of morphine is slower than after comparable doses of fentanyl, and respiratory depression induced by small doses of morphine usually lasts longer than after equipotent doses of fentanyl. Plasma fentanyl concentrations of 1.5 to 3.0 ng/mL are associated with significant decreases in CO 2 responsiveness. With higher doses of fentanyl (50-100 μg/kg), respiratory depression can persist for many hours. When moderately large doses (20-50 μg/kg or greater) of fentanyl are used, the potential need for postoperative mechanical ventilation should be anticipated. The effects of remifentanil are attenuated rapidly and completely within 5 to 15 minutes following termination of its administration. In healthy humans, the EC 50 for depression of minute ventilation with remifentanil and alfentanil was 1.17 ng/mL and 49.4 ng/mL, respectively. In healthy volunteers, fentanyl 1 μg/kg and remifentanil 0.5 μg/kg had similar maximum decreases in minute ventilation (∼50%), but the onset of and recovery from ventilatory depression were faster with remifentanil. Naloxone has been accepted as a standard therapy for opioid-induced respiratory depression. However, reports have noted naloxone-resistant respiratory depression after intrathecal morphine administration.
A major component of the excitatory synaptic drive necessary for respiratory rhythmogenesis and activation of respiratory motoneurons is via the amino-3-hydroxy-5-methyl-4-isoxazolepropionate (AMPA) type of glutamate receptors. This finding has led to investigations of ampakine (positive modulators of AMPA receptors) therapy to alleviate opioid-induced respiratory depression. 5-HT released from the raphe nuclei potently alters the excitability of respiratory motoneurons, the preBötzinger complex (preBötC), and other brainstem respiratory nuclei. The activation of 5-HT 1A receptors with befiradol alleviates fentanyl-induced respiratory depression in rats. PreBötC, the main region of respiratory rhythm–pattern generation, is the main target of opioid-induced respiratory depression. However, it has been demonstrated that preBötC partially mediates opioid effects on respiratory phase timing, but does not mediate the opioid-induced depression of respiratory rate. By the use of knockout mice and pharmacologic approaches, G-protein–gated inwardly rectifying κ channels were shown to contribute to respiratory depression by μ-opioid receptors and opioids. In healthy humans, GAL021, a calcium-activated potassium (BKCa) channel blocker possessing a stimulatory effect on ventilation at the carotid bodies, was preliminarily shown to reverse alfentanil-induced respiratory depression.
Factors Affecting Opioid-Induced Respiratory Depression
Many factors affect the magnitude and duration of opioid-induced respiratory depression ( Box 24.2 ).
High dose
Sleep
Old age
Central nervous system depressant
Inhaled anesthetics, alcohol, barbiturates, benzodiazepines
Renal insufficiency
Hyperventilation, hypocapnia
Respiratory acidosis
Decreased clearance
Reduction of hepatic blood flow
Secondary peaks in plasma opioid levels
Reuptake of opioids from muscle, lung, fat, and intestine
Pain
Older patients are more sensitive to the anesthetic and respiratory depressant effects of opioids and experience higher plasma concentrations of opioids administered on a weight basis. In addition, morphine can produce greater respiratory depression on a weight basis in neonates than adults, because morphine easily penetrates the brain of neonates and infants with incomplete blood-brain barriers.
The respiratory depressant effects of opioids are increased or prolonged (or both) when these drugs are administered with other CNS depressants, including potent inhaled anesthetics, alcohol, barbiturates, benzodiazepines, most intravenous sedatives, and hypnotics. However, droperidol, scopolamine, and clonidine do not enhance the respiratory depressant effects of fentanyl or other opioids. Curiously, Setnik and associates observed a decrease in end-tidal CO 2 when 0.7 g/kg ethanol was administered in addition to 80 mg oral morphine, suggesting a stimulatory effect of ethanol on opioid-induced respiratory depression. However, it was reported that ethanol (1 g/L of breath ethanol concentration) caused a significant increase in the apneic events and increased end-tidal CO 2 induced by 20 mg oxycodone per os in opioid-naïve healthy volunteers.
Although opioid action is usually dissipated by redistribution and hepatic metabolism, rather than by urinary excretion, the adequacy of renal function may influence the duration of opioid activity. In renal insufficiency, the respiratory depressant properties of morphine and hydromorphone, as well as of the morphine metabolite M6G, become evident as these accumulate.
Hypocapnic hyperventilation enhances and prolongs postoperative respiratory depression after fentanyl (10 and 25 μg/kg). Intraoperative hypercarbia produces the opposite effects. Possible explanations for these findings include increased brain opioid penetration (increased un-ionized fentanyl with hypocarbia) and removal (decreased CBF with hypocarbia). In patients who hyperventilate because of anxiety or pain, even small doses of intravenous opioids can result in transient apnea because of acute shifts in apneic thresholds. Respiratory depression by remifentanil (50 μg infused over 60 seconds) was more pronounced in hyperoxia (inhaling 50% O 2 ) than normoxia as determined by minute ventilation, end-tidal PCO 2 , and respiratory rate. During hyperoxia, respiratory depression may be masked when measuring SpO 2 as pulse oximetry remains in normal values during the first minutes of respiratory depression.
All opioid agonists with a longer plasma half-life than naloxone have a hypothetical potential to show renarcotization with time, especially when a bolus dose of naloxone is used to treat opioid-induced respiratory depression.
Overall, opioids exert their primary respiratory depressant effects through μ-receptor activation in the brainstem respiratory center, although other pathways have been defined. Dose-dependent opioid respiratory depression increases the apneic threshold, and reduces the respiratory stimulatory drive of CO 2 and hypoxia. Research efforts to block or reverse opioid-induced respiratory depression are ongoing and include a focus on AMPA, 5-HT 1A , and calcium-activated potassium channels. The μ-receptor antagonist, naloxone, continues to be the most commonly used clinical intervention to reverse opioid-induced respiratory depression, however its effectiveness may be limited if the administered opioid has a greater binding affinity and/or half-life than naloxone.
Cardiovascular Effects of Opioids
Numerous reports have demonstrated that large doses of opioid, administered as the sole or primary anesthetic, result in hemodynamic stability throughout the operative period. This remarkable physiologic state is a result of a number of complementary mechanisms.
Neurologic Mechanisms
Key areas of the brainstem that integrate cardiovascular responses and maintain cardiovascular homeostasis are the nucleus solitarius, the dorsal vagal nucleus, the nucleus ambiguous, and the parabrachial nucleus. The nucleus solitarius and parabrachial nucleus play an important role in the hemodynamic control of vasopressin secretion. Enkephalin-containing neurons and opioid receptors are distributed in these regions. The direct administration of μ-agonists into the CNS of rats most commonly, but not always, produces hypotension and bradycardia. The ventrolateral PAG region, a key central site mediating opioid analgesia, also affects hemodynamic control. In addition, opioids can modulate the stress response through receptor-mediated actions on the hypothalamic-pituitary-adrenal axis. Most opioids reduce sympathetic and enhance vagal and parasympathetic tone. Patients who are volume depleted, or individuals depending on high sympathetic tone or exogenous catecholamines to maintain cardiovascular function, are predisposed to hypotension after opioid administration.
The predominant and usual effect of opioids on heart rate is bradycardia resulting from stimulation of the central vagal nucleus. Blockade of sympathetic actions may also play a role in opioid-induced bradycardia. Meperidine, in contrast to other opioids, rarely results in bradycardia, but it can cause tachycardia. Tachycardia after meperidine may be related to its structural similarity to atropine, to normeperidine, its principal metabolite, or to early manifestations of its toxic CNS effects.
Cardiac Mechanisms
The direct cardiac actions of opioids, in particular the effects on myocardial contractile mechanisms, are significantly less pronounced than are those of many other intravenous and inhaled anesthetics. However, opioid receptors exist in cardiac myocytes of several species.
Contractility
Morphine decreases Ca 2+ transients but not cardiac contraction and enhances myofilament Ca 2+ sensitivity through the action on the δ 1 -opioid receptor expressed in the heart. In rabbit ventricular myocytes, morphine prolonged action potential duration by increasing L-type Ca 2+ current, an effect mediated by δ- and κ-opioid receptors, and hyperpolarized cardiac resting membrane potential by increasing the inwardly rectifying K + current, which is not mediated by opioid receptors. Conversely, investigators demonstrated that morphine decreased the isometric force of contraction in atrial muscles from nonfailing and failing human hearts through a naloxone-insensitive mechanism. Fentanyl produces little or no change in myocardial contractility. Usually, most hemodynamic variables remain unchanged after large doses of fentanyl. Alfentanil, at concentrations achieved in clinical practice, increases contraction in ventricular cells by a mechanism involving an increase in the sensitivity of the contractile apparatus to Ca 2+ . The negative inotropic effect of tumor necrosis factor-α (TNF-α) and interleukin-1β (IL-1β) on ventricular myocytes caused by disruption of sarcoplasmic reticulum Ca 2+ handling and Ca 2+ transient was reported to be ameliorated by alfentanil, but this response may not be mediated by opioid receptors. A study using transthoracic echocardiography demonstrated that continuous target-controlled infusion of remifentanil (target effect-site concentration 2 ng/mL, infusion rate 0.08-0.09 μg/kg/min) did not affect systolic and diastolic left ventricular function in young healthy subjects during spontaneous breathing.
Cardiac Rhythm Conduction
Opioid-induced bradycardia is primarily mediated by the CNS. However, there have been reports on direct effects of opioids on cardiac pacemaker cells. Fentanyl may depress cardiac conduction by a mechanism mediated by direct membrane actions, as opposed to opioid receptor interactions. During induction of anesthesia in patients undergoing coronary artery bypass graft surgery, the QT interval increased significantly after the injection of fentanyl. However, pretreatment with fentanyl 2 μg/kg or remifentanil 1 μg/kg significantly attenuated QTc prolongation associated with laryngoscopy and tracheal intubation during propofol or sevoflurane induction. Both sufentanil and alfentanil have been demonstrated to be devoid of electrophysiologic effects on the normal or accessory pathways in patients with Wolff-Parkinson-White syndrome. Clinically, cardiac conduction disturbances attributable to opioids are rare, but they may be more likely to occur in the presence of Ca 2+ channel or β-adrenergic blockers.
The overall effect of opioid anesthesia is antiarrhythmic. Naloxone, morphine, and levorphanol protected against arrhythmia induced by coronary artery occlusion in rats. A direct effect on ionic currents in cardiac muscle was suggested as the mechanism of antiarrhythmic activity of opioids. It was also reported that opioid antagonists are more antiarrhythmogenic than agonists in rats. Some of the electrophysiologic actions of opioids resemble those of class III antiarrhythmogenic drugs.
Myocardial Ischemia
Determining the effects and consequences of opioid action on myocardial ischemia is complex because results can depend on such factors as the species studied and experimental design. In an experimental model of myocardial ischemia in rabbits, fentanyl had antiarrhythmic and antiischemic action with central and peripheral opioid receptor involvement. Opioids can mimic ischemic preconditioning. Opioid receptor stimulation results in a reduction in infarct size similar to that produced by ischemic preconditioning. Although the preconditioning effect of opioids is mediated mainly by the cardiac κ- and δ-opioid receptors, part of the protective effect of remifentanil may be produced by μ-agonist activity outside the heart. Preconditioning with small doses of intrathecal morphine can provide comparable cardioprotection to myocardial ischemic preconditioning and preconditioning with intravenous morphine, and the effect seems to involve μ-, δ-, and κ-opioid receptors. Late preconditioning, in which cardioprotective effects can be observed 24 hours after drug administration, was also produced by morphine-induced activation of the opioid receptor in rat hearts.
It was found that remote preconditioning by brief ischemia of other distant organs, such as intestine, kidney, and limb, similarly provides cardioprotection that is as effective as classic ischemic preconditioning. The myocardial κ-opioid receptors were demonstrated to mediate cardioprotection by remote preconditioning. Brief cycles of ischemia and reperfusion during the early phase of reperfusion protect the heart from infarction. This phenomenon, termed postconditioning, was shown to be induced by activation of the δ-opioid receptor in the heart. Volatile anesthetics may also be capable of producing protection against ischemic injury when these drugs are administered solely on reperfusion. This anesthetic-induced postconditioning can be enhanced by morphine through the activation of phosphatidyl-3-kinase and opioid receptors. It has been shown that brief exposure to exercise (1-3 days of moderate-intensity exercise) preconditions the heart against tissue injury and death resulting from myocardial ischemia–reperfusion. It was demonstrated that endogenous opioids produced in the heart mediate exercise-induced cardioprotection against ischemia–reperfusion injury through its action on the δ-opioid receptor in rats. Stimulation of δ 1 -opioid receptor also generates oxygen radicals through mitochondrial ATP-sensitive K + channels, with resulting attenuation of oxidant stress and cell death in cardiomyocytes. Involvement of adenosine A1 receptor and protein kinase C in the cardioprotective effect of opioids was also suggested. Whether the experimental results showing protective effects of opioid against myocardial ischemia will translate into reductions in morbidity and mortality in patients with coronary artery disease has yet to be established by clinical trials. Clinically, high doses of opioids can maintain myocardial perfusion and the O 2 supply-demand ratio as well or better than can inhalation-based techniques.
Coronary Circulation
Opioids appear to have no significant effect on coronary vasomotion or myocardial metabolism, do not produce steal phenomena, nor diminish the ability of large coronary arterioles to respond to vasoactive agents. Coronary conductance is regulated by arterial baroreflex control, and vasodilator response is induced by a rise in aortic pressure. This baroreflex control is enhanced by low plasma concentration of fentanyl (1-2 ng/mL), but appears to be depressed at increased concentrations of fentanyl.
Circulatory Reflexes
In an experiment examining baroreceptor reflex responses induced by perfusion of the carotid sinus at predetermined levels, baroreceptor reflexes were well preserved by moderate doses of fentanyl while high doses of fentanyl depressed baroreceptor reflexes. The oculocardiac reflex, which is caused by traction of extraocular muscles during strabismus surgery, was significantly augmented by fentanyl, sufentanil, and remifentanil. Among pediatric patients undergoing strabismus surgery, anesthetized with propofol (12 mg/kg/h) and alfentanil (0.04 mg/kg/h), virtually all patients developed oculocardiac reflex and atrioventricular rhythm disorders were frequent.
Histamine Release
It is well known that morphine causes histamine release and sympathoadrenal activation. Increases in plasma histamine after morphine cause dilation of terminal arterioles and direct positive cardiac chronotropic and inotropic actions. In patients pretreated with both H 1 – and H 2 -antagonists, the cardiovascular responses are significantly attenuated despite comparable increases in plasma histamine concentrations. Codeine and meperidine are examples of other opioids that can induce mast cell activation with the release of histamine, probably by a mechanism other than the μ-opioid receptors. Unlike morphine or meperidine, the opioids fentanyl, alfentanil, sufentanil, and remifentanil do not produce increases in plasma histamine, subsequently hypotension is less frequent with their administration.
Vascular Mechanisms
The pharmacologically defined opioid receptor subtype μ 3 is expressed in human endothelial cells and coupled to vasodilation via nitric oxide (NO) production. Although μ 3 is opiate alkaloid sensitive it is insensitive to opioid peptides including peptides previously shown to have affinities for the μ-opioid receptors. Morphine-induced vasodilation may be partially caused by activation of the μ 3 receptor. Other pharmacologic studies evaluating alfentanil, fentanyl, and sufentanil in the dog also demonstrate direct peripheral vessel smooth muscle relaxation although exact mechanism(s) remains under study. For example, measurement of forearm blood flow after infusion of sufentanil into the brachial artery indicated that sufentanil has a direct vasodilatory effect on human vascular tissue that is likely independent of a neurogenic or systemic mechanism. A supraclinical dose of alfentanil attenuates the phenylephrine-induced contraction via an inhibitory effect on calcium influx by blocking the L-type calcium channels in the rat aortic vascular smooth muscle. Remifentanil can cause transient instability in hemodynamic variables. However, this change may not be solely the result of autonomic or central nervous system inhibition or of centrally mediated vagal stimulation. A pharmacologic study using rat thoracic aortic rings indicated that remifentanil vasodilates by an endothelium-dependent mechanism, involving prostacyclin and NO released from the endothelium, and an endothelium-independent vasodilation probably mediated by the suppression of voltage-sensitive Ca 2+ channels. In patients with a total artificial heart in which cardiac output is preload-independent, remifentanil induces a dose-dependent and significant systemic vasodilation without significant effects on capacitance vessels.
Opioids may affect the pulmonary vasculature as well as systemic circulation. It was shown that phenylephrine-induced contraction of canine pulmonary artery is primarily mediated by α 1B -adrenergic receptor activation, and is attenuated by fentanyl by binding to and directly inhibiting the α 1B -adrenergic receptor. Pharmacologic studies in cats demonstrated that sufentanil and remifentanil have potent vasodepressor activity in the pulmonary vascular bed and these responses may be mediated by histamine and opioid receptor-sensitive pathway.
Opioids in Shock
Opioids are often administered for patients requiring surgical intervention for the control of hemorrhage. An animal study demonstrated that pretreatment with morphine before inducing shock state decreases leukocyte adhesion and vascular permeability in the microcirculation of the mesenteric venule, suggesting the survival benefit for use of morphine during acute resuscitation.
It has been shown that endogenous opioids contribute to the pathophysiology of hypovolemic shock through central and peripheral sympathetic inhibition and contributes to hypotension during severe hemorrhage. Liu et al. reported that the selective δ-opioid receptor antagonist (ICI 174,864) may be beneficial for the early management of traumatic hemorrhagic shock in rats, suggesting a pathophysiological role of the δ-opioid receptor in hemorrhagic shock.
Endocrinologic Effects of Opioids
Opioids can induce a variety of endocrinological responses ( Table 24.5 ). In humans, opioids generally increase growth hormone, thyroid stimulating hormone, and prolactin, and decrease luteinizing hormone, testosterone, estradiol, and oxytocin. The effects of opioids on arginine vasopressin and ACTH are conflicting. The primary endocrine disorder that results from opioid misuse is hypogonadism, particularly in males.
Acute | Chronic | |||
---|---|---|---|---|
Hormone | Animals | Humans | Animals | Humans |
GH | ↑ | ↑ | = | ? |
PRL | ↑ | ↑ | ↑ | ↑/= |
TSH | ↓ | ↑ | ? | ?/= |
ACTH | ↑ | ↓ | ↓/↑ | ↓/= |
LH | ↓ | ↓↓ | ↓ | ↓↓ |
FSH | = | = | = | = |
Estradiol | ↓ | ↓↓ | = | ↓= |
Testosterone | ↓ | ↓↓ | ↓ | ↓↓ |
AVP | ↑/↓ | ↑/↓ | ↑/↓ | ↑/↓ |
OT | ↓ | ↓ | ↓/= | ↓/= |
Hormonal and metabolic responses to surgery are often extreme and are thought to contribute to operative mortality. Opioids are capable of reducing the stress response by modulating nociception at several levels of the neuraxis, as well as by influencing centrally mediated neuroendocrine responses. The main components of the neuroendocrine stress response are the corticotropin-releasing hormone brain centers (e.g., paraventricular hypothalamic nucleus) and the locus ceruleus-norepinephrine/autonomic nervous system. Increased levels of stress hormones are considered undesirable because they promote hemodynamic instability and intraoperative and postoperative metabolic catabolism. Opioids are potent inhibitors of the pituitary-adrenal axis. Endogenous opioid peptides may serve as stress hormones themselves and not just as modulators of other hormones’ secretion. This is suggested by the finding that β-endorphin and ACTH are derived from the same precursor preproopiomelanocortin and cosecreted during stress.
Morphine modifies hormonal responses to surgical trauma in a dose-related fashion through blockade of ACTH release, suppression of surgically induced increases in plasma cortisol, and attenuation of the pituitary-adrenal response to surgical stress. Morphine can increase some stress-responding hormones due to increases in plasma histamine release, adrenal medullary release mechanisms, and catecholamine release from sympathetic nerve endings.
Fentanyl and its congeners are more effective than morphine in modifying hormonal responses to surgery. The efficacy of fentanyl in controlling the hormonal manifestations of the stress response can be dose-dependent. Fentanyl doses greater than or equal to 50 μg/kg can help to reduce the hyperglycemic response to cardiac surgery in pediatric patients to less than 200 mg/dL throughout operation. In contrast, it has been demonstrated that neither fentanyl nor sufentanil alone can completely block sympathetic and hormonal stress responses and that perhaps no dose-response relationship exists for opioid-associated stress response control. A randomized controlled trial showed that remifentanil (0.85 μg/kg/min), when compared with fentanyl (total doses of 15 and 28 μg/kg), blunts the hypertensive responses and cortisol excretion associated with cardiac surgery but is associated with more hypotension.
Stress Reduction and Outcome
Anesthetic techniques or agents that minimize the stress response may reduce morbidity and mortality in a variety of circumstances. Anand and Hickey evaluated the impact of sufentanil versus morphine-halothane anesthesia on hormonal and metabolic responses and morbidity and mortality in neonates undergoing cardiac surgery. Most strikingly, a difference in postoperative mortality was observed (0 of 30 given sufentanil versus 4 of 15 given halothane plus morphine). Mangano et al. also reported that, after myocardial revascularization, patients receiving intense postoperative analgesia with sufentanil (1 μg/kg/h) experience a decrease in the incidence and severity of electrocardiographically documented ischemia compared with patients receiving intermittent IV morphine (2.2 ± 2.1 mg/h) for postoperative analgesia. It was also shown that large-dose opioids (remifentanil 0.85 μg/kg/min or fentanyl 28 μg/kg) were associated with a decreased rate of myocardial infarction after cardiac surgery.
Many different hormonal changes induced by surgery have been described. However, the concomitant neural, cellular, immune, and biochemical changes have been less well defined, and little is understood or proven with regard to how modifying hormonal responses alters outcome. Additional studies are necessary for complete elucidation of the relationship between control of the surgery-induced hormonal responses and outcome.
In summary, the mechanisms underlying the ability of opioids to provide perioperative cardiovascular stability include: a reduced sympathetic tone and enhanced parasympathetic activity often producing bradycardia; minimal changes in cardiac contractility; function generally as an antiarrhythmic; potentially function as cardioprotective agents to reduce the effect of ischemia by mimicking an endogenous opioid-peptide/preconditioning pathway; have no significant effect on the coronary circulation; produce modest vascular smooth muscle relaxation with the exception of a morphine-induced histaminergic mechanism; reduce the surgical stress response through the nervous system and adrenal-pituitary axis—depending on the opioid class.
Opioid Tolerance
The precise mechanism(s) that drive opioid dependence and tolerance are not known, however they appear to encompass a range of factors including: genetic, molecular, cellular, physiologic, and functional. In the locus ceruleus, the major noradrenergic nucleus in the brain, long-term opioid exposure results in inhibition of adenylyl cyclase, reduced activity of protein kinase A, and upregulation of the cyclic AMP pathway. Changes in μ-receptor density that occur prior to or during the development of tolerance do not appear to be essential for development of opioid tolerance. Possible mechanisms involve protein kinase signal transduction cascades that link extracellular signals to cellular changes by regulating target gene expression. Central glucocorticoid receptors (GRs) have been implicated in the cellular mechanism of neuronal plasticity that has many cellular steps in common with the mechanism of opioid tolerance. It was shown that the development of tolerance to the antinociceptive effect of morphine was substantially attenuated when the GR antagonist was coadministered with morphine but the GR agonist dexamethasone facilitated the development of morphine tolerance, suggesting an important role of spinal GRs in the cellular mechanisms of morphine tolerance in rats. Cholecystokinin and NMDA-NO system were also shown to be involved in development of acute tolerance to opioids, which is also affected by spinal serotonin activity. Neuroinflammation driven by chemokines may represent one of the major mechanisms underlying pathologic pain. A chemokine, CXCL1, was upregulated in both opioid-tolerant patients and rodents, and the onset and extent of opioid tolerance was affected by antagonizing intrathecal CXCL1/CXCR2 signaling. A chemokine, CXCL12, was significantly upregulated in the cerebrospinal fluid of opioid-tolerant patients, and CXCL12 neutralizing antibody and antagonist for CXCR4, a receptor which interacts with CXCL12, attenuated morphine tolerance in rats.
Morphine tolerance occurs more rapidly in younger rats than older rats and is unlikely to be the result of differences in drug metabolism or clearance, suggesting that aging may impact molecular processes involved in development of tolerance. It has been suggested that activation of glial cells, including astrocytes and microglia, at the level of the spinal cord plays an important role in the development of opioid tolerance. However, the mechanism for opioid-induced activation of glial cells is not completely understood.
Although the notion that opioid tolerance and dependence occur only after chronic administration has been widespread, it has now become recognized that tolerance can also develop rapidly after acute opioid exposure in animals and humans. Intraoperative remifentanil infusion (0.3 μg/kg/min) in patients undergoing major abdominal surgery under desflurane anesthesia increased postoperative pain and morphine requirement compared with low-dose remifentanil (0.1 μg/kg/min), suggesting the development of acute remifentanil tolerance. In contrast, there is a report that target-controlled infusion of alfentanil and remifentanil for postoperative analgesia does not lead to opioid tolerance. In human volunteers, continuous infusion of remifentanil (0.08 μg/kg/min) for 3 hours did not decrease the pain threshold. On the other hand, intraoperative use of 0.3 μg / kg/min remifentanil for approximately 3 hours did not induce acute tolerance, but the administration of 0.6 and 0.9 μg / kg/min remifentanil to young children resulted in acute tolerance for 24 hours after surgery revealed by increased use of postoperative fentanyl in an apparently dose-related manner. Differences in these outcomes may reflect divergent methodologies and limited sample size and are an area of ongoing investigation.
Differential opioid tolerance, an important phenomenon in clinical opioid pharmacology, proposes that different targets of opioid drugs do not develop tolerance at the same rate and to the same degree. In a study using rhesus monkeys, acute morphine administration predictably induced dose-dependent analgesia, and chronic morphine administration induced dose-dependent tolerance to the analgesic effect, while no tolerance development to the respiratory depressive effect could be demonstrated in the chronically opioid-treated animals ( Fig. 24.14 ). In humans, patients receiving chronic opioids for pain control, especially at high doses, most likely have developed opioid tolerance. However, there is little data to help predict the magnitude or clinical impact of an individual’s loss of opioid-induced analgesia or potentially their resistance/vulnerability to the respiratory depressive effects of opioids. As discussed later, individuals chronically consuming opioids are dose dependently at increased risk for overdose and death.

Management of Opioid-Dependent Patients
A variety of problems should be taken into consideration for anesthetic management of opioid-dependent patients or patients suffering from opioid use disorder (OUD). Complications in opioid-addicted patients include cardiopulmonary problems, renal problems, and anemia. Long-term morphine administration causes adrenal hypertrophy and impairs corticosteroid secretion. Viral and nonviral hepatitis, acquired immunodeficiency syndrome, osteomyelitis, muscle weakness, and neurologic complications may be found in patients suffering from OUD or poly substance use disorder. As it is common to underestimate and undertreat pain in opioid-dependent patients, it is important to identify the goals in their acute pain management ( Box 24.3 ). Anesthetic management for the opioid-dependent or patient with OUD should include adequate premedication with opioids, administration of supplemental intraoperative and postoperative opioids, and providing nonopioid analgesics and neural blockade. There is no ideal anesthetic agent or technique to employ in the patient with OUD or in the patient with an acute opioid overdose—with the possible exception of judicious use of an opioid antagonist as indicated. As mentioned previously, combined techniques utilizing regional anesthetic approaches, as well as concurrent use of low-dose ketamine and α2-agonists have been successful. Support of the circulatory system with fluids and monitoring of arterial blood gases and pulmonary function are essential.
- 1.
Identification of the population of at-risk patients receiving long-term opioid therapy for various chronic pain situations (musculoskeletal disease, neuropathic conditions, sickle cell disease, HIV-related disease, palliative care), persons recovering in opioid maintenance programs
- 2.
Prevention of withdrawal symptoms and complications
- 3.
Symptomatic treatment of psychological affective disorders such as anxiety
- 4.
Effective analgesic treatment in the acute phase
- 5.
Rehabilitation to an acceptable and suitable maintenance opioid therapy
HIV , Human immunodeficiency virus.
Owing to the high risk of relapse, overdose, and death in individuals with OUD, medication-assisted treatment (MAT) in which opioids with different pharmacokinetic and pharmacodynamic properties such as methadone or buprenorphine are commonly prescribed. Because of the injection-deterring potential of naloxone hydrochloride and a better safety profile compared with methadone, daily administration of combined buprenorphine and naloxone is becoming a first-line choice for MAT in a number of countries. Other treatment approaches have emerged including rapid opioid detoxification with high-dose naloxone or naltrexone. For this treatment, general anesthesia is induced before the start of opioid antagonization and maintained for several hours to prevent perception of withdrawal symptoms by the patient. Blockade of μ-opioid receptors by naloxone (total dose of 12.4 mg) in opioid-addicted patients induces sympathetic neural activation, including increase in plasma catecholamine concentration and cardiovascular stimulation, which can be managed in part by α 2 -agonists. Long-term opioid abstinence is not guaranteed, even with an ongoing program that includes MAT, given the neurobiology, societal factors involved in addiction, and potential for repeat exposure—often medically in the perioperative period.
Renal and Urodynamic Effects of Opioids
Opioids can have significant effects on renal function. μ-receptor activation causes antidiuresis and decreases electrolyte excretion. κ-receptor stimulation predominantly produces diuresis with little change in electrolyte excretion. Indirect actions may involve inhibiting or altering the secretion of ADH and atrial natriuretic peptide. The absence of increases in plasma ADH, renin, and aldosterone indicate that fentanyl, sufentanil, alfentanil, and probably remifentanil most likely preserve or minimally alter renal function in humans. If renal function does change during opioid anesthesia and surgery, it is probably due to secondary changes in systemic and renal hemodynamics.
The mechanism by which opioids cause urinary retention is incompletely understood. Opioid effects on the lower urinary tract include disturbances of micturition characterized by urinary retention, especially after intrathecal opioid administration. Intrathecal administration of morphine and sufentanil caused dose-dependent suppression of detrusor contractility and decreased sensation of urge. Mean times to recovery of normal lower urinary tract function were 5 and 8 hours after 10 or 30 μg sufentanil and 14 and 20 hours after 0.1 or 0.3 mg morphine, respectively. Not all opioid agonists behave similarly, and morphine appears to be particularly potent with regard to producing urodynamic problems. Malinovsky et al. compared urodynamic effects of intravenously administered morphine (10 mg), buprenorphine (0.3 mg), fentanyl (0.35 mg), and nalbuphine (20 mg). It was shown that all of the opioids altered bladder sensations, but that detrusor contraction decreased only after administration of fentanyl and buprenorphine. Urinary retention induced by intravenous infusion of remifentanil, 0.15 μg/kg/min could be reversed by a single intravenous dose of methylnaltrexone 0.3 mg/kg or naloxone 0.01 mg/kg. Reversal of urinary retention by methylnaltrexone indicates that peripheral mechanisms may play a role in opioid-induced bladder dysfunction.
A retrospective study for adult patients with chronic kidney disease undergoing orthopedic surgery estimated glomerular filtration rate during the postoperative period was significantly higher in the group in which remifentanil was used for anesthesia management than the group in which remifentanil was not used. This finding may suggest that anesthesia management using remifentanil may have a renal protective effect in adult patients with chronic kidney disease.
Effects of Opioids on Digestive Organs
Effects on Gastrointestinal Tract
The adverse gastrointestinal effects of exogenous opioid treatment include nausea, vomiting, altered fluid dynamics, inhibited gastric emptying, inhibited intestinal coordinated propulsive activity, and increased transit time, all of which may contribute to postoperative ileus ( Table 24.6 ). Opioid-dependent mechanisms driving these effects are complex and those involving GI motility are believed to involve opioid receptors expressed throughout the myenteric plexus. Several opioid receptor types can be demonstrated on myenteric neurons, and both κ- and μ-receptor agonists regulate cholinergic transmission in the myenteric plexus. κ-Agonists appear to modulate acetylcholine release more potently than μ-agonists by inhibition of N-type voltage-sensitive Ca 2+ channels via a pertussis toxin-sensitive G protein in guinea pig ileum.
Pharmacologic Action | Clinical Effect |
---|---|
Decreased gastric motility and emptying | Decreased appetite; increased gastroesophageal reflux |
Decreased pyloric tone | Nausea and vomiting |
Decreased enzymatic secretion | Delayed digestion; hard, dry stools |
Inhibition of small and large bowel propulsion | Delayed absorption of medication; straining; incomplete evacuation; bloating; abdominal distension; constipation |
Increased fluid and electrolyte absorption | Hard, dry stools |
Increased nonpropulsive segmental contractions | Spasms; abdominal cramps; pain |
Increased anal sphincter tone | Incomplete evacuation |
The effect of morphine on esophageal motility has been little explored. Morphine (80 μg/kg) increased the velocity but did not alter the amplitude or duration of primary peristalsis of the esophagus, and it decreased the duration and magnitude of swallow-induced lower esophageal sphincter relaxation. Gastric emptying is delayed by opioids, via supraspinal (vagus nerve-mediated) and spinal, as well as peripheral, mechanisms. Intrathecal morphine (0.4 mg) significantly decreased the gastroduodenal propagation velocity and acetaminophen absorption, and intramuscular morphine (4 mg) gave additional effects. Tramadol (1.25 mg/kg IV) has a measurable but smaller inhibitory effect on gastric emptying compared with codeine (1 mg/kg IV) or morphine (0.125 mg/kg IV). Opioids administered epidurally as well as intrathecally reduce gastrointestinal motility. It was reported that translocation of enteric microorganisms from the intestinal tract to extraintestinal sites is promoted by reduction of gut propulsion after morphine treatment in rats. Propofol (0.3 mg/kg bolus and 1.0 mg/kg/h) abolished the decrease of gastric tone induced by morphine (0.1 mg/kg intravenously), but did not abolish morphine-induced delay of gastric emptying.
Naloxone reverses opioid-induced delays in gastric emptying. Methylnaltrexone, a quaternary naloxone derivative that does not cross the blood-brain barrier, can attenuate morphine-induced delays in gastric emptying, suggesting that a peripheral mechanism is involved in the opioid effect on gastrointestinal tract. Naloxone (0.7 mg/kg) significantly inhibited gastric emptying of saline and milk in rats. This observation might suggest that opioids can affect the gastrointestinal tract by a mechanism independent from the opioid receptors. Intravenous, but not intramuscular, metoclopramide (10 mg) also can reverse morphine-induced delays in gastric emptying.
Opioid effects on the intestine are complex. Transit time from mouth to ileum may not be significantly altered by morphine, because morphine enhances ileal propulsion before decreasing motility. Opioids increase tone and decrease propulsive activity in most of the intestine. Constipation is the frequent side effect in patients who are administered opioids. Naloxonazine attenuated the fentanyl-induced inhibition of gastrointestinal transit more potently than the inhibition induced by morphine or oxycodone. Naloxone methiodide suppressed the oxycodone-induced inhibition of gastrointestinal transit more potently than the inhibition induced by morphine. Thus, μ-opioid receptor agonists induce the inhibition of gastrointestinal transit and drive constipation through different mechanisms.
Biliary and Hepatic Effects
Opioid agonists increase biliary duct pressure and sphincter of Oddi (choledochoduodenal sphincter) tone in a dose- and drug-dependent manner through opioid receptor-mediated mechanisms. However, the clinical consequences of opioid-induced biliary tract actions are usually minimal. Although traditional teaching dictates that morphine induces “spasm” in the sphincter of Oddi and should not be used in acute pancreatitis, no studies or evidence exist to indicate morphine is contraindicated for use in acute pancreatitis. Increases in biliary pressure caused by opioids are, with the exception of meperidine, reversible with naloxone. Oddi’s sphincter manometry via choledochoscope demonstrated that the regular dose of morphine could increase common bile duct pressure, whereas pethidine had no effect on Oddi’s sphincter motility and tramadol shows inhibited motility of the sphincter of Oddi. Fragen et al. studied the effect of remifentanil (0.1 mg/kg/min) on the flow of dye from the gall bladder into the duodenum, and showed that remifentanil delays the drainage of dye from the gall bladder into the duodenum, but the delay is shorter than that reported after morphine or meperidine.
Opioids produce minimal effects on liver function during anesthesia and surgery but can affect ischemia-reperfusion injury. Remifentanil pretreatment can attenuate liver injury induced by ischemia-reperfusion, which was shown to be mediated by inducible NO synthase expression and exhausting reactive oxygen species but does not involve opioid receptors. Morphine administered either intravenously or intrathecally 10 minutes before 1 hour of ischemia protects against ischemia-reperfusion injury after 6 hours of reperfusion in both normal and cirrhotic rat liver by a mechanism involving the opioid receptor. Remifentanil significantly attenuated increases in serum aminotransferase levels and the liver histological changes induced by ischemia-reperfusion injury of the liver in rats, by a mechanism possibly involving hepatic interleukin-18. These reports might suggest the beneficial effects of opioids in anesthetic management of liver surgery.
Nausea and Vomiting
Postoperative nausea and vomiting are serious problems which often embarrass anesthesiologists. Etiology, treatment, and prevention of PONV have been extensively investigated ( Fig. 24.15 ). Intraoperative use of opioids is a well-known risk factor for PONV . Opioids stimulate the chemoreceptor trigger zone in the area postrema of the medulla possibly through δ-receptors, leading to nausea and vomiting. Alfentanil, compared with approximately equipotent doses of fentanyl and sufentanil, was found in one study to be associated with a lower incidence of PONV .

The use of propofol in balanced or total IV anesthesia (TIVA) significantly reduces the incidence of opioid-induced nausea and vomiting. When opioids are employed, antiemetic prophylaxis should be considered, which includes drugs with anticholinergic activity, butyrophenones, dopamine antagonists, serotonin antagonists and acupressure. Ondansetron, a serotonin type 3 (5-HT 3 ) receptor antagonist, was proved to be effective for postoperative opioid-induced nausea and vomiting. A meta-analysis concluded that prophylactic use of 5-HT 3 receptor antagonists significantly reduced the incidence of PONV and the need of rescue antiemetic therapy in parturients who received intrathecal morphine for cesarean delivery. Nausea and vomiting after epidural morphine (3 mg) for postcesarean section analgesia could be prevented by dexamethasone (8 mg IV) as efficiently as droperidol (1.25 mg IV). Cannabinoid receptor agonists have been demonstrated to be effective antiemetics in some clinical settings. Animal experiments have shown that the cannabinoid agonist suppresses opioid-induced retching and vomiting by activation of the cannabinoid CB1 receptor. A continuous low-dose naloxone infusion (0.25 μg/kg/h) ameliorates some of the opioid-induced side effects, including nausea, vomiting, and pruritus, in many but not all patients without adversely affecting analgesia. Transdermal scopolamine was shown to be effective for prophylactic use in parturients receiving intrathecal morphine while undergoing cesarean delivery but was associated with higher incidence of side effects such as dry mouth and blurry vision.
Taken together, the prevention and/or treatment of opioid-induced nausea and vomiting continues to be a clinical challenge. Strategies that include the administration of 5-HT 3 receptor antagonists and/or the steroid dexamethasone show efficacy in well controlled trials. However, such approaches require exposure to additional medications that, in themselves, may carry additional risks of side effects.
Other Opioid Effects
Obstetrics
The parenteral administration of opioids for oocyte retrieval and prior to delivery remains a commonly used method of analgesia.
Alfentanil and pethidine have been safely used as analgesics during the harvesting of human oocytes for subsequent in vitro fertilization. Teratogenic actions of opioids, including fentanyl, sufentanil, and alfentanil, at least in animal models, appear to be minimal. Nociception due to uterine cervical distension could be suppressed by μ- and κ-agonists in rats, but the analgesic effect of μ-agonist but not κ-agonist was reduced by estrogen. Aortocaval compression and associated hypotension may be exacerbated by parenteral opioids, especially following morphine or meperidine. Fetal manifestations of maternal opioid administration include decreases in heart rate variability. Adverse neonatal effects can occur after either morphine or meperidine administration to mothers. Fetal acidosis increases opioid transfer from the mother. Attempts to minimize neonatal effects of opioids include restricting opioid administration to the first stage of labor. The short-acting opioid alfentanil administered before cesarean delivery attenuated the maternal stress response, but led to slightly reduced Apgar score. In a randomized, double-blind, controlled study, it was shown that a single bolus of 1 μg/kg remifentanil administered to patients undergoing elective cesarean delivery effectively attenuated hemodynamic changes after induction and tracheal intubation, but remifentanil crosses the placenta and may cause mild neonatal depression.
Because the fetus is capable of pain perception after the 26th week of gestation, adequate postoperative fetal pain management is essential after fetal surgery. It was shown that the sheep fetus absorbs sufentanil after intraamniotic instillation, and that significantly greater plasma concentrations were obtained in the fetal lamb as compared with the mother sheep.
Morphine and meperidine have been found in the breast milk of mothers receiving intravenous opioid analgesia. Although both fentanyl and morphine are concentrated in breast milk in milk-to-plasma ratios of 2 to 3:1, newborn exposure is reported to be insignificant. Newborns of mothers with OUD or taking prescription opioids can exhibit opioid withdrawal often referred to as neonatal abstinence syndrome (NAS) and require appropriate treatment and observation.
Anaphylactoid Reactions
True allergic reactions and systemic anaphylactoid reactions to opioids are rare. More commonly, local reactions caused by preservatives or histamine may occur. In 32% of heroin addicts dying suddenly after injection, the concentration of tryptase was elevated (>10 μg/L), but no correlation was found between the IgE levels and tryptase, supporting the hypothesis that mast cell degranulation was not mediated by allergic reaction. This report suggests that heroin fatalities may be in part driven by anaphylactoid reaction.
Ocular Effects
The use of fentanyl, sufentanil, and alfentanil during induction of anesthesia can help to prevent increases in intraocular pressure. Fentanyl, alfentanil, and sufentanil doses as small as 2.5, 10, and 0.1 μg/kg, respectively, may be sufficient as long as appropriate anesthetic depth is achieved prior to tracheal intubation. Remifentanil (1 μg/kg) combined with propofol (2 mg/kg) or thiopental (5 mg/kg) was reported to be effective for prevention of intraocular pressure change after succinylcholine and tracheal intubation.
Immune Effects
Opioids can affect immune function through adaptive immunity, innate immunity, and neuroendocrine system ( Box 24.4 ). The literature investigating the function of classical opioid receptors (μ, δ, and κ) present on immune cells suggest a complex relationship between clinically used opioids and immune function, including through indirect mechanisms. However, because the μ-opioid receptor knockout mice showed no immune modulatory effects after central administration of opioids, the central immune modulatory effect of opioids is mediated by μ-opioid receptors.
Adaptive Immunity
↓ Splenic and thymic weight (rodents)
↓ T cell viability and proliferative response
↓ T-helper cell function
↓ CD4/CD8 population in vivo
↓ IL1β, IL-2, TNF-α, and IFN-γ (mouse splenocytes)
↓ Th1/Th2 ratio of T-helper cell population (PBMCs)
↓ NK cell activity
↓ Primary antibody response (B cells)
↓ B cells mitogenic response to bacterial LPS
↓ Macrophage activity
↓ TGF-β1 and IL-10 (antiinflammatory cytokines)
↑ T cell apoptosis (NF-κβ and AP-1/NFAT pathways)
Inhibition of CD3/28 mAb induced IL-2 transcripts
Innate Immunity
↓ Number of macrophages available to fight infections
↓ Leucocyte migration
↓ Peritoneal macrophages phagocytosis
↓ Respiratory burst activity and chemotaxis
Inhibition of Fc γ receptor mediated phagocytosis
↓ Superoxide production from neutrophils and macrophages
Alteration of IL-8 induced neutrophil chemotaxis
↓ Neutrophil cytokines involved in wound healing
↑ Apoptosis of macrophages impairing host defense barrier
↓ Leucocytes endothelial adhesion (intracellular adhesion molecules expression)
Neuroendocrine System
↑ Growth hormone, prolactin, and thyroid stimulating hormone secretion in humans
May affect the function of the HPA axis (ACTH and CRH) with risk of adrenal insufficiency
↓ Sex hormones [LH and testosterone (hypogonadism)], oxytocin, and estradiol
ACTH , Adrenocorticotropic hormone; AP-1 , activator protein 1; CRH , corticotropin releasing hormone; Fc , fragment crystallizable region; HPA , hypothalamic pituitary adrenal axis; IL , interleukin; IFN-γ , interferon-gamma; LH , luteinizing hormone; LPS , lipopolysaccharides; NF-κβ , nuclear factor kappa beta; NFAT , nuclear factor of activated T-cells; NK , natural killer; PBMC , peripheral blood mononuclear cell; TGF-β , transforming growth factor beta; TNF-α , tumor necrosis factor-alpha.
It was shown that the maximal suppression of natural killer (NK) cell activity, proliferation of splenic T and B cells, and interferon-γ production are observed 0.5 to 1 hour after 15 mg/kg morphine injection in rats. The time course was nearly concordant with the antinociceptive effect of morphine. Postoperative administration of morphine (10 mg IM) did not significantly affect the NK cell activity, while tramadol (100 mg IM) enhanced NK cell activity. It was reported that intravenously administered fentanyl causes a rapid increase in NK cell cytotoxicity, which was coincident with an increase in the percentage of CD16 + and CD8 + cells in peripheral blood. Compared with fentanyl (1000 μg), administration of morphine (40 mg) as part of balanced anesthetic technique suppressed several components of the inflammatory response (IL-6, CD11b, CD18, postoperative hyperthermia) to cardiac surgery and cardiopulmonary bypass.
As a potential mechanism for the immunosuppressive effects of morphine, it was demonstrated that NF-κβ activation induced by an inflammatory stimulus was inhibited by morphine-induced activation of μ 3 -opioid receptors in a NO-dependent manner. Several investigators have independently reported direct effects of morphine on apoptosis in cultured human peripheral blood lymphocytes, which may result in compromising immune functions. However, there is also a report that morphine has no effect on apoptosis-related molecules and does not promote apoptosis of human peripheral blood lymphocytes.
With respect to the effects of opioids on neutrophils, it was reported that remifentanil, but not sufentanil, alfentanil, or fentanyl, could attenuate activation of human neutrophils exposed to lipopolysaccharides, and decreased activation of intracellular signaling pathways, including p38 and ERK1/2, and expression of proinflammatory cytokines, including TNF-α, IL-6, and IL-8, through a mechanism involving the κ-opioid receptor. It was also reported that remifentanil attenuates lipopolysaccharide-induced acute lung injury by inhibition of proinflammatory cytokine production by downregulating the NF-κβ pathway, suggesting a beneficial effect of remifentanil in acute lung injury or acute respiratory distress syndrome in sepsis.
A prospective study for adult patients who underwent elective colorectal surgery demonstrated that the number of patients who developed surgical site infection was higher after remifentanil-based anesthesia (11.6%) compared with fentanyl-based anesthesia (3.4%). A possible reason for this finding may be opioid-induced immunosuppression or opioid withdrawal-induced immunosuppression.
Cancer Progression
Epidemiological studies have suggested that patients who receive general anesthesia with opioids have a greater rate of cancer recurrence than patients who receive local or regional anesthetics, although there is no direct evidence to support altering anesthetic technique in cancer patients. Opioids may directly stimulate proliferation and invasion of tumor cells and inhibit apoptosis of tumor cells, or indirectly affect cancer recurrence by immunosuppression. Overexpression of the μ-opioid receptor in human non-small cell lung cancer was suggested to promote tumor growth and progression. Furthermore, it was reported that women with A118G genotype of the μ-opioid receptor have decreased breast cancer-specific mortality, suggesting that opioid pathways may be involved in tumor growth. Drawing from preclinical studies using animals or cultured cells opioids may promote tumor growth and metastasis through multiple mechanisms, whereas it is also reported that opioids can direct various anticarcinogenic pathways ( Fig. 24.16 ). Preclinical data have also suggested that μ-opioid receptor inhibition can reverse the adverse effect of μ-opioid receptor signaling on cancer progression, when a combination of methylnaltrexone, which blocks peripheral but not central μ-opioid receptor, and chemotherapy is tested.

One of the mechanisms involved in the effects of opioids on cancer prognosis is their effects on angiogenesis. Morphine can stimulate angiogenesis by a variety of mechanisms involving NO, MAPK, VEGF, and Rho/Rho kinase. Blebea et al. reported that activation of opioid receptors inhibited angiogenesis, via endogenous opioid ligands. Although both pro- and anti-angiogenic actions of opioids have been reported, it is generally believed that the pro-angiogenic (or neo-vasculogenic) effects predominate.
The opioid growth factor receptor (OGFR) is localized in both the nucleus and the cytoplasm and functions as a receptor for OGF, also known as methionine-enkephalin. OGFR is distinguished from classic opioid receptors (μ, δ, and κ) as not having any role in analgesia but functions as a negative regulator of cell proliferation. Morphine can also interact with OGFR expressed in lung cancer tissues and cell lines and may suppress lung cancer progression.
Wound Healing
Topical application of opioids has been explored as a strategy for reducing pain associated with cutaneous wounds. In addition to its analgesic functions, the peripheral opioid receptor system affects skin homeostasis by influencing cell differentiation, migration, and adhesion. Activation of peripheral opioid receptors on primary afferent neurons reduces the excitability of these neurons and suppresses the antidromic release of substance P and calcitonin gene-related peptide, which play an essential role in wound repair. It was shown that topical morphine application significantly reduced the number of myofibroblasts and macrophages in the closing wound. These findings might limit the topical application of opioids as an analgesic therapeutic strategy in the treatment of painful cutaneous wounds. In contrast, it was shown that activation of the δ-opioid receptors destabilizes intercellular adhesion and promotes the migratory keratinocyte phenotype, which is required for fast wound closure. If these divergent findings can be resolved in favor of wound healing and enhanced localized analgesia, there is great potential for opioid applications in wound healing. Most clinical studies have not focused on opioid effects on the wound healing process but substantial analgesic effects without delaying wound closure were found. Larger clinical trials are required to examine whether opioids interfere with wound healing by inhibition of proinflammatory cytokine release or by development of hypertrophic scars, as shown in animal studies.
Pharmacokinetics and Pharmacodynamics of Opioids
With the advent of modern drug assay technology and the widespread availability of computers, it is now possible to analyze and model pharmacologic data which separates drug response into pharmacokinetic and pharmacodynamic components. Pharmacokinetic parameters govern the relationship between opioid dose and the opioid concentrations in the blood (or other body fluids). Pharmacodynamic parameters describe the relationship between opioid concentration in blood (or other fluids) and opioid effect.
Computer simulation techniques predict “context-sensitive half–times,” the time necessary to achieve a 50% decrease in drug concentration after termination of a variable-length continuous infusion to a steady-state drug level (see ). Such simulations are intended to provide more clinically relevant meaning to pharmacokinetic parameters. The context-sensitive half-time and computer simulations have helped clinicians to select opioids in a more rational fashion. After 1-hour continuous infusion, the context-sensitive half-time of fentanyl is nearly six times that of alfentanil or sufentanil. Remifentanil’s context-sensitive half-time is independent of infusion duration.
Physicochemical Properties
Opioids are weak bases. When dissolved in solution, they are dissociated into protonated and free-base fractions, with the relative proportions depending on the pH and pKa. The free-base fraction is more lipid-soluble than the protonated fraction. High lipid solubility facilitates opioid transport into the biophase or site of action. Therefore, highly lipid-soluble opioids have a more rapid onset of action. However, because the opioid receptor recognizes an opioid molecule in the protonated form, the intensity of opioid effects is closely related to the ionized concentration of drug in the biophase.
All opioids are to some extent bound to plasma proteins, including albumin and α 1 -acid glycoprotein. It is only the un-ionized, unbound fraction that constitutes the diffusible fraction and provides the concentration gradient that promotes diffusion of opioid from the blood to the tissue of interest. Thus, the speed of onset of opioid effect is affected by both the lipid solubility and the protein binding.
Pharmacokinetic Features of Individual Drugs
Representative pharmacokinetic parameters for the opioids commonly used in anesthesia are displayed in Table 24.7 .
Morphine | Fentanyl | Sufentanil | Alfentanil | Remifentanil | |
---|---|---|---|---|---|
p K a | 8.0 | 8.4 | 8.0 | 6.5 | 7.1 |
% Un-ionized at pH 7.4 | 23 | <10 | 20 | 90 | 67? |
Octanol/H 2 O partition coefficient | 1.4 | 813 | 1778 | 145 | 17.9 |
% Bound to plasma protein | 20-40 | 84 | 93 | 92 | 80? |
Diffusible fraction (%) | 16.8 | 1.5 | 1.6 | 8.0 | 13.3? |
t 1/2 α (min) | 1-2.5 | 1-2 | 1-2 | 1-3 | 0.5-1.5 |
t 1/2 β (min) | 10-20 | 10-30 | 15-20 | 4-17 | 5-8 |
t 1/2γ (h) | 2-4 | 2-4 | 2-3 | 1-2 | 0.7-1.2 |
Vd c (L/kg) | 0.1-0.4 | 0.4-1.0 | 0.2 | 0.1-0.3 | 0.06-0.08 |
Vd ss (L/kg) | 3-5 | 3-5 | 2.5-3.0 | 0.4-1.0 | 0.2-0.3 |
Clearance (mL/min/kg) | 15-30 | 10-20 | 10-15 | 4-9 | 30-40 |
Hepatic extraction ratio | 0.6-0.8 | 0.8-1.0 | 0.7-0.9 | 0.3-0.5 | NA |
Morphine
Morphine pharmacokinetics is notably different from that of the fentanyl congeners. This difference is in large part due to morphine’s comparatively low lipid solubility. There is relatively little transient first-pass uptake of morphine by the lung. The pKa of morphine (8.0) is greater than physiologic pH, and thus after intravenous injection only a small fraction (10%-20%) of morphine is un-ionized. Penetration of morphine into and out of the brain is presumably slower compared with that of other opioids. Approximately 20% to 40% of morphine is bound to plasma proteins, mostly albumin.
Morphine is principally metabolized by conjugation in the liver, but the kidney plays a key role in the extrahepatic metabolism of morphine. M3G is the major metabolite of morphine, but does not bind to opioid receptors and possesses little or no analgesic activity. M3G may actually antagonize morphine, and this effect may contribute to both variability in response and resistance to morphine analgesic therapy. M3G was reported to produce seizures in animals and cause allodynia in children. M6G accounts for nearly 10% of morphine metabolite and is a more potent μ-receptor agonist than morphine with a similar duration of action. It was reported that M6G contributes substantially to morphine’s analgesic effects even in patients with normal renal function. A recent study reported that based on area under the concentration–time curve there was a major contribution of M6G to the overall analgesic effect; the mean contributions being estimated as 96.6%, 85.6%, 85.4%, and 91.3% after oral, subcutaneous, intravenous, and rectal administration of morphine, respectively. In patients with renal insufficiency, 97.6% of the analgesic effect is caused by M6G when morphine is given orally. Especially in patients with renal dysfunction, the accumulation of M6G can lead to an increased incidence of adverse effects, including respiratory depression. Except for renal function, M6G accumulation was shown to be affected by transmembrane transporters inhibited by probenecid. M6G can induce respiratory depression similarly as morphine, but the site of action in the ventilatory control system might be different between M6G and morphine. It was suggested that SNP at the μ-opioid receptor affects the susceptibility to M6G-related opioid toxicity. Because the hepatic extraction ratio of morphine is high, the bioavailability of orally administered morphine is significantly lower (20%-30%) than after intramuscular or subcutaneous injection. It appears that M6G is in fact the primary active compound when morphine is administered orally ( Fig. 24.17 ). In contrast to the reports suggesting the high potency of M6G, there have been reports showing that short-term intravenous administration of M6G does not provide effective analgesia.

Fentanyl
A three-compartment model is typically used to describe plasma fentanyl concentration decay. The lungs exert a significant first-pass effect and transiently take up approximately 75% of an injected dose of fentanyl. Approximately 80% of fentanyl is bound to plasma proteins, and significant amounts (40%) are taken up by red blood cells. Fentanyl is relatively long acting, in large part because of this widespread distribution in body tissues.
Fentanyl is primarily metabolized in the liver by N -dealkylation and hydroxylation. Metabolites begin to appear in the plasma as early as 1.5 minutes after injection. Norfentanyl, the primary metabolite, is detectable in the urine for up to 48 hours after intravenous fentanyl in humans.
Alfentanil
Following IV injection, alfentanil plasma concentrations are described by either two-compartment or three-compartment model. Alfentanil is bound to plasma proteins (mostly glycoproteins) in higher proportions (92%) than fentanyl. At physiologic pH, it is mostly (90%) un-ionized because of its relatively low pKa (6.5). Thus, despite more intense protein binding, the diffusible fraction of alfentanil is higher than fentanyl. This explains, in part, its short latency to peak effect after intravenous injection.
The main metabolic pathways of alfentanil are similar to those of sufentanil and include oxidative N -dealkylation and O -demethylation, aromatic hydroxylation, and ether glucuronide formation. The degradation products of alfentanil have little, if any, opioid activity. Human alfentanil metabolism may be predominantly, if not exclusively, by cytochrome P-450 3A3/4 (CYP3A3/4). This enzyme is known to display at least 8-fold difference in activity in humans. Alfentanil is also metabolized by human liver microsomal CYP3A5, which shows more than 20-fold pharmacogenetic variability in expression level leading to significant differences in human liver alfentanil metabolism. In vitro study demonstrated that propofol in clinically relevant concentrations interferes with oxidative metabolic degradation of alfentanil and sufentanil in the microsomal fraction of pig and human liver.
Sufentanil
The pharmacokinetic property of sufentanil is adequately described by a three-compartment model. After intravenous injection, first-pass pulmonary extraction, retention, and release are similar to those of fentanyl. The pKa of sufentanil at physiologic pH is the same as that of morphine (8.0), and, therefore, only a small amount (20%) exists in the un-ionized form. Sufentanil is twice as lipid-soluble as fentanyl and is highly bound (93%) to plasma proteins including α 1 -acid glycoprotein.
The major metabolic pathways of sufentanil include N -dealkylation, oxidative O -demethylation, and aromatic hydroxylation. Major metabolites include N -phenylpropanamide.
Remifentanil
Although chemically related to the fentanyl congeners, remifentanil is structurally unique because of its ester linkages. Remifentanil’s ester structure renders it susceptible to hydrolysis by blood- and tissue-nonspecific esterases, resulting in rapid metabolism and rapid reduction of blood concentration after an infusion has stopped ( Fig. 24.18 ). Remifentanil thus constitutes the first ultrashort-acting opioid for use as a supplement to general anesthesia.

Pharmacokinetic properties of remifentanil are best described by a three-compartment model. Its clearance is several times greater than normal hepatic blood flow, consistent with widespread extrahepatic metabolism. But, remifentanil is not significantly metabolized or sequestered in the lungs. It is a weak base with a pKa of 7.07. It is highly lipid-soluble with an octanol/water partition coefficient of 19.9 at pH 7.4. Remifentanil is highly bound (= 70%) to plasma proteins (mostly α 1 -acid glycoprotein). The remifentanil free base is formulated with glycine. Because glycine has been shown to act as an inhibitory neurotransmitter that causes a reversible motor weakness when injected intrathecally in rodents, remifentanil is not approved for spinal or epidural use.
The primary metabolic pathway of remifentanil is de-esterification to form a carboxylic acid metabolite, GR-90291 ( Fig. 24.19 ), which is 0.003 to 0.001 times as potent as remifentanil. The low in vivo potency of GR90291 can be explained by its low affinity to the μ-receptor in combination with a poor brain penetration. Excretion of GR-90291 is dependent on renal clearance mechanisms. Evidence from dogs suggests that the remifentanil metabolites are, for practical purposes, completely inactive, even in the face of renal failure. Its pharmacokinetics is not appreciably influenced by renal or hepatic failure. In blood, remifentanil is metabolized primarily by enzymes within erythrocytes. Remifentanil is not a good substrate for pseudocholinesterase and, therefore, is not influenced by pseudocholinesterase deficiency.

Surrogate Measures of Opioid Potency
Because a high-resolution measure of analgesia is not available, opioid potencies are usually estimated by some surrogate measures. Reduction of the MAC required to produce lack of movement to skin incision has been a frequently utilized surrogate measure in the estimation of opioid potency ( Fig. 24.20 ). However, MAC is not useful as a surrogate measure of opioid potencies outside the operation rooms.

To guide the administration of opioids in anesthetized patients, indirect parameters that reflect the patient’s physiologic reaction to nociception like sweating, movement, heart rate, and blood pressure should be monitored. However, the low specificity of these signs can cause underdosage or overdosage of intraoperative analgesia. The Analgesia Nociception Index (ANI), derived from an electrocardiogram trace, has been proposed as a noninvasive guide to analgesia. The ANI monitor calculates heart rate variation with respiration, a response mediated primarily by changes in the parasympathetic nervous system stimulation to the sinoatrial node of the heart. Patients receiving intraoperative ANI-guided fentanyl administration during sevoflurane anesthesia for lumbar discectomy and laminectomy demonstrated decreased pain in the recovery room, likely as a result of more objective intraoperative fentanyl administration.
The EEG has been another widely utilized surrogate measure in estimating opioid potency. The EEG is advantageous, because it is noninvasive and can be used as an effect measure when an experimental subject is unconscious or apneic. When processed by Fourier spectral analysis, the raw EEG changes translate into a significant decrease in the value of the spectral edge, a parameter that quantitates the frequency below which a given percentage (usually 95%) of the power in the EEG signal is found. Although the clinical meaning of the EEG changes produced by opioids is unclear, the opioid potencies estimated using the EEG as a surrogate measure appear to be clinically reliable because they relate to clinically determined potencies in a proportional, reproducible fashion. However, because the surrogate measures do not always assess the drug effect of clinical interest (analgesia), estimations of potency based on surrogate measures must be interpreted with caution.
Factors Affecting Pharmacokinetics and Pharmacodynamics of Opioids
Age
Pharmacokinetics and pharmacodynamics of opioids can be influenced by age. It is clear that neonates exhibit a reduced rate of elimination of essentially all opioids. This is presumably due to immature metabolic mechanisms, including the cytochrome P-450 system. The prolonged elimination of opioids observed in the neonatal period quickly normalizes toward adult values within the first year of life.
Intraoperative requirements of opioids are different between adults and children. The infusion rate of remifentanil to block somatic and autonomic response to skin incision was almost 2-fold higher in children (2-11 years) than adults (20-60 years). With advanced age, although pharmacokinetic changes may play a minor role, pharmacodynamic differences are primarily responsible for the decreased dose requirement in the elderly. Age was inversely correlated with central volume of distribution, clearance, and potency of remifentanil ( Fig. 24.21 ). These combined pharmacokinetic and pharmacodynamic changes mandate a reduction in remifentanil dosage by at least 50% or more in the elderly.

Body Weight
Many opioid pharmacokinetic parameters, especially clearance, appear to be more closely related to lean body mass. Total body weight-based dosing in an obese patient results in much higher remifentanil effect-site concentrations than does lean body mass-based dosing. In contrast, for lean patients, the concentrations that result from total body weight-based dosing are not much greater than those based on body mass ( Fig. 24.22 ). Clinically, context-sensitive half-times are not significantly different between obese and lean subjects ( Fig. 24.23 ). There is mounting evidence to suggest that lean body mass is a better predictor of metabolic capacity than total body weight. Ideal body weight, a parameter closely related to lean body mass and one that is perhaps more easily estimated by the clinician, is probably an acceptable alternative of lean body mass. Because obesity and obesity-associated disease have increased and continue to do so, obese patients will frequently present for anesthesia and operations. Understanding the influence of obesity on the disposition of opioids is an important question in contemporary anesthesia practice. In response, new pharmacokinetic models incorporating the influence of body mass have been recently reported.


Renal Failure
Renal failure has implications of major clinical importance with respect to morphine, hydromorphone, and meperidine. For the fentanyl congeners, the clinical importance of renal failure is less marked.
Morphine is an opioid with active metabolites that are dependent on renal clearance mechanisms for elimination. Morphine is principally metabolized by conjugation in the liver, and the water-soluble glucuronides (M3G and M6G) are excreted via kidney. The kidney also plays a role in the conjugation of morphine, accounting for nearly 40% of its metabolism. Patients with renal failure can develop very high levels of M6G and life-threatening respiratory depression ( Fig. 24.24 ). In view of these changes induced by renal failure, morphine is not considered a good choice in patients with severely altered renal clearance mechanisms. There are similar concerns for the use of hydromorphone in the setting of renal dysfunction.

The clinical pharmacology of meperidine is also significantly altered by renal failure. Because normeperidine, the main metabolite of meperidine with analgesic and CNS excitatory effect, is subject to renal excretion, the potential CNS toxicity secondary to normeperidine accumulation is especially a concern in patients in renal failure.
In contrast, the clinical pharmacology of the fentanyl congeners is not grossly altered by renal failure, although a decrease in plasma protein binding may potentially alter the free fraction of the fentanyl class of opioids. In the presence of renal impairment, none of fentanyl, alfentanil, sufentanil, and remifentanil deliver a high active metabolite load, or suffer from significantly prolonged clearance. Neither the pharmacokinetics nor the pharmacodynamics of remifentanil is altered by impaired renal function. Levels of GI-90291 that develop during a remifentanil infusion in patients in renal failure are not likely to produce any clinically significant effects.
Hepatic Failure
Even though the liver is the metabolic organ primarily responsible for opioid biotransformation, the degree of liver failure typically observed in perioperative patients, with the exception of patients undergoing liver transplantation, does not have a major impact on the pharmacokinetics of most opioids. In addition to reduced metabolic capacity (i.e., cytochrome P-450 system and conjugation), liver disease may also lead to reductions in hepatic blood flow, hepatocellular mass, and plasma protein binding. The increase in total body water and the edema of advanced liver disease may alter the distribution characteristics of a drug. Enzyme induction such as observed in early alcoholism can actually increase metabolic capacity of the liver.
Morphine pharmacokinetics is relatively unchanged by developing liver disease, such as liver cirrhosis and hepatic carcinoma, because of the substantial compensatory extrahepatic metabolism of morphine. A reduction in hepatic blood flow would be expected to slow the decline of morphine plasma concentrations. After liver resection, M6G/morphine and M3G/morphine ratios were significantly reduced and circulating morphine concentration was increased mainly due to a lower morphine clearance. In patients with cirrhosis, the metabolism of meperidine is decreased, leading to accumulation of the parent drug and possible CNS depressive effects similar to hepatic encephalopathy. Although the elimination of normeperidine is decreased as well in these patients, the ratio of normeperidine to meperidine is generally low, and the opioid effects of meperidine usually predominate. The disposition of fentanyl and sufentanil appears to be unaffected in liver diseases. Reductions in liver blood flow that result from either liver disease or some other disorder (e.g., shock) can affect the pharmacokinetic parameters of alfentanil, fentanyl, and sufentanil. It was demonstrated that there was a significant decrease in clearance of alfentanil in patients with mild to moderate cirrhosis compared with volunteers from the historical control group. Remifentanil is an opioid whose pharmacokinetics is completely unchanged by liver disease ( Fig. 24.25 ). Its kinetics does not change during the anhepatic phase of orthotopic liver transplantation. It was reported that 0.25 to 0.5 μg/kg/min remifentanil could provide perioperative analgesia without neurological deterioration in a patient suffering from chronic hepatic failure with mild encephalopathy.

In summary, a reduction in renal function may have the greatest effects on morphine due to a decrease in capacity for extrahepatic glucuronidation and clearance. Therefore, fentanyl may be a safer choice in renal failure patients due to their lack of active metabolites. In contrast, only under conditions of severe hepatic failure does one observe clinically significant changes in morphine or fentanyl clearance.
Cardiopulmonary Bypass
CPB produces significant alterations in the pharmacokinetics of most opioids. These alterations are a result of CPB-induced modifications in distribution volumes (secondary to priming), changes in acid-base balance, organ blood flow, plasma protein concentrations, and body temperature. The binding of drugs to components of the bypass circuit can also alter opioid pharmacokinetics.
When morphine is given as a premedicant before cardiac anesthesia, its concentrations decline significantly on initiation of CPB. Miller et al. examined the effect of CPB on plasma fentanyl concentration and showed that total concentration of fentanyl in plasma was significantly decreased and the unbound fraction of fentanyl rose on initiation of CPB. The total fentanyl concentration remained relatively stable during bypass until near the end of CPB when the mean total concentration increased, coinciding with rewarming. Population pharmacokinetic modeling applied to concentration-versus-time data from patients undergoing coronary artery bypass grafting using CPB demonstrated that the effect of CPB on fentanyl pharmacokinetics is clinically insignificant, and that a simple three-compartment model accurately predicts fentanyl concentrations throughout surgery using CPB. Elimination of alfentanil is prolonged by CPB primarily because of increased distribution. It was reported that the volume of distribution in steady state (Vd ss ) and the volume of central compartment for alfentanil were significantly greater in the CPB groups, compared to the nonbypass group. However, the elimination half-life (T1/2β) of alfentanil did not differ significantly between the normothermic CPB, hypothermic CPB, and nonbypass groups. Between the normothermic and hypothermic CPB groups no significant differences in Vd ss , clearance, or T1/2β were found. The free fraction of alfentanil during CPB remains constant despite complex changes in binding protein concentrations. In adult patients undergoing elective myocardial revascularization with hypothermic CPB who received continuous infusions of remifentanil 1.0 to 2.0 μg/kg/min, no evidence of accumulation or sequestration was found. Russell et al. reported that normothermic CPB did not significantly affect the clearance of remifentanil, but hypothermic CPB reduced it by an average of 20%, and this was attributed to the effect of temperature on blood and tissue esterase activity. In pediatric patients undergoing CPB for repair of atrial septal defect, there was no change in the Vd ss , the volume of the central compartment, T1/2α, and T1/2β, but clearance values of remifentanil increased 20% in the postbypass period. In patients undergoing coronary artery bypass graft surgery with hypothermic CPB receiving continuous infusion of remifentanil, the volume of distribution increased by 86% with institution of CPB and remained increased after CPB, and elimination clearance decreased by 6.37% for each degree from 37°C. Thus, although clearance of remifentanil reduces during CPB, remifentanil remains a very short-acting drug even during CPB.
Acid-Base Changes
It was demonstrated that pH changes influence the protein binding of fentanyl, sufentanil, and alfentanil, resulting in an increase in protein binding with alkalosis and a decrease with acidosis. These effects are greater for fentanyl than sufentanil and sufentanil than alfentanil. Relative changes in the free-drug fraction with pH variation from 7.4 to 7.0 were much higher for fentanyl (52%) when compared with sufentanil (29%) and alfentanil (6%). The pH dependence of plasma protein binding of the opioids significantly correlates with their partition between an organic and aqueous phase, suggesting the hydrophobic character of the interaction between plasma proteins and opioids. Increased ionization decreases the amount of fentanyl available for hepatic metabolism or renal excretion. Intraoperative hyperventilation during surgical procedures can significantly influence the pharmacokinetics of sufentanil resulting in an increased distribution volume and a prolonged elimination half-time.
Thus, both intraoperative respiratory alkalosis and respiratory acidosis, especially in the immediate postoperative period, can prolong and exacerbate opioid-induced respiratory depression.
Hemorrhagic Shock
It is a common practice to administer reduced doses of opioids to patients suffering from hemorrhagic shock to minimize adverse hemodynamic consequences and to prevent prolonged opioid effect. This is at least partially attributable to a pharmacokinetic mechanism. Analysis with pigs receiving fentanyl suggested that central clearance and central- and second-compartment distribution volumes were significantly reduced in hemorrhagic shock, resulting in higher fentanyl concentrations for any given dosages and prolonged context-sensitive half-time ( Fig. 24.26 ). Hemorrhagic shock also altered pharmacokinetics of remifentanil, suggesting that less remifentanil would be required to maintain a target plasma concentration ( Fig. 24.27 ). However, because of its rapid metabolism, changes in context-sensitive half-time are small. In a stepwise hemorrhage model of pigs receiving total intravenous anesthesia with remifentanil (0.5 μg/kg/min) and propofol (6 mg/kg/h after 2 mg/kg bolus), the plasma remifentanil concentration showed a threefold greater increase than that of propofol. Thus, the remifentanil dose should be reduced substantially compared with propofol during total intravenous anesthesia for patients with significant blood loss.


Genetic Variations in Opioid Metabolism
All opioid drugs are substantially metabolized mainly by cytochrome P450 (CYP) and to a lesser extent by UDP-glucuronosyltransferases (UGTs). Although CYP3A4 is involved in the metabolism of many of the opioids, it is the role of the highly polymorphic CYP2D6 that is of greater clinical interest with respect to the weaker opioids (codeine, dihydrocodeine, oxycodone, hydrocodone, and tramadol), because of the formation of their more potent hydroxyl metabolites (morphine, dihydromorphine, oxymorphone, and hydromorphone), which have about a 30-fold higher affinity for the μ-receptor. The CYP2D6 gene is highly polymorphic, with 100 allelic variants identified, and enzymatic activity of some alleles may be either significantly high or low. In the case of a variant more rapidly producing metabolites of higher affinity there exists the possibility of administering an unintended opioid overdose. This has been especially of concern for the use of codeine-containing products in pediatric populations resulting in changes in prescribing practice. Alternately, variants leading to low production of potent metabolites may result in subtherapeutic outcomes. Finally, UGTs mediate mainly the formation of glucuronides from buprenorphine, codeine, dihydrocodeine, dihydromorphine, hydromorphone, morphine, naloxone, naltrexone, and oxymorphone. UGT2B7 gene is polymorphic, and less than 20 allelic variants have been identified. It is possible that differences in metabolic activity among UGT alleles affect pharmacokinetics of opioids.
Anesthetic Techniques Using Opioids
Analgesia
Opioids are frequently used to relieve pain during monitored anesthesia care and regional anesthesia. A single bolus administration of opioids can provide significant pain relief. Morphine is slow in onset and does not allow rapid titration to effect. Meperidine (50-100 mg IV) produces variable degrees of pain relief and is not always effective in patients with severe pain. IV boluses of fentanyl (1-3 μg/kg), alfentanil (10-20 μg/kg), or sufentanil (0.1-0.3 μg/kg) can produce potent and short-lasting analgesia. Infusion rates range from 0.01 to 0.05 μg/kg/min for fentanyl, 0.0015 to 0.01 μg/kg/min for sufentanil, 0.25 to 0.75 μg/kg/min for alfentanil, and 0.05 to 0.25 μg/kg/min for remifentanil. Plasma concentrations of opioids necessary for various purposes are listed in Table 24.8 .
Fentanyl (ng/mL) | Sufentanil (ng/mL) | Alfentanil (ng/mL) | Remifentanil (ng/mL) | |
---|---|---|---|---|
Predominant agent | 15-30 | 5-10 | 400-800 | — |
Major surgery | 4-10 | 1-3 | 200-400 | 2-4 |
Minor surgery | 3-6 | 0.25-1 | 50-200 | 1-3 |
Spontaneous ventilation | 1-3 | <0.4 | <200 | 0.3-0.6 |
Analgesia | 1-2 | 0.2-0.4 | 50-150 | 0.2-0.4 |
Changes in the excitability of central neurons play an important role in establishment of pain. In rats, low doses of fentanyl block the synaptic form of central sensitization in the rat spinal cord in vivo, suggesting the possibility of preemptive analgesia by fentanyl, but higher doses do not have this effect. In fact, dose escalation of fentanyl or other potent opioids may produce a hyperalgesic state—mentioned previously. Reductions in postoperative pain and improved recovery have been attributed to preemptive analgesia with either epidural fentanyl or bupivacaine after radical prostatectomy. In contrast, in patients undergoing transperitoneal tumor nephrectomy, preoperative intravenous administration of a combination of morphine, ketamine, and clonidine failed to exert a clinically relevant effect on postoperative pain. Aida et al. reported that the effectiveness of preemptive analgesia varies according to the type of surgery, and preemptive analgesia with epidural morphine was reliably effective in limb and breast surgeries but ineffective in abdominal surgery. A meta-analysis demonstrated that the results of preemptive analgesia with systemic administration of opioids are equivocal. Thus, whether or not preemptive analgesia can be effectively achieved clinically by the early administration of opioids remains uncertain and may be highly context specific.
Patient-controlled analgesia (PCA) with opioids has become a widely accepted technique in which to provide postoperative analgesia, but pharmacokinetic optimization of opioid treatment in acute pain is a complex matter. Without considering effect-site drug concentrations over time, the choice of opioid and the amount, method, and frequency of its administration cannot be optimal. Morphine and fentanyl are often used for PCA therapy, and piritramide is also frequently used for PCA in European countries. A double-blind randomized study demonstrated that effect-site target-controlled remifentanil PCA with a slow and progressive adapted algorithm is feasible in young women undergoing uterine artery embolization. Combination of opioid with other drugs might be a method for improvement of PCA. For thoracic surgery, addition of ketamine to opioid for intravenous PCA was superior to intravenous PCA opioid alone, whereas the effect of added ketamine was not significant for orthopedic or abdominal surgery. Despite the utility of an opioid-based PCA, critical considerations must also be made as to an individual patient’s relative risk of opioid-induced respiratory depression with appropriate oversight and monitoring.
Sedation
Critically ill subjects in the intensive care unit (ICU) often experience anxiety and agitation while being exposed to numerous stressful or noxious stimuli. ICU patients generally require a combination of analgesia and sedation to relieve their state of anxiety, improve adaptation to the endotracheal tube, and aid compliance with mechanical ventilation. Morphine, fentanyl, and sufentanil are frequently used intravenous analgesic agents in the ICU. A randomized double-blind study indicated that remifentanil (0.15 μg/kg/min) and morphine (0.75 μg/kg/min) could provide comparable levels of sedation, and the remifentanil-based regimen allowed a more rapid emergence from sedation and facilitated earlier extubation. However, use of ultrapotent opioid agents such as remifentanil may also drive tolerance development and OIH—as discussed previously.
Balanced Anesthesia
Anesthesia with a single agent can often require doses that produce excessive hemodynamic depression. Alternately, “balanced anesthesia” with a balance of agents and techniques can be used to more selectively direct different components of anesthesia (i.e., analgesia, amnesia, muscle relaxation, and abolition of autonomic reflexes with maintenance of homeostasis). For example, inclusion of an opioid as a component of balanced anesthesia can reduce preoperative pain and anxiety, decrease somatic and autonomic responses to airway manipulations, improve hemodynamic stability, lower requirements for inhaled anesthetics, and provide immediate postoperative analgesia. Opioids interact synergistically and markedly reduce the dose of propofol and other sedative-hypnotics required for loss of consciousness and during noxious stimulation such as skin incision ( Fig. 24.28 ). Although the intent of combining opioids with sedative-hypnotics and/or volatile anesthetics is to produce anesthetic conditions with stable hemodynamics prior to as well as after noxious stimulation, this ideal is not always achieved.

The timing, rate of administration, and dose of supplemental opioids should also be tailored to the specific condition of the patient and the expected duration of the operation in order to avoid problems. Giving a large dose of any opioid shortly before the end of surgery is very likely to result in postoperative respiratory depression. However, analgesic concentrations of opioids have little effect on the MAC awake of inhaled anesthetics.
The ideal opioid would permit rapid titration, prevent unwanted responses to noxious stimuli, require little supplementation, not depress cardiovascular function, permit the return of adequate spontaneous ventilation in a timely manner, and produce effective postoperative analgesia with minimal side effects. Alfentanil and remifentanil provide the greatest ability to titrate opioids rapidly because of their extremely rapid time to onset (1-2 minutes) of peak effect. Sufentanil, alfentanil, and remifentanil are arguably superior to fentanyl in most respects. Antagonism of opioid action with naloxone for troublesome respiratory depression is required less frequently after alfentanil and sufentanil compared with fentanyl. Pharmacologic antagonism is not required after remifentanil administration because of its short half-life.
Fentanyl
Anesthetic induction is usually achieved by combining a loading dose of fentanyl (2-6 μg/kg) with a sedative-hypnotic, most commonly thiopental or propofol, and a muscle relaxant. Maintenance of anesthesia can be achieved with low concentrations of potent inhaled anesthetics, and additional fentanyl (intermittent boluses of 25-50 μg every 15-30 minutes or a constant infusion of 0.5-5.0 μg/kg/h).
The plasma concentration of fentanyl required for postoperative analgesia was approximately 1.5 ng/mL. MAC of isoflurane at skin incision can be reduced by 50% and 63% with plasma fentanyl concentrations of 1.67 and 3.0 ng/mL, respectively. Increasing plasma fentanyl concentrations from 3.0 to 10 ng/mL only further reduced the MAC of isoflurane from 63% to 82%. Intraoperative requirement of propofol for hypnosis is also reduced by fentanyl. In patients undergoing spine fusion, to keep the mean arterial pressure within 15% of the control value when fentanyl was infused to keep the plasma concentration at 0, 1.5, 3.0, and 4.5 ng/mL, average propofol infusion rates were 10.1 ± 2.5 (mean ± SD), 7.5 ± 1.2, 5.7 ± 1.1, and 4.9 ± 1.2 mg/kg/h, respectively.
Opioid pharmacokinetics and pharmacodynamics vary considerably among patients. It was reported that fentanyl dose based on total body weight may cause overdosing in obese patients. As discussed previously, considerations for dosing based on lean body weight and/or ideal body weight may have merit in this context. Nevertheless, a balanced technique with fentanyl, titrating the opioid in anticipation of various stimuli and patient responses with pharmacokinetic guidelines in mind, will often result in a stable hemodynamic course and rapid awakening in a pain-free patient. Repeated doses or continuous infusions of fentanyl are most likely to result in significant depression of spontaneous ventilation.
Alfentanil
Because alfentanil penetrates the brain so rapidly, equilibration of alfentanil between plasma and CNS can be achieved while plasma alfentanil levels are relatively low compared with sufentanil and fentanyl. This property explains how low doses (10-30 μg/kg) of alfentanil, administered just before or simultaneously with a sedative-hypnotic, are effective.
Alfentanil (25-50 μg/kg IV), followed by small titrated sleep doses of any sedative-hyponic (e.g., 50-100 mg sodium thiopental), is usually successful in preventing significant hemodynamic stimulation from laryngoscopy and intubation. The optimum dose of alfentanil, coadministered with 2.5 mg/kg propofol, when inserting a classic laryngeal mask airway was 10 μg/kg. Further opioid supplementation can be achieved with an alfentanil infusion (0.5-2.0 μg/kg/min) or intermittent boluses of alfentanil (5-10 μg/kg) for shorter procedures. In balanced anesthetic techniques in which potent inhaled anesthetics are also employed, relatively low plasma alfentanil concentrations (e.g., 29 ng/mL) can reduce the MAC of isoflurane by approximately 50%. The EC50 of alfentanil during propofol anesthesia, in which plasma concentration of propofol was kept at target concentrations of 3 μg/mL, was 92 ng/mL for intubation, 55 ng/mL for skin incision, 84 ng/mL for the opening of the peritoneum, and 66 ± 38 ng/mL for the intraabdominal part of surgery. It was reported that hemodynamic changes induced by propofol might have an important influence on the pharmacokinetics of alfentanil. Propofol (target concentration, 1.5 μg/mL) decreased the elimination clearance of alfentanil by 15%, rapid distribution clearance by 68%, slow distribution clearance by 51%, and lag time by 62%. Alfentanil infusions or repeated doses should be minimized 15 to 30 minutes prior to the end of surgery in order to avoid problematic residual respiratory depression.
Sufentanil
CP 50 for prevention of hemodynamic responses to laryngoscopy and tracheal intubation was 1.08 ng/mL with a range of 0.73 to 2.55 ng/mL. In combination with propofol for induction of anesthesia in children, bolus administration of sufentanil 0.3 μg/kg can completely abolish the cardiovascular response to tracheal intubation. In healthy normotensive adult patients, a bolus sufentanil 0.1 μg/kg followed by a continuous infusion at 0.08 μg/kg/min for at least 5 minutes proved to be effective for blunting the cardiovascular response to intubation. Maintenance of anesthesia can be achieved with sufentanil (intermittent boluses, 0.1-0.25 μg/kg, or constant infusion, 0.5-1.5 μg/kg/h). The CP 50 for sufentanil during skin incision (2.08 ± 0.62 ng/mL) is twice as great as that for intubation in unpremedicated patients. CP 50 value ratios at skin incision for sufentanil, fentanyl, and alfentanil are approximately 1:2:150 and represent different, and probably more accurate, potency ratios than those traditionally published based on drug dose. In patients undergoing coronary artery bypass grafting, sufentanil greater than 1.25 ± 0.21 ng/mL reduced isoflurane requirements to less than 0.5% through the operation.
Remifentanil
Very short duration of action of remifentanil mandates that an infusion (0.1-1.0 μg/kg/min) be started prior to or soon after a small bolus dose to ensure sustained opioid effect. Maintenance infusion rates of remifentanil range from 0.1 to 1.0 μg/kg/min for balanced anesthesia. Remifentanil can reliably suppress automatic, hemodynamic, and somatic responses to noxious stimulation and allows the most predictable and rapid trouble-free emergence from anesthesia without postoperative respiratory depression. Infusion rates of 0.1 ± 0.05 μg/kg/min should permit the return of spontaneous ventilation and responsiveness with maintenance of analgesia. A randomized, double-blind, placebo-controlled study demonstrated that combination of 0.05-0.1 μg/kg/min remifentanil and 2 mg midazolam provided effective sedation and analgesia during outpatient surgical procedures performed under local anesthesia.
Associated with emergence from remifentanil anesthesia, the need for alternative analgesic therapies should be anticipated and administered in a timely fashion. Exposure to high doses of remifentanil may paradoxically reduce the pain threshold after its discontinuation, resulting in postoperative hyperalgesia, which is known to be associated with acute and persistent pain. It was reported that perioperative administration of morphine (0.15 or 0.25 mg/kg IV) or fentanyl (0.15 mg) did not provide entirely adequate immediate postoperative pain control after remifentanil-based anesthesia in major abdominal surgery. Administration of ketamine (0.15 mg/kg followed by 2 μg/kg/min) decreased intraoperative remifentanil use during abdominal surgery and postoperative morphine consumption without increasing the incidence of side effects. In children undergoing strabismus surgery, combination of sevoflurane (2.5%) and remifentanil (1 μg/kg followed by 0.1-0.2 μg/kg/min) showed less frequent postoperative vomiting but higher postoperative pain scores compared with fentanyl (2 μg/kg followed by 1 μg/kg every 45 minutes).
Postoperative pain relief with low-dose remifentanil infusion was also reported. After general anesthesia with propofol (75 mg/kg/min) and remifentanil (0.5-1.0 mg/kg/min) for abdominal or thoracic surgery, continuous infusion of remifentanil (0.05 or 0.1 mg/kg/min) provided adequate analgesia.
Total Intravenous Anesthesia
Many different IV anesthetic and analgesic compounds can be employed in a number of combinations to provide TIVA. Most commonly, an opioid is combined with another drug more likely to provide hypnosis and amnesia. For example, combination of alfentanil and propofol can produce desirable TIVA. Alfentanil provides analgesia and hemodynamic stability while blunting responses to noxious stimuli. On the other hand, propofol provides hypnosis and amnesia and is antiemetic. Anesthetic induction with alfentanil (25-50 μg/kg) and propofol (0.5-1.5 mg/kg) followed by infusions of 0.5 to 1.5 μg/kg/min of alfentanil and 80 to 120 μg/kg/min of propofol will produce complete anesthesia for a variety of procedures. It is proposed that alfentanil concentrations as low as 85 ng/mL, when combined with a blood propofol concentration of 3.5 μg/mL, can produce both optimal anesthetic conditions and speed of recovery. Stanski and Shafer suggested that bolus doses and initial infusion rates would be 30 μg/kg and 0.35 μg/kg/min for alfentanil and 0.7 mg/kg and 180 μg/kg/min for propofol. Recognizing that these calculations were based on EC 50 data in patients undergoing only moderately painful procedures, anesthesiologists should adjust these doses accordingly. In patients undergoing ear-nose-throat surgery, TIVA with remifentanil and propofol provided a more rapid respiratory recovery after brief surgical procedures, compared with TIVA with alfentanil and propofol.
Maintenance infusions vary according to patient condition and surgical stimuli. Propofol (75-125 μg/kg/min) and alfentanil (1.0-2.0 μg/kg/min) were initially recommended. Propofol infusions should be terminated 5 to 10 minutes before anticipated patient awakening. Alfentanil infusion rates do not need to be less than 0.25 to 0.5 μg/kg/min until surgery is terminated. A multicenter evaluation demonstrated that in patients of elective inpatient surgery remifentanil 1 μg/kg IV followed by 1.0 μg/kg/min, when combined with propofol 75 μg/kg/min, effectively controlled responses to tracheal intubation. Reduction of remifentanil infusion rate to 0.25-0.40 μg/kg/min after tracheal intubation was also recommended. Although midazolam-opioid combinations can also provide complete anesthesia, midazolam-alfentanil TIVA has not been found to compare favorably to propofol-alfentanil TIVA even with flumazenil reversal of benzodiazepine actions.
TIVA techniques are especially useful when delivery of inhaled agents is compromised. By keeping the goals of balanced anesthesia in mind, combining modern opioids and other drugs, utilizing infusion pumps, and employing an increased understanding of pharmacokinetics, clinicians can successfully perform a variety of TIVA techniques. Approximate opioid doses and infusion rates for TIVA are listed in Table 24.9 .
Loading Dose (μg/kg) | Maintenance Infusion Rate | Additional Boluses | |
---|---|---|---|
Alfentanil | 25-100 | 0.5-2 μg/kg/min | 5-10 μg/kg |
Sufentanil | 0.25-2 | 0.5-1.5 μg/kg/h | 2.5-10 μg |
Fentanyl | 4-20 | 2-10 μg/kg/h | 25-100 μg |
Remifentanil | 1-2 | 0.1-1.0 μg/kg/min | 0.1-1.0 μg/kg |
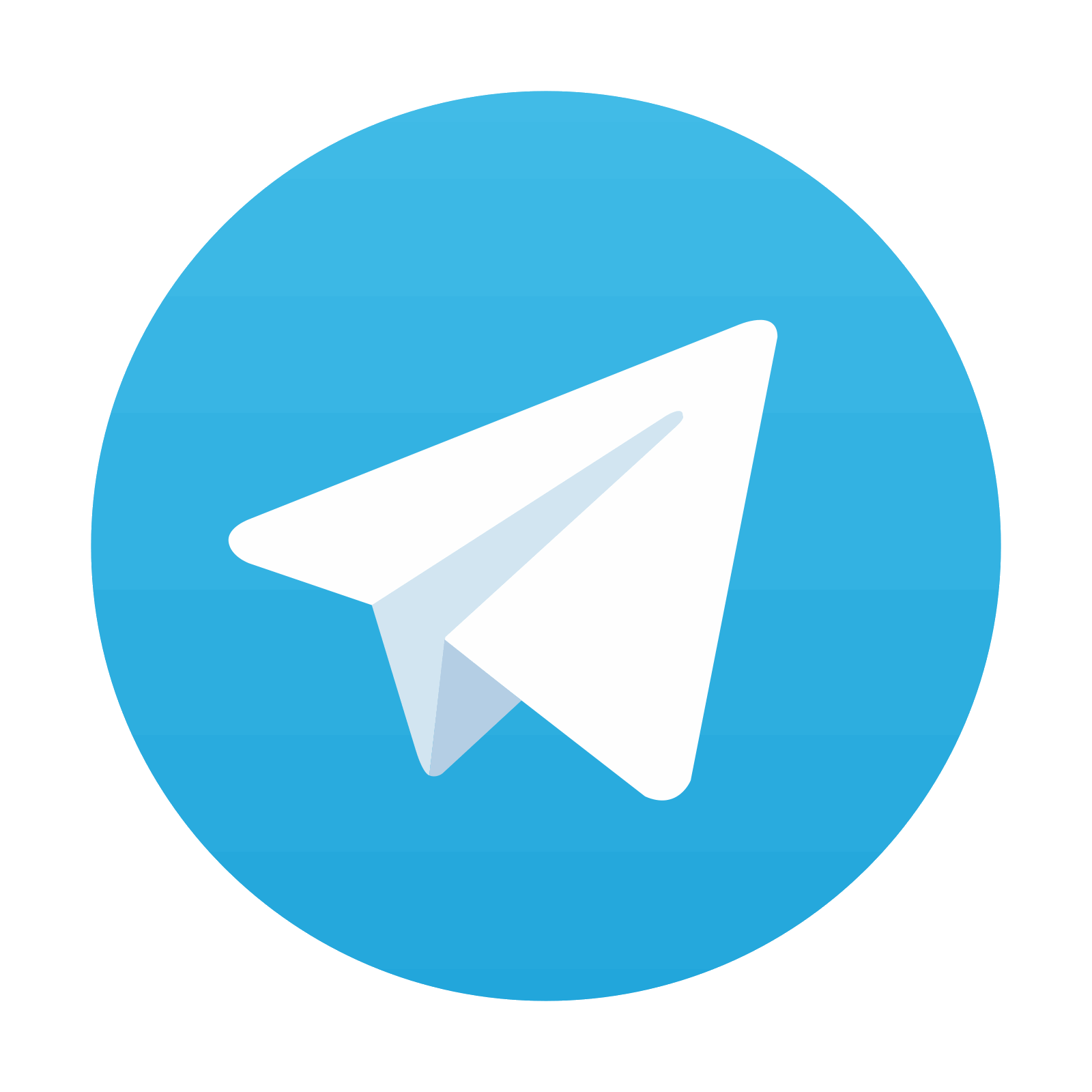
Stay updated, free articles. Join our Telegram channel
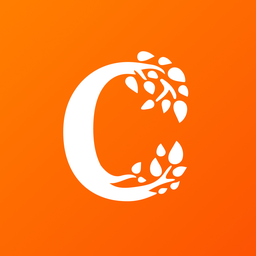
Full access? Get Clinical Tree
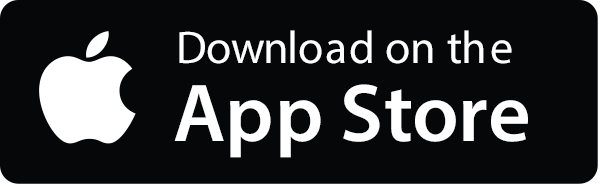
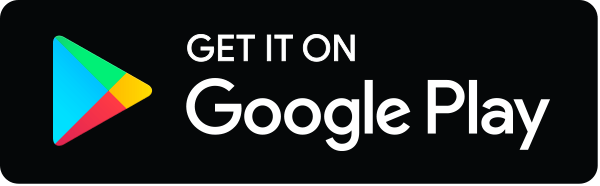
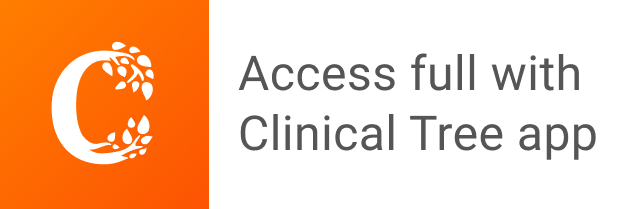