Pearls
- •
Pulse oximetry is based on the principles that the pulsatile component of the optical absorbance detected from tissue is primarily from arterial blood and that oxyhemoglobin and reduced (deoxy-)hemoglobin have different optical absorption spectra.
- •
Limitations of pulse oximetry include motion artifact, effects of ambient light, presence of pigmentation or dyes, low perfusion states, and dyshemoglobinemia.
- •
Most pulse oximeters measure only oxyhemoglobin and deoxyhemoglobin. However, blood CO-oximeters and noninvasive CO-oximeters can account for other absorbing species such as methemoglobin and carboxyhemoglobin.
- •
Capnography can be a good global indicator of the patient’s condition and can detect alveolar hypoventilation before changes detected by pulse oximetry.
- •
The accuracy of the capnogram depends on the sampling site. If the tidal volume is small and the sample flow rate is large, the gas sample may be diluted by entrained fresh gas.
Noninvasive monitoring in the form of vital signs (i.e., heart rate, respiratory rate, noninvasive blood pressure, fluid intake and output, and temperature) has been used routinely for all patients receiving care in the intensive care unit (ICU) since the birth of the specialty. Guidelines for equipment and monitoring and for levels of care for pediatric ICUs (PICUs) were specified by the American College of Critical Care Medicine in 2004. The majority of children admitted to the PICU present with cardiorespiratory disease or with an acute illness that may progress to involve the respiratory system, emphasizing the specific need for careful monitoring of respiratory parameters. Close respiratory examination and monitoring allow titration of therapies to minimize oxygen toxicity, minimize ventilator-induced injury, optimize patient-ventilator interaction, and aid in weaning from the ventilator. Pulse oximetry and capnometry have significantly affected the practice of critical care medicine and are now standards of care. New technologies currently under development to noninvasively monitor physiologic function may significantly decrease the need for more invasive monitoring and lessen the associated risks of such modalities.
Pulse oximetry
Pulse oximetry is a significant technologic advance that has improved patient safety. Its ease of application and accuracy have resulted in widespread use, and it is now a standard monitoring modality for many aspects of medical care. Pulse oximetry allows for earlier detection of hypoxemia than clinical examination and aids the clinician in the identification and treatment of hypoxemia, with possible prevention of serious complications. In the ICU, pulse oximetry is also used to titrate oxygen therapy in patients, including those undergoing mechanical ventilation. Within pediatrics, pulse oximetry is now used for neonatal congenital heart disease screening.
Standard pulse oximeters are not accurately calibrated for the low saturations seen with some congenital cardiac lesions. However, there is a “blue” sensor that is more accurate in the range of saturations encountered in patients with cyanotic congenital heart disease. Accurate pulse oximetry is especially vital in the neonatal population, who benefit from tight control of oxygenation in order to minimize oxidative stress and to decrease the risk of retinopathy of prematurity. Additionally, tight control of oxygenation (94%–99%) is now included in the Pediatric Advanced Life Support (PALS) guidelines for pediatric resuscitation.
Principles of pulse oximetry
Pulse oximetry is based on the principles that (1) the pulsatile optical absorbance detected in biological tissue is primarily due to arterial blood and that (2) oxyhemoglobin and reduced (deoxygenated) hemoglobin have different optical absorption spectra. The attenuation of light passing through blood-perfused tissue changes with pulsation of blood and the alternating component of the light attenuation results from the composition of arterial blood. Fig. 43.1 is a schematic diagram showing that the component of light attenuation as a result of pulsatility comes from arterial blood. This information can be analyzed to determine the hemoglobin saturation in the arterial blood. Absorption of light as a result of other tissue components and capillary and venous blood in the static (nonpulsatile) portion of the signal is ignored in the analysis.

Pulse oximeters typically use red (660-nm) and infrared (940-nm) wavelengths of light to determine the ratio of oxygenated to deoxygenated blood. Deoxygenated blood absorbs more red light, whereas oxygenated blood absorbs more infrared light. The two wavelengths are passed through an arterial bed, and the ratio of infrared and red light transmitted to the photodetector is determined. The absorption around 940 nm is relatively low and fairly constant over the range of saturations. Thus, a change in absorbance at 660 nm can be referenced to the absorption at the 940-nm wavelength and is used to determine the saturation. The ratio is calibrated against measurements of arterial oxygen saturations from human volunteers and their absorbance ratios. Each pulse oximeter uses a complex algorithm to convert the change in absorbance at the two wavelengths to an absolute saturation value. More wavelengths can be used to improve the accuracy of the measurement.
Hemoglobin has characteristic light-absorbing properties that change with oxygen binding. Fig. 43.2 shows absorption spectra of oxyhemoglobin and deoxyhemoglobin in the visible and near-infrared spectral region. At any given wavelength, there is a difference in absorption between oxyhemoglobin and deoxyhemoglobin except where the spectra cross at wavelengths called isosbestic wavelengths , where the absorption is the same for each state. At nonisosbestic wavelengths, the difference in absorption can be used to determine the fraction of oxyhemoglobin. Saturation of hemoglobin is defined as follows:

where Hb sat is fractional saturation of hemoglobin, [ OxyHb ] is concentration of oxyhemoglobin, and [ DeoxyHb ] is concentration of deoxyhemoglobin. Hemoglobin percent saturation, as commonly reported, is determined by multiplying Hb sat by 100.
In the presence of other forms of hemoglobin, primarily carboxyhemoglobin or methemoglobin, the saturation of hemoglobin is correctly determined by the more complex relationship:
where [ MetHb ] is concentration of methemoglobin and [ CarboxyHb ] is concentration of carboxyhemoglobin. Most pulse oximeters cannot accurately account for the presence of these other forms of hemoglobin. Blood CO-oximeters, however, do account for these species, as do the latest generation of multiwavelength pulse oximeters.
Validation
Numerous studies have been performed to validate existing pulse oximeters. , Pulse oximeters also must be subjected to extensive testing before obtaining US Food and Drug Administration (FDA) approval for marketing in the United States. Despite all of the current testing, difficulties in both calibration and validation remain. One of the most significant issues surrounding calibration is the development of an appropriate universal test that will accurately test the pulse oximeter for a wide range of potential clinical applications. Pulse oximeters must be accurate for a wide range of skin thickness and color, and over a wide range of saturations. In general, pulse oximeters are most accurate at higher saturations, usually above 75%.
Sources of error
Although pulse oximetry is widely accepted as a valid clinical monitor and provides valuable instantaneous clinical data, pulse oximeters are subject to multiple potential sources of error. The accuracy (difference between peripheral capillary oxygen saturation [Sp o 2 ] and arterial oxygen saturation [Sa o 2 ]) reported by manufacturers is approximately 2%. Studies evaluating the performance of pulse oximetry in clinical applications have demonstrated an accuracy of 0.02% to 4% for a single Sp o 2 measurement. , , , It is important to note that these values are based on measurements when patient Sa o 2 was above 90% and that precision of Sp o 2 worsens as Sa o 2 decreases. Additionally, Sa o 2 and partial pressure of arterial oxygen (Pa o 2 ) are not linearly related; the oxyhemoglobin dissociation curve is sigmoid in shape ( Fig. 43.3 ). Large changes in Pa o 2 at high levels of oxygen—the upper flat portion of the oxyhemoglobin dissociation curve—occur with little change in saturation. Saturations measured by pulse oximetry overestimate Sa o 2 in the range of 76% to 90%, on average, and the accuracy of pulse oximetry falls with arterial oxygen saturations less than 70%. , At arterial oxygen saturations below 70%, pulse oximetry may be more appropriate for showing trends.

Of particular note to ICU providers, low peripheral perfusion and motion artifact are the most common causes of inaccurate pulse oximetry readings. , , Pulse oximetry sensors may be unable to distinguish a true signal from background in low perfusion states that result in diminished pulsations (e.g., vasoconstriction, low cardiac output, and hypothermia). This situation is usually displayed as an inadequate pulse message. Newer designs of pulse oximeters with signal-progressing algorithms that detect and ignore motion and pulse rate interferences help overcome these limitations. , Recently, pulse oximeter probes that use reflectance technology have been developed for use on the forehead. , These pulse oximeters are designed so that both light-emitting diodes and detectors are on the same surface, decreasing scattering of light through the tissue as compared to traditional probes. , In studies of critically ill patients with poor perfusion, forehead reflectance probe oximetry measurement was found to be more accurate than finger probe. , , While limited, data suggest that forehead reflectance sensors are comparable in accuracy to digit sensors in pediatric patients.
Other sources of error include interfering dyes, other pigments in the blood and ambient light. The extent of ambient light interference has been questioned for some of the pulse oximeters studied, and shielding of the probe from ambient light is often used clinically to improve performance. Intravenous dyes and certain colors of nail polish may falsely lower pulse oximetry readings.
The presence of dyshemoglobinopathies is an infrequent clinical problem but can result in erroneous pulse oximetry readings. Abnormal hemoglobin levels (carboxyhemoglobin, methemoglobin) that have similar absorbance spectra can lead to overestimation of the true Sa o 2 . In methemoglobinemia, the iron in the heme groups in hemoglobin becomes oxidized from the ferrous (Fe 2+ ) state to the ferric (Fe 3+ ) state, which results in hemoglobin being unable to bind oxygen. The presence of significant quantities of methemoglobin leads to tissue hypoxia because these molecules no longer participate in oxygen transport. However, light absorbance by methemoglobin more closely resembles oxyhemoglobin than deoxyhemoglobin at the measured wavelengths, erroneously leading the pulse oximeter to indicate a higher percentage of oxygen saturation than expected. , Similarly, the presence of carboxyhemoglobin may result in an erroneous reading in pulse oximetry because carbon monoxide–bound hemoglobin also does not participate in oxygen transport.
To address this source of error, multiwavelength oximeters that can specifically detect carboxyhemoglobin and methemoglobin have been developed. Masimo has developed a pulse oximeter, known as the Masimo Rainbow SET Rad 57, that uses eight wavelengths of light and is capable of determining levels of oxyhemoglobin, deoxyhemoglobin, carboxyhemoglobin, and methemoglobin. Because these other species of hemoglobin are recognized, it is also possible to have a continuous readout of total hemoglobin. Since its development, multiple studies have been performed to determine the accuracy of the Rad 57 and potential for clinical application. In healthy volunteers, the Rad 57 was found to measure carboxyhemoglobin levels within the range of 0% to 15% with an uncertainty of ±2% and measure methemoglobin levels within the range of 0% to 12% with an uncertainty of 0.5%. , Data from patients suspected of carbon monoxide poisoning have demonstrated varied degrees of difference between carboxyhemoglobin levels measured by Rad 57 pulse oximetry and laboratory carboxyhemoglobin levels, ranging from 1.4% to 4.2%. , For patients seen in the emergency department, large limits of agreement between measurement were noted (−11.6% to 14.14%). Based on these findings, some authors have suggested that carboxyhemoglobin level measurement by CO-oximetry may not be interchangeable with laboratory evaluation and that further studies are required. , Because CO-oximeters account for the presence of both carboxyhemoglobin and methemoglobin, blood gas samples sent for CO-oximetry should correctly measure hemoglobin saturation in cases in which measurable levels of either methemoglobin or carboxyhemoglobin are present or suspected.
Probe placement
Pulse oximetry probes typically are placed on fingers or toes, with the light-emitting diodes placed across the digit, opposite from the detector. For premature and small infants, the probe often is placed around the entire palm or foot with good results. In larger pediatric patients, the nasal ala may also be used. Transesophageal probes have been designed and are used for care of operative or critically ill patients with potentially poor peripheral perfusion. The complications of pulse oximetry are rare. They include skin burns and pressure necrosis in newborns. , Limited understanding of pulse oximetry by healthcare providers may be an underrecognized problem, along with time spent determining whether alarms are false. ,
Tissue oximetry
In theory, measurement of tissue oxygenation will allow the clinician to fully assess oxygen delivery and utilization as opposed to solely oxygen content of the blood. Because oxygen delivery is dependent on cardiac function, a primary benefit of tissue oximetry measurements may be for assessment of perfusion and cardiovascular function. A number of technologies have been developed in order to measure the oxygenation of tissues themselves, including near-infrared spectroscopy (NIRS), direct tissue partial pressure of oxygen (P o 2 ) measurement with an oxygen electrode, transcutaneous tissue electrodes, microdialysis, and electron paramagnetic resonance. Within pediatric ICU medicine, the use of NIRS is center and provider dependent, with many variations in practice.
Near-infrared spectroscopy
NIRS is a noninvasive method used to measure the Hgb-oxygen saturation of a local region of interest. This is achieved through the use of multiple (2 to 4) wavelengths of near-infrared light directed via a cutaneous probe into the underlying tissue, which are absorbed by pigments such as myoglobin, hemoglobin, and cytochrome. In contrast to pulse oximetry, NIRS sampling is not specific to pulsatile blood flow and captures measurements from arterial, capillary, and venous blood compartments. Cerebral NIRS oximeters are calibrated by manufacturers with the assumption that the contribution of venous blood to oximetry readings is approximately 70% to 75% and the displayed SaO 2 is determined through an algorithm. , Accordingly, this may be a source of error.
Studies have reported varying degrees of accuracy for cerebral NIRS oximeters and have also found large variation in reading errors. Authors of these studies have postulated that this is possibly due to variations in the ratios of cerebral venous and arterial blood volume in response to alterations of blood oxygen and carbon dioxide (CO 2 ) levels, violating the assumption of constant ratios of 70:30 or 75:25. , Extracranial tissue changes due to superficial vasoconstriction secondary to vasoactive medication (e.g., norepinephrine, phenylephrine) or sympathetic response to pain or hypothermia can be additional sources of error with cerebral NIRS oximetry. Based on these findings, many authors recommend the use of cerebral oximetry as trend monitors and not absolute tissue oxygenation measures or injury threshold determinants. ,
Capnometry and capnography
Another monitoring technology routinely used in the ICU is the measurement of CO 2 . Capnometry is the measurement of the partial pressure (or concentration) of CO 2 in the patient’s airway during the entire ventilatory cycle. A capnometer provides a numeric measurement of inspired and expired, proximal end-tidal CO 2 (P etco 2 ). Capnography is the graphic display of the partial pressure or concentration of CO 2 as a waveform (capnogram), usually plotted as P co 2 versus time ( Fig. 43.4 ). When the waveform display is calibrated, capnography includes capnometry.

Operating principles of capnometry
Sampling of exhaled CO 2 can be at the patient-ventilator interface (mainstream), diverted to a monitor (sidestream), or an intermediate connection. In the most common sampling method, gas is diverted from the airway and aspirated through a tube (sidestream) to the CO 2 monitor. A low dead space sidestream CO 2 monitor is optimal for use in patients weighing less than 10 kg. An alternative to the diverting instrument is the nondiverting—or “mainstream”—capnometer, in which a special flow-through adapter and CO 2 monitor are placed in the patient’s airway. The exhaled gas sample is exposed to various wavelengths of infrared light. The relative amount of light absorbed by the exhaled sample is compared with the amount of light absorbed by a sample that does not contain CO 2 . By comparing the difference in absorption between the two samples, the capnometer determines the amount of CO 2 in the sample gas, which it then displays as the CO 2 concentration. CO 2 also can be measured semiquantitatively using a pH-sensitive indicator that changes from purple to yellow when exposed to CO 2 . The Easy Cap P etco 2 detector is placed between the endotracheal tube and resuscitation bag after intubation; a color change from purple to yellow should be observed. Up to six breaths may be necessary to allow for washout of retained CO 2 in the event of esophageal intubation. A constant purple color indicates that the tube is not in the trachea or the presence of poor pulmonary perfusion. A tan color may indicate tracheal intubation with poor pulmonary perfusion or esophageal intubation with retained CO 2 .
Clinical and technical issues
Both physiologic anomalies and technical factors can result in P etco 2 values that do not approximate partial pressure of arterial carbon dioxide (Pa co 2 ). For P etco 2 to approximate Pa co 2 , two assumptions must be met: (1) the lung units must empty synchronously with uniform time constants and (2) ventilation and perfusion must be well matched in the lung units. Additionally, technical variables can produce P etco 2 values that do not approximate Pa co 2 . These include the design of the gas sampling system, distance the gas must be transported, and the instrument’s calibration methods.
Gas sampling issues
The gas sampling method used by a capnometer affects the accuracy of the capnogram and P etco 2 measurements. Relevant factors include the location of the ventilatory circuit from which the gas is sampled, the distance over which the gas is transported before analysis, and the sample flow rate of the instrument. With a nondiverting or mainstream device, the CO 2 monitor is placed on the airway so that there is no need to divert gas from the airway. This sampling configuration typically is available only in infrared capnometers because only infrared CO 2 monitors can be designed small enough to fit on the airway. A study comparing mainstream P etco 2 to a novel method that sampled distal end-tidal CO 2 via a special double-lumen tube found that the distal end-tidal samples had the best correlation with Pa co 2 and remained reliable even when severe lung disease was present. Another sampling configuration is seen in the proximal-diverting device. A lightweight, low-profile airway adapter is placed in the patient’s airway, and gas is sampled from the airway and transported to the sensor, which is placed near the patient but not in the airway itself. A third sampling configuration is found in the distal-diverting device, the classic sidestream capnometer. In a distal-diverting system, gas is sampled from the airway and transported to the CO 2 monitor, which is located in the display unit distal to the patient.
The accuracy of the capnogram, P etco 2 measurements, and displayed values depends on the sampling site ( Fig. 43.5 ). In continuous gas flow circuits, sampling in or at the endotracheal tube results in the most accurate values because there is little contamination with fresh gas from the breathing circuit (point A in Fig. 43.5 ). The Y-connector of the breathing circuit is the next best sampling site (point B in Fig. 43.5 ). However, if the fresh gas flow is large compared with the expiratory flow rate of the patient (as may be the case in neonates and small children), the capnogram and P etco 2 values may be distorted as a result of dilution with the fresh gas flowing through the Y-connector. If gas is sampled “downstream” from the patient, the waveform and P etco 2 are increasingly diluted by fresh gas from the circuit (points C and D in Fig. 43.5 ). If gas is sampled “upstream” from the patient in the fresh gas supply, none of the exhaled CO 2 is detected and the measured P etco 2 is zero (point E in Fig. 43.5 ). Therefore, the best sampling site is within the patient’s endotracheal tube or at the tube connector, as far as possible from the Y-connector of the breathing circuit.
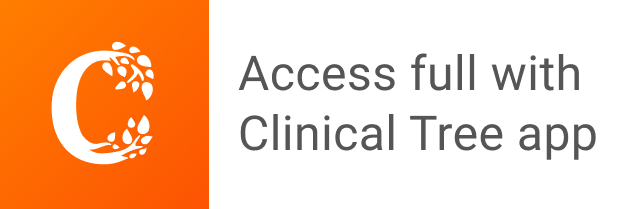