Clinical observation provides vital information regarding the patient. Observations gained from the use of the various monitors should augment that information; skin perfusion, capillary refill, cyanosis, pallor, skin temperature and turgor, chest movement and heart auscultation are just a few examples. The equipment used to monitor the patient is becoming more sophisticated. It is vital that the clinician using these monitors is aware of their limitations and the potential causes of error. Errors can be due to patient, equipment and/or sampling factors.
Monitoring equipment can be invasive or non-invasive. The latter is discussed in this chapter, whereas the former is discussed in Chapter 11 .
Integrated monitoring
Until recently, it was common to see the anaesthetic machine adorned with discrete, bulky monitoring devices. Significant advances in information technology have allowed an integrated monitoring approach to occur. Plug-in monitoring modules feed a single visual display on which selected values and waveforms can be arranged and colour-coded ( Figs 10.1–10.3 ).



Although some would argue that such monitoring systems are complex and potentially confusing, their benefits in terms of flexibility and ergonomics are undisputed.
More recently, wireless monitoring systems are becoming available. An example is wireless invasive pressure monitoring systems ( Fig. 10.4 ). This reduces the clutter of cables surrounding the patients.

Electrocardiogram (ECG)
This monitors the electrical activity of the heart with electrical potentials of 0.5–2 mV at the skin surface. It is useful in determining the heart rate, ischaemia, the presence of arrhythmias and conduction defects. It should be emphasized that it gives no assessment of cardiac output.
The bipolar leads (I, II, III, AVR, AVL and AVF) measure voltage difference between two electrodes. The unipolar leads (V1–6) measure voltage at different electrodes relative to a zero point.
Components
- 1.
Skin electrodes detect the electrical activity of the heart ( Fig. 10.5 ). Silver and silver chloride form a stable electrode combination. Both are held in a cup and separated from the skin by a foam pad soaked in conducting gel. Changes in potential difference at the skin lead to polarization of the silver/silver chloride electrode.
Fig. 10.5
An ECG electrode.
- 2.
Colour-coded cables transmit the polarization signal from electrodes to the monitor. Cables are available in 3- and 5-lead versions as snap or grabber design and with a variety of lengths. All the cables of a particular set should have the same length to minimize the effect of electromagnetic interference.
- 3.
The ECG signal is then boosted using an amplifier. The amplifier covers a frequency range of 0.05–150 Hz. It also filters out some of the frequencies considered to be noise. The amplifier has ECG filters that are used to remove the noise/artefacts from ECG and produce a ‘clean’ signal.
- 4.
An oscilloscope displays the amplified ECG signal. A high-resolution monochrome or colour monitor is used.
Mechanism of action
- 1.
Proper attachment of ECG electrodes involves cleaning the skin, gently abrading the stratum corneum and ensuring adequate contact using conductive gel. Skin impedance varies at different sites and it is thought to be higher in females. The electrodes are best positioned on bony prominences to reduce artefacts from respiration.
- 2.
Modern ECG monitors use multiple filters for signal processing. The filters used should be capable of removing the unwanted frequencies, leaving the signal intact ( Fig. 10.6 ). Two types of filters are used for this purpose:
- a.
high-pass filters attenuate the frequency components of a signal below a certain frequency. They help to remove lower frequency noise from the signal. For example, the respiratory component from ECG can be removed by turning on a 1-Hz high-pass filter on the amplifier. The filter will centre the signal around the zero isoline
- b.
low-pass filters attenuate the frequency components of a signal above a certain frequency. They are useful for removing noise from lower frequency signals. So an amplifier with a 35-Hz low-pass filter will remove/attenuate signals above 35 Hz and help to ‘clean’ the ECG signal.
Fig. 10.6
ECG filters. (A) Unfiltered signal with noise. (B) Filtered ‘clean’ signal.
- a.
- 3.
The ECG monitor can have two modes:
- a.
the monitoring mode has a limited frequency response of 0.5–40 Hz. Filters are used to narrow the bandwidth to reduce environmental artefacts. The high-frequency filters reduce distortions from muscle movement, and mains current and electromagnetic interference from other equipment. The low-frequency filters help provide a stable baseline by reducing respiratory and body movement artefacts
- b.
the diagnostic mode has a wider frequency response of 0.05–150 Hz. The high-frequency limit allows the assessment of the ST segment, QRS morphology and tachyarrhythmias. The low-frequency limit allows representation of P- and T-wave morphology and ST-segment analysis.
- a.
- 4.
There are many ECG electrode configurations. Usually during anaesthesia, three skin electrodes are used (right arm, left arm and indifferent leads). The three limb leads used include two that are ‘active’ and one that is ‘inactive’ (earth). Sometimes five electrodes are used. Lead II is ideal for detecting arrhythmias. CM5 configuration is able to detect more than 80% of ST-segment changes due to left ventricular ischaemia. In CM5, the right arm electrode is positioned on the manubrium (chest lead from manubrium), the left arm electrode is on V5 position (fifth interspace in the left anterior axillary line) and the indifferent lead is on the left shoulder or any convenient position ( Fig. 10.7 ).
Fig. 10.7
The CM5 ECG lead configuration.
- 5.
The CB5 configuration is useful during thoracic anaesthesia. The right arm electrode is positioned over the centre of the right scapula and the left arm electrode is over V5.
- 6.
A display speed of 25 mm/s and a sensitivity of 1 mV/cm are standard in the UK.
Problems in practice and safety features
- 1.
Incorrect placement of the ECG electrodes in relation to the heart is a common error, leading to false information.
- 2.
Electrical interference can be a 50-Hz (in the UK) mains line interference because of capacitance or inductive coupling effect. Any electrical device powered by alternating current (AC) can act as one plate of a capacitor and the patient acts as the other plate. Interference can also be because of high-frequency current interference from diathermy. Most modern monitors have the facilities to avoid interference. Shielding of cables and leads, differential amplifiers and electronic filters all help to produce an interference-free monitoring system. Differential amplifiers measure the difference between the potential from two different sources. If interference is common to the two input terminals (e.g. mains frequency), it can be eliminated as only the differences between the two terminals is amplified. This is called common mode rejection ratio (CMRR). Amplifiers used in ECG monitoring should have a high CMRR of 100 000 : 1 to 1 000 000 : 1, which is a measurement of capability to reject the noise. They should also have a high input impedance (about 10 MΩ) to minimize the current taken from the electrodes. Table 10.1 shows the various types and sources of interference and how to reduce the interference.
Table 10.1
ECG signal interference
Type of interference
Sources of interference
How to reduce interference
Electromagnetic induction
Any electrical cable or light
Use long ECG and twisted leads (rejecting the induced signal as common mode)
Use selective filters in amplifiers
Electrostatic induction and capacitance coupling
Stray capacitances between table, lights, monitors, patients and electrical cables
ECG leads are surrounded by copper screens
Radiofrequency interference (> 150 Hz)
Diathermy enters the system by:
mains supply
direct application by probe
radio transmission via probe and wire
High-frequency filters clean up signal before entering input
Filtering power supply of amplifiers
Double screen electronic components of amplifiers and earth outer screen
Newer machines operate at higher frequencies
- 3.
Muscular activity, such as shivering, can produce artefacts. Positioning the electrodes over bony prominences and the use of low-pass filters can reduce these artefacts.
- 4.
High and low ventricular rate alarms and an audible indicator of ventricular rate are standard on most designs. More advanced monitors have the facility to monitor the ST segment ( Fig. 10.8 ). Continuous monitoring and measurement of the height of the ST segment allows early diagnosis of ischaemic changes.
Fig. 10.8
ECG and ST-segment monitoring.
(Courtesy Philips Healthcare, Guildford, UK.)
- 5.
Absence of or improperly positioned patient diathermy plate can cause burns at the site of ECG skin electrodes. This is because of the passage of the diathermy current via the electrodes causing a relatively high current density.
- •
Silver and silver chloride skin electrodes detect the electrical activity of the heart, 0.5–2 mV at the skin surface.
- •
The signal is boosted by an amplifier and displayed by an oscilloscope.
- •
The ECG monitor can have two modes, the monitoring mode (frequency range 0.5–40 Hz) and the diagnostic mode (frequency range 0.05–150 Hz).
- •
CM5 configuration is used to monitor left ventricular ischaemia.
- •
Electrical interference can be due either to diathermy or mains frequency.
- •
Differential amplifiers are used to reduce interference (common mode rejection).
Arterial blood pressure
Oscillometry is the commonest method used to measure arterial blood pressure non-invasively during anaesthesia. The systolic, diastolic and mean arterial pressures and pulse rate are measured, calculated and displayed. These devices give reliable trend information about the blood pressure. They are less reliable in circumstances where a sudden change in blood pressure is anticipated, or where a minimal change in blood pressure is clinically relevant. The term ‘device for indirect non-invasive automated mean arterial pressure’ (DINAMAP) is used for such devices.
Components
- 1.
A cuff with a tube is used for inflation and deflation. Some designs have an extra tube for transmitting pressure fluctuations to the pressure transducer.
- 2.
The case where the microprocessor, pressure transducer and a solenoid valve which controls the deflation of the arm cuff are housed. It contains the display and a timing mechanism which adjusts the frequency of measurements. Alarm limits can be set for both high and low values.
Mechanism of action
- 1.
The microprocessor is set to control the sequence of inflation and deflation.
- 2.
The cuff is inflated to a pressure above the previous systolic pressure, then it is deflated incrementally. The return of blood flow causes oscillation in cuff pressure ( Fig. 10.9 ).
Fig. 10.9
Diagram showing how oscillations in cuff pressure correspond to mean, systolic and diastolic pressures.
- 3.
The transducer senses the pressure changes which are interpreted by the microprocessor. This transducer has an accuracy of ± 2%.
- 4.
The output signal from the transducer passes through a filter to an amplifier that amplifies the oscillations. The output from the amplifier passes to the microprocessor through the analogue digital converter (ADC). The microprocessor controls the pneumatic pump for inflation of the cuff and the solenoid valve for deflation of the cuff.
- 5.
The systolic pressure corresponds to the onset of rapidly increasing oscillations. The mean arterial blood pressure corresponds to the maximum oscillation at the lowest cuff pressure.
- 6.
The diastolic pressure corresponds to the onset of rapidly decreasing oscillations. In addition, it is mathematically computed from the systolic and mean pressure values (mean blood pressure = diastolic blood pressure + 1/3 pulse pressure).
- 7.
The cuff must be of the correct size ( Table 10.2 ). It should cover at least two-thirds of the upper arm. The width of the cuff’s bladder should be 40% of the mid-circumference of the limb. The middle of the cuff’s bladder should be positioned over the brachial artery.
Table 10.2
A guide to the correct blood pressure cuff size
Width (cm)
Patient
3
Nneonate
5
Infant
6
Child
9
Small adult
12
Standard adult
15
Large adult
- 8.
Some designs have the ability to apply venous stasis to facilitate intravenous cannulation.
Problems in practice and safety features
- 1.
For the device to measure the arterial blood pressure accurately, it should have a fast cuff inflation and a slow cuff deflation (at a rate of 3 mmHg/s or 2 mmHg/beat). The former is to avoid venous congestion and the latter provides enough time to detect the arterial pulsation.
- 2.
If the cuff is too small, the blood pressure is over-read, whereas it is under-read if the cuff is too large. The error is greater with too small than too large a cuff.
- 3.
The systolic pressure is over-read at low pressures (systolic pressure less than 60 mmHg) and under-read at high systolic pressures.
- 4.
Atrial fibrillation and other arrhythmias affect performance.
- 5.
External pressure on the cuff or its tubing can cause inaccuracies.
- 6.
Frequently repeated cuff inflations can cause ulnar nerve palsy and petechial haemorrhage of the skin under the cuff.
The Finapres ( fin ger a rterial pres sure) device uses a combination of oscillometry and a servo control unit. The volume of blood in the finger varies with the cardiac cycle. A small cuff placed around the finger is used to keep the blood volume of the finger constant. An infrared photo-plethysmograph detects changes in the volume of blood within the finger with each cardiac cycle. A controller system alters the pressure in the cuff accordingly, to keep the volume of blood in the finger constant. The applied pressure waveform correlates with the arterial blood volume and, therefore, with the arterial blood pressure. This applied pressure is then displayed continuously, in real time, as the arterial blood pressure waveform.
The Von Recklinghausen oscillotonometer
During the premicroprocessor era, the Von Recklinghausen oscillotonometer was widely used ( Fig. 10.10 ).

Components
- 1.
It has two cuffs: the upper, occluding cuff (5-cm wide) overlaps a lower, sensing cuff (10-cm wide). An inflation bulb is attached.
- 2.
The case contains:
- a.
two bellows, one connected to the atmosphere, the other connected to the lower sensing cuff
- b.
a mechanical amplification system
- c.
the oscillating needle and dial
- d.
the control lever
- e.
the release valve.
- a.
Mechanism of action
- 1.
With the control lever at rest, air is pumped into both cuffs and the air-tight case of the instrument using the inflation bulb to a pressure exceeding systolic arterial pressure. By operating the control lever, the lower sensing cuff is isolated and the pressure in the upper cuff and instrument case is allowed to decrease slowly through an adjustable leak controlled by the release valve. As systolic pressure is reached, pulsation of the artery under the lower cuff results in pressure oscillations within the cuff and its bellows. The pressure oscillations are transmitted via a mechanical amplification system to the needle. As the pressure in the upper cuff decreases below diastolic pressure, the pulsation ceases.
- 2.
The mean pressure is at the point of maximum oscillation.
- 3.
This method is reliable at low pressures. It is useful to measure trends in blood pressure.
Problems in practice and safety features
- 1.
In order for the device to operate accurately, the cuffs must be correctly positioned and attached to their respective tubes.
- 2.
The diastolic pressure is not measured accurately with this device.
- •
Oscillometry is the method used.
- •
Mean arterial pressure corresponds to maximum oscillation.
- •
A cuff with a tube(s) is connected to a transducer and a microprocessor.
- •
Accurate within the normal range of blood pressure.
- •
Arrhythmias and external pressure affect the performance.
Pulse oximetry
This is a non-invasive measurement of the arterial blood oxygen saturation at the level of the arterioles. A continuous display of the oxygenation (plethysmographic oxygen saturation, SpO 2 ) is achieved by a simple, accurate and rapid method ( Fig. 10.11 ).

Pulse oximetry has proved to be a powerful monitoring tool in the operating theatre, recovery wards, intensive care units, general wards and during the transport of critically ill patients. It is considered to be the greatest recent technical advance in monitoring. It enables the detection of arterial hypoxaemia, allowing treatment before tissue damage occurs.
Components
- 1.
A probe is positioned on the finger, toe, ear lobe or nose ( Fig. 10.12 ). Two light-emitting diodes (LEDs) produce beams at red and infrared frequencies (660 nm and 940 nm, respectively) on one side and there is a sensitive photodetector on the other side. The LEDs operate in sequence at a rate of about 30 times/s ( Fig. 10.13 ).
Fig. 10.12
Pulse oximeter probes. Finger probe (top) and ear probe (bottom).
Fig. 10.13
Working principles of the pulse oximeter. The LEDs operate in sequence and when both are off the photodetector measures the background level of ambient light.
- 2.
The case houses the microprocessor. There is a display of the oxygen saturation, pulse rate and a plethysmographic waveform of the pulse. Alarm limits can be set for a low saturation value and for both high and low pulse rates.
Mechanism of action
- 1.
The oxygen saturation is estimated by measuring the transmission of light, through a pulsatile vascular tissue bed (e.g. finger). This is based on Beer’s law (the relation between the light absorbed and the concentration of solute in the solution) and Lamberťs law (relation between absorption of light and the thickness of the absorbing layer).
- 2.
The amount of light transmitted depends on many factors. The light absorbed by non-pulsatile tissues (e.g. skin, soft tissues, bone and venous blood) is constant (direct current [DC]). The non-constant absorption (AC) is the result of arterial blood pulsations ( Fig. 10.14 ). The sensitive photodetector generates a voltage proportional to the transmitted light. The AC component of the wave is about 1–5% of the total signal.
Fig. 10.14
Schematic representation of the contribution of various body components to the absorbance of light.
- 3.
The high frequency of the LEDs allows the absorption to be sampled many times during each pulse beat. This is used to enable running averages of saturation to be calculated many times per second. This decreases the ‘noise’ (e.g. movement) effect on the signal.
- 4.
The microprocessor is programmed to mathematically analyse both the DC and AC components at 660 and 940 nm calculating the ratio of absorption at these two frequencies (R : IR ratio). The result is related to the arterial saturation. The absorption of oxyhaemoglobin and deoxyhaemoglobin at these two wavelengths is very different. This allows these two wavelengths to provide good sensitivity. 805 nm is one of the isobestic points of oxyhaemoglobin and deoxyhaemoglobin. The OFF part allows a baseline measurement for any changes in ambient light.
- 5.
A more recent design uses multiple wavelengths to eradicate false readings from carboxyhaemoglobin and methaemoglobinaemia. Advanced oximeters use more than seven light wavelengths. This has enabled the measurement of haemoglobin value, oxygen content, carboxyhaemoglobin and methaemoglobin concentrations.
- 6.
A variable pitch beep provides an audible signal of changes in saturation.
Problems in practice and safety features
- 1.
It is accurate (± 2%) in the 70–100% range. Below the saturation of 70%, readings are extrapolated.
- 2.
The absolute measurement of oxygen saturation may vary from one probe to another but with accurate trends. This is due to the variability of the centre wavelength of the LEDs.
- 3.
Carbon monoxide poisoning (including smoking), coloured nail varnish, intravenous injections of certain dyes (e.g. methylene blue, indocyanine green) and drugs responsible for the production of methaemoglobinaemia are all sources of error ( Table 10.3 ).
Table 10.3
Sources of error in pulse oximetry
HbF
No significant clinical change (absorption spectrum is similar to the adult Hb over the range of wavelengths used)
MetHb
False low reading
CoHb
False high reading
SulphHb
Not a clinical problem
Bilirubin
Not a clinical problem
Dark skin
No effect
Methylene blue
False low reading
Indocyanine green
False low reading
Nail varnish
May cause false low reading
- 4.
Hypoperfusion and severe peripheral vasoconstriction affect the performance of the pulse oximeter. This is because the AC signal sensed is about 1–5% of the DC signal when the pulse volume is normal. This makes it less accurate during vasoconstriction when the AC component is reduced.
- 5.
The device monitors the oxygen saturation with no direct information regarding oxygen delivery to the tissues.
- 6.
Pulse oximeters average their readings every 10–20 seconds. They cannot detect acute desaturation. The response time to desaturation is longer with the finger probe (more than 60 seconds) whereas the ear probe has a response time of 10–15 seconds.
- 7.
Excessive movement or malposition of the probe is a source of error. Newer designs such as the Masimo oximeter claim more stability despite motion. External fluorescent light can be a source of interference.
- 8.
Inaccurate measurement can be caused by venous pulsation. This can be because of high airway pressures, the Valsalva manoeuvre or other consequences of impaired venous return. Pulse oximeters assume that any pulsatile absorption is caused by arterial blood pulsation only.
- 9.
The site of the application should be checked at regular intervals as the probe can cause pressure sores with continuous use. Some manufacturers recommend changing the site of application every 2 hours especially in patients with impaired microcirculation. Burns in infants have been reported.
- 10.
Pulse oximetry only gives information about a patienťs oxygenation. It does not give any indication of a patienťs ability to eliminate carbon dioxide.
- •
Consists of a probe with two LEDs and a photodetector.
- •
A microprocessor analyses the signal.
- •
Accurate within the clinical range.
- •
Inaccurate readings in carbon monoxide poisoning, the presence of dyes and methaemoglobinaemia.
- •
Hypoperfusion and severe vasoconstriction affect the reading.
Exam tip : it is important to have a good understanding of the physical principles used in pulse oximetry. Also know its limitations and the factors that can affect its accuracy.
End-tidal carbon dioxide analysers (capnographs)
Gases with molecules that contain at least two dissimilar atoms absorb radiation in the infrared region of the spectrum. Using this property, both inspired and exhaled carbon dioxide concentration can be measured directly and continuously throughout the respiratory cycle ( Fig. 10.15 ).
- 1.
Capnograph is the device that records and shows the graphical display of waveform of CO 2 (measured in kPa or mmHg). It displays the value of CO 2 at the end of expiration, which is known as end-tidal CO 2 .
- 2.
Capnogram is the graphical plot of CO 2 partial pressure (or percentage) versus time.
- 3.
Capnometer is the device which only shows numerical concentration of CO 2 without a waveform.

The end-tidal CO 2 is less than alveolar CO 2 because the end-tidal CO 2 is always diluted with alveolar dead space gas from unperfused alveoli. These alveoli do not take part in gas exchange and so contain no CO 2 . Alveolar CO 2 is less than arterial CO 2 as the blood from unventilated alveoli and lung parenchyma (both have higher CO 2 contents) mixes with the blood from ventilated alveoli. In healthy adults with normal lungs, end-tidal CO 2 is 0.3–0.6 kPa less than arterial CO 2 . This difference is reduced if the lungs are ventilated with large tidal volumes. The Greek root kapnos, meaning ‘smoke’, give us the term capnography (CO 2 can be thought as the ‘smoke’ of cellular metabolism).
End−tidalCO2<alveolarCO2<PaCO2
In reality, the devices used cannot determine the different phases of respiration but simply report the minimum and maximum CO 2 concentrations during each respiratory cycle.
Components
- 1.
The sampling chamber can either be positioned within the patienťs gas stream (main-stream version, Fig. 10.16 ) or connected to the distal end of the breathing system via a sampling tube (side-stream version, Fig. 10.17 ).
Fig. 10.16
A main-stream end-tidal carbon dioxide analyser.
Fig. 10.17
The Penlon PM9000 Express which measures end-tidal CO 2 , oximetry and inhalational agent concentration using a side-stream method.
(Courtesy Penlon Ltd, Abingdon, UK [ http://www.penlon.com ].)
- 2.
A photodetector measures light reaching it from a light source at the correct infrared wavelength (using optical filters) after passing through two chambers. One acts as a reference whereas the other one is the sampling chamber ( Fig. 10.18 ).
Fig. 10.18
Components of a gas analyser using an infrared light source suitable for end-tidal carbon dioxide measurement. The reference chamber has been omitted for the sake of clarity.
Mechanism of action
- 1.
Carbon dioxide absorbs the infrared radiation particularly at a wavelength of 4.3 μm.
- 2.
The amount of infrared radiation absorbed is proportional to the number of carbon dioxide molecules (partial pressure of carbon dioxide) present in the chamber.
- 3.
The remaining infrared radiation falls on the thermopile detector, which in turn produces heat. The heat is measured by a temperature sensor and is proportional to the partial pressure of carbon dioxide gas present in the mixture in the sample chamber. This produces an electrical output. This means that the amount of gas present is inversely proportional to the amount of infrared light present at the detector in the sample chamber ( Fig 10.19 ).
Fig. 10.19
Principles of infrared detector: due to the large amount of infrared absorption in the sample chamber by the carbon dioxide, little infrared finally reaches the detector.
- 4.
In the same way, a beam of light passes through the reference chamber which contains room air. The absorption detected from the sample chamber is compared to that in the reference chamber. This allows the calculation of carbon dioxide values.
- 5.
The inspired and exhaled carbon dioxide forms a square wave, with a zero baseline unless there is rebreathing ( Fig. 10.20A ).
Fig. 10.20
(A) An end-tidal carbon dioxide waveform which does not return to the baseline during inspiration indicating that rebreathing is occurring. (B) An end-tidal carbon dioxide waveform which illustrates the sloping plateau seen in patients with chronic obstructive airways disease. The normal waveform is superimposed (dotted line).
- 6.
A microprocessor-controlled infrared lamp is used. This produces a stable infrared source with a constant output. The current is measured with a current-sensing resistor, the voltage across which is proportional to the current flowing through it. The supply to the light source is controlled by the feedback from the sensing resistor maintaining a constant current of 150 mA.
- 7.
Using the rise and fall of the carbon dioxide during the respiratory cycle, monitors are designed to measure the respiratory rate.
- 8.
Alarm limits can be set for both high and low values.
- 9.
To avoid drift, the monitor should be calibrated regularly with known concentrations of CO 2 to ensure accurate measurement.
Photo-acoustic spectroscopy: in these infrared absorption devices, the sample gas is irradiated with pulsatile infrared radiation of a suitable wavelength. The periodic expansion and contraction produces a pressure fluctuation of audible frequency that can be detected by a microphone.
The advantages of photo-acoustic spectrometry over conventional infrared absorption spectrometry are as follows:
- 1.
The photo-acoustic technique is extremely stable and its calibration remains constant over much longer periods of time.
- 2.
The very fast rise and fall times give a much more accurate representation of any change in CO 2 concentration.
Carbon dioxide analysers can be either side-stream or main-stream analysers.
Side-stream analysers
- 1.
This consists of a 1.2-mm internal diameter tube that samples the gases (both inspired and exhaled) at a constant rate (e.g. 150–200 mL/min). The tube is connected to a lightweight adapter near the patienťs end of the breathing system (with a pneumotachograph for spirometry) with a small increase in the dead space. It delivers the gases to the sample chamber. The tube is made of Teflon so it is impermeable to carbon dioxide and does not react with anaesthetic agents.
- 2.
As the gases are humid, there is a moisture trap with an exhaust port, allowing gas to be vented to the atmosphere or returned to the breathing system.
- 3.
In order to accurately measure end-tidal carbon dioxide, the sampling tube should be positioned as close as possible to the patienťs trachea.
- 4.
A variable time delay before the sample is presented to the sample chamber is expected. The transit time delay depends on the length (which should be as short as possible, e.g. 2 m) and diameter of the sampling tube and the sampling rate. A delay of less than 3.8 seconds is acceptable. The rise time delay is the time for the analyser to respond to the signal and depends upon the size of the sample chamber and the gas flow.
- 5.
Other gases and vapours can be analysed from the same sample.
- 6.
Portable handheld side-stream analysers are available ( Fig. 10.21 ). They can be used during patient transport and out-of-hospital situations.
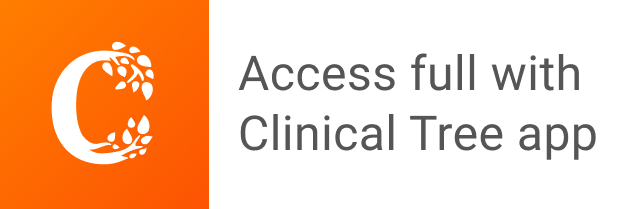